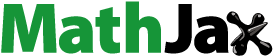
ABSTRACT
Membrane technology can play a suitable role in removing pharmaceutical active compounds since it requires low energy and simple operation. Even though membrane technology has progressed for wastewater applications nowadays, modifying membranes to achieve the strong desired membrane performance is still needed. Thus, this study overviews a comprehensive insight into the application of modified polymer membranes to remove pharmaceutical active compounds from wastewater. Biotoxicity of pharmaceutical active compounds is first prescribed to gain deep insight into how membranes can remove pharmaceutical active compounds from wastewater. Then, the behavior of the diffusion mechanism can be concisely determined using mass transfer factor model that represented by β and B with value up to 2.004 g h mg−1 and 1.833 mg g−1 for organic compounds including pharmaceutical active compounds. The model refers to the adsorption of solute to attach onto acceptor sites of the membrane surface, external mass transport of solute materials from the bulk liquid to the membrane surface, and internal mass transfer to diffuse a solute toward acceptor sites of the membrane surface with evidenced up to 0.999. Different pharmaceutical compounds have different solubility and relates to the membrane hydrophilicity properties and mechanisms. Ultimately, challenges and future recommendations have been presented to view the future need to enhance membrane performance regarding fouling mitigation and recovering compounds. Afterwards, the discussion of this study is projected to play a critical role in advance of better-quality membrane technologies for removing pharmaceutical active compounds from wastewater in an eco-friendly strategy and without damaging the ecosystem.
1.0 Introduction
The pharmaceutical industry has many effluents that contain antibiotic drugs, antipyretic drugs, analgesic drugs, and synthetic hormones contaminants [Citation1]. The growing pharmaceutical industry at 5.8% since 2017 is a line with the demand for enhancing the quality of human life [Citation2]. Instead of fulfilling human needs, effluent discharge causes environmental and health problems for non-targeting organisms [Citation3]. Several potential issues caused by pharmaceutical effluent contamination are resistance genes for antibiotics, carcinogens, cancer, mutagen, teratogen, higher anti-oxidant response, abnormalities, and mortalities of organisms and allergic reactions for humans [Citation4; Citation5]. Even antibiotic drugs, antipyretic drugs, analgesic drugs, and synthetic hormones such as amoxicillin, paracetamol, and several types of synthetic estrogens are easy to be degraded, their existence in the waters because of pharmaceutical wastewater has not been given much attention, and many countries still do not have standard medication disposal, such as Brazil [Citation6], East African [Citation7], and Malaysia [Citation8]. However, since the pharmaceutical effluent is quite different from the rest of the conventional effluents, there is a need to remove the pharmaceutical active compounds from wastewater before discharging [Citation9].
Polymer membrane technology is one of the alternative techniques to meet desirable discharge that has been instigated globally [Citation10]. Even though several challenges need to be solved for utilizing polymer membranes to remove the pharmaceutical active compounds from wastewater, polymer membrane is the potential to be developed further by their modification [Citation11]. In addition, several advantages can be obtained by employing a polymer membrane to eliminate pharmaceutical active compounds from wastewater and minimize the compounds contained in effluent. These advantages include its flexibility, chemical and mechanical properties, ease of fabrication, and, last but not least, its reasonable cost [Citation12]. Nowadays, non-modified polymer membrane used for removing pharmaceutical active compounds has several limitations, especially their removal efficiencies; modifying the polymer membrane is a promising better alternative to solve this issue. Moreover, modification of the membrane for removing pharmaceutical active compounds will be of great interest since it needs further exploration to achieve the finest modified polymer membrane [Citation13].
The use of modified polymer membranes in the pharmaceutical industry for eliminating pharmaceutical active compounds before discharging has a bright future. It has shown promising removal efficiencies and is easily implementable, cost-effective, and reusable, as shown in several recent studies. Polyvinylidene fluoride (PVDF) can be modified using several nanoparticles including silicon nanoparticles (SiO2), silver nanoparticles (Ag), titanium nanoparticles (TiO2), paladium nanoparticles (Pd), and zinc nanoparticles (ZnO). PVDF/SiO2 and PVDF/Ag-SiO2 membranes, had been fabricated for eliminating chemical oxygen demand from pharmaceutical wastewater with removal efficiencies 95.2% and 94.5%, which were higher compared to PVDF only (92.1%) [Citation14]. Polymer membrane (polysulfone) decorated 4% wt TiO2-HAP nanoparticles had been observed eliminating antibiotic drug chloramphenicol, up to 61.59% [Citation15]. A similar study obtained 51% of paracetamol removal percentage for polysulfone decorated 14% wt TiO2 for a membrane surface area of 80 cm2 and 80% for a membrane surface area of 320 cm2 [Citation16]. Another study employed Ag and Pd for the fabrication Ag-Pd/PVDF membrane for rejecting carbamazepine, caffeine, sulfamethoxazole, ibuprofen, and naproxen from pharmaceutical active compounds wastewater up to 40% [Citation17]. A study on eliminating ibuprofen also obtained 35.27% of percentage removal by utilizing PVDF-decorated Ag2CO3/Ag2O [Citation18]. Carbon nanotube utilized zeolitic imidazolate framework 8 (CNT@ZIF8) decorating PVDF-co-hexafluoropropylene (PVDF-co-HFP) could remove > 99.4% of antibiotics, for instance, tetracycline and fluoroquinolones [Citation19]. PVDF decorated Fe(III)-TiO2 has been proven to degrade 69.9% of bisphenol-A (Yang et al., 2021). Cerium fluoride (CeF3) nanoparticles embedding polysulfone achieved up to 97% of chemical oxygen demand removal from pharmaceutical active compounds wastewater [Citation20]. The presence of CeF3 on the membrane surface can enhance hydrophilicity and antifouling properties for eliminating contaminants in wastewater. However, these studies indicated that membrane technology can degrade and eliminate contaminants from pharmaceutical wastewater.
It is becoming increasingly clear that many studies are looking into using membrane technology in wastewater. Recently, it has been sought to organize and analyze information systematically through reviews. The following reviews have been produced on the performance of membranes for the treatment of wastewater: metal oxide functionalized ceramic membranes [Citation21], membranes for the removal of emerging contaminants [Citation22], membrane aerated biofilm reactor [Citation23], and carbon nanotubes for the sequestration of pollutants from wastewater [Citation24]. However, reviews need to comprehensively present the application of polymer membranes for treating wastewater, specifically removing pharmaceutical active compounds from wastewater, including membrane modification and the mass transfer mechanism of pharmaceutical active compounds through a modified polymer membrane. As in the case of most inventions and breakthroughs, these advances must be reviewed extensively in a comprehensive format as they will facilitate further refinement. Thus, this article fills a significant void in the literature by providing comprehensive deep insight into pharmaceutical active compounds biotoxicity and the application of modified polymer membranes to eliminate pharmaceutical active compounds from wastewater. It also overviews essential insights into future challenges and recommendations and proposes a mechanism for the mass transfer of pharmaceutical active compounds through modified polymer membranes. In following up on this study, the findings are expected to contribute to developing improved membrane technologies for removing pharmaceutical active compounds from wastewater in an environmentally friendly way without damaging the ecosystem as a consequence of the process.
2.0 Biotoxicity of pharmaceutical active compounds
2.1 Amoxicillin
Amoxicillin, as seen in , is usually used as an antibiotic for treating gastrointestinal health-associated diseases in humans and animals against various bacteria, including gram-negative and gram-positive bacteria. Amoxicillin is an effective treatment for some diseases, and consequently, its consumption and ingestion have increased in recent years. The ingestion of amoxicillin via excretion by up to 85% for human and 75% for the animal after utilized for maintaining human and animal health lead to several negative impacts on the environment, resulting in it being classified as an emerging contaminant [Citation25]. Considering amoxicillin is an emerging contaminant because of its soluble nature in water, it is easy to enter the soil, waterways, and aquatic environments easily through excretion. Even though excretion is the primary source of amoxicillin, several other major sources of amoxicillin, such as effluent discharged by pharmaceutical industrial facilities and hospitals, contribute to it. As a result of the continuous presence of amoxicillin in the environment that has been accumulated from these major sources, there is a tendency for antibiotic-resistance genes, carcinogens, mutagens, and teratogens to emerge, which pose a threat to organisms that are not targeted.
Figure 1. Chemical structure of pharmaceutical active compounds: (a) amoxicillin, (b) paracetamol, and (c) estrogen (17α-ethinylestradiol).

An earlier study has observed that amoxicillin can induce abnormal development in fetal mice and inhibit the function of several organs and systems, such as the reproductive organs, bones, the hippocampal system, and the liver [Citation26]. In a similar study, it was shown to induce hippocampal hypoplasia in the offspring of mice with impaired neuronal synaptic plasticity and a suppressed proliferation of hippocampal neuronal cells [Citation27]. In the aquatic environment, amoxicillin also induces mortality and oxidative stress in Daphnia magna [Citation28], as well as effects on sex ratio and egg-hatching time of the marine copepod Tigriopus fulvus [Citation29]. Aside from these effects, amoxicillin profoundly affects the enzymological, biochemical, and hematological responses in Labeo rohita [Citation30]. It indicated that amoxicillin exposure in fish induces hepatotoxicity, nephrotoxicity, leukocytosis, hematopoiesis, and lymphocytosis, inferring sustained toxic effects over time. As a result of the presence of amoxicillin in soil, the activity of the catalase enzyme in the soil is induced, and this may harm the growth and weight of plants, the root length, and shoot length, and the oxidative stress in the soil, such as Lemna minor [Citation31] and Spirodela polyrhiza [Citation32]. Amoxicillin also acutely inhibits Lactuca sativa seed germination [Citation33]. Relating to fish and plants, amoxicillin can also be acquired indirectly by non-target humans via the food chain, which can accumulate in the human body. There is evidence that antibiotics such as amoxicillin can negatively impact the health of young children, including weight gain, celiac disease, the presence of Escherichia coli, and genes related to antibiotic resistance [Citation34]. Further, it has adverse effects on the human body, resulting in immuno-allergic reactions and other undesirable effects [Citation35].
2.2 Paracetamol
As seen in , paracetamol is categorized as a non-steroidal anti-inflammatory drug generally employed as antipyretic and analgesic compounds to reduce fever and relieve pain such as backache, headaches, migraine, and cephalgia. Paracetamol is regarded as one of the most commonly used drugs in the pharmaceutical industry because it delivers the desired results, and its excretion rate is within 5% without any changes [Citation36]. Along with amoxicillin, it is also known that paracetamol can be found in hospital effluent, wastewater treatment plants, groundwater, surface water, and drinking water. Paracetamol concentrations were found to be as high as 0.042 mg L−1 in the surface water of the Beberibe River in Brazil [Citation37]. As in the Beberibe River, Brazil’s Semiarid Surface Water also contains 533.64 µg L−1 of paracetamol [Citation38]. On the north coast of Java Island, paracetamol levels have been determined to be 610 ng L−1 [Citation39]. A high concentration of paracetamol was found in the influent of the wastewater treatment plant, about 24,000 ng L−1, and in the Zarqa River, about 600 ng L−1 [Citation40]. There is also evidence of paracetamol in a drinking water treatment plant in Spain, with a concentration of 25.6 ng L−1 [Citation41]. Exposure to paracetamol in the environment threatens the organism and leads to several impacts.
Paracetamol exposure in the environment can affect embryonic development, locomotor behavior, biochemical processes, and epigenetic mechanisms in larvae and embryos of Danio rerio [Citation42]. Nostoc muscorum also displayed higher levels of antioxidant enzymes, including superoxide dismutase, catalase, ascorbate peroxidase, glutathione transferase, and glutathione reductase as well as osmolytes (proline) as a result of the increased paracetamol to counteract the oxidative stress [Citation43]. According to the previous study, exposure to paracetamol affected antioxidant response, which was shown to increase glutathione transferase activity, contrary to the increase found in Daphnia longispina, whereas the glutathione transferase activity decreased in Daphnia magna [Citation44]. It has also been reported that paracetamol has adverse effects on Mytilus edulis gonads, where hemocyte infiltration is found to be the most widespread pathology associated with paracetamol [Citation45]. Paracetamol exposure to Rhamdia quelen resulted in genotoxicity in the blood, gills, and posterior kidney at concentrations of 2.5 and 25 ng mL−1, which was confirmed by the presence of genotoxicity in blood and gills [Citation46].
Furthermore, paracetamol exposure led to lethality in Dugesia japonica [Citation47]. However, these studies indicated that paracetamol exposure could highly affect metabolic response in aquatic organisms, suggesting toxic effects in continuous time. Moreover, paracetamol exposure also led to several effects on soil organisms, such as increased hypoactivity (28 days) and burrowing time (96 h) in Hediste diversicolor [Citation48]. Paracetamol increases superoxide dismutase activity in intermediate exposure levels and glutathione peroxidase and glutathione reductase in chronic levels of exposure. In addition, paracetamol exposure to plants, such as Lens culinaris and Pisum sativum, influenced the percentage of chromosomal abnormalities, mitotic index, root growth, and the presence of micronucleus [Citation49]. Relating to the presence of paracetamol in water bodies, drinking water, and soil, paracetamol can be consumed by humans and organisms that are not targeted.
2.3 Estrogen
Estrogen is a female sex hormone mostly used for oral contraceptive consumption to control female fertility related to birth control [Citation50]. There are several types of estrogen, including estrone, 17β-estradiol, 17α-ethinylestradiol, estriol, and diethylstilbestrol. One of the estrogen molecule structures, 17α-ethinylestradiol, is presented in . It is also identified that 17α-ethinylestradiol can be found in wastewater treatment plants, groundwater, surface water, and soil. In wastewater treatment plants of 282 municipal wastewater treatment plants across 29 countries, 17α-ethinylestradiol in influent and effluent is up to 7890 and 549 ng L−1 [Citation51]. Still in wastewater treatment plants also, similarly, a previous study in Macao, China, presented that 17α-ethinylestradiol has been detected up to 0.96 ng g−1 in the wastewater treatment plant, 0.35 ng g−1 dw in the ecological zone, 0.48 ng g−1 dw in Taipa, and 0.38 ng g−1 dw in an industrial area [Citation52]. Other estrogen particulate concentrations, such as estrone, 17β-estradiol, and estriol, were inspected up to 14.4 ng g−1, 16.6 ng g−1, and 37.5 ng g−1 in the SPM of Yangzi River in China [Citation53]. In addition, colloidal bound phases also ranged from 14.6% to 35.5% for estrone, 7.10–39.5% for 17β-estradiol, and 10.9–29.8% for estriol. However, estrogen in the environment post ingestion can lead to undesired effects on organisms and human health, such as inhibiting metabolism and sexual fitness and inducing vitellogenin in male fish since non-target organisms potentially consume it.
Figure 2. Transformation of pharmaceutical active compounds: (a) amoxicillin, (b) paracetamol, and (c) estrogen (17α-ethinylestradiol).

17α-ethinylestradiol exposure in the aquatic environment cause the reduction of zebrafish larvae size by up to 25%, the swimming behavior of zebrafish larvae, changes the gene expression and proliferation cells, changes hair cell regeneration by up to 50%, and disrupts the annual nerve regeneration of the lateral line of zebrafish as well as the peripheral nervous system in zebrafish [Citation54]. 17α-ethinylestradiol exposure also affected Oncorhynchus mykiss gene expression and hepatic vitellogenin [Citation55]. In longer exposure, 17α-ethinylestradiol can decrease values of the swim tunnel respirometry. 17α-ethinylestradiol exposure can worsen the growth and reproduction of C. elegans [Citation56]. Estrogen, not only 17α-ethinylestradiol but also 17β-estradiol, exhibited a U-shape curve for A. clause embryos, a resistant gene that is not sensitive to their exposure, a reproductive ability and prosome length was noted in females raised with 100-gram L−1 of 17α-ethinylestradiol [Citation57]. In addition, higher stress, catalase activity, carboxylesterases activity, metabolic capacity, and cellular damage had been revealed for Mytilus galloprovincialis, which is exposed to the mixture of 17α-ethinylestradiol and salicylic acid, indicating that the potential severe effect will be found for the mixture of estrogens [Citation58]. These studies indicated that estrogen, especially 17α-ethinylestradiol and the mixture of estrogen with another pharmaceutical active compounds, severely impact aquatic organisms’ survival, development, sex ratio, and reproduction.
3.0 Modified polymer membrane for removing pharmaceutical active compounds
Polymer membranes have been widely contributed to removing contaminants from wastewater since there are many benefits to using this material [Citation59]. These benefits include its flexibility, chemical and mechanical properties, ease of fabrication, and, last but not least, its reasonable cost [Citation60]. Moreover, there are several types of polymer membranes, for instance, according to their sources, geometries, morphologies, and pore characteristics [Citation61]. Polymer membrane sources generally differentiate into biopolymer that extracted and processed from natural polymers, such as deoxyribonuclecic acid, ribonucleis acid, and gelatine [Citation62; Citation63], and synthetic polymer that derived from petroleum oil [Citation64]. According to membrane geometries, there are tubular, flat sheet, hollow fiber, and electrospun nanofiber [Citation65]. According to membrane morphologies, there are symmetric and asymmetric membranes [Citation66]. Symmetric membranes have one layer with uniform properties, while asymmetric membranes have two main layers with diverse properties, such as morphology and permeability. Asymmetric membranes can be differentiated into asymmetric integral membranes and composite membranes. Similar materials produce the top layer of asymmetric integral membranes, while composite membranes have different materials for their dense top layer. In addition, according to pore characteristics, there are porous and non-porous membranes.
Porous membranes are composed of pores ranging from 0.1 to 10 µm in diameter when used for microfiltration and 0.001 to 0.1 µm for ultrafiltration [Citation67]. It is widely appreciated that the mechanism of separation of porous membranes depends on the distribution of pore size and the molecular size of the membrane [Citation68]. The challenge for porous membranes is membrane fouling that causes flux decline over time. Furthermore, chemical and thermal stability plays a role in membrane selectivity and flux [Citation69]. In this case, it is possible to use the porous membrane support underneath, which can provide sufficient mechanical stability, strength, and high permeability by minimizing the barrier of resistance [Citation70]. Compared to porous membranes, non-porous membranes have pores less than 0.001 µm, making them ideal for nanofiltration, reverse osmosis, and molecular separation in gas phase applications. Non-porous membrane separation depends on pressure, concentration, and electrical potential gradient [Citation71].
Furthermore, there is a difference in solubility as well as diffusivity that leads to this phenomenon. Like porous membranes, non-porous membranes have the same challenge of low flux; they are usually made extremely thin and deposited on top of asymmetric membranes [Citation72]. However, above, composite membrane, categorized as a non-porous type, is potentially used for eliminating pharmaceuticals from wastewater that contains amoxicillin, paracetamol, and estrogen compounds.
In attempts to improve membrane performance and desirable characteristics for eliminating amoxicillin, paracetamol, and estrogen compounds from wastewater, several techniques are applied for modifying membranes, including bulk modification, blending modification, and surface modification [Citation73]. The previous studies employed Ag with SiO2 for grafting polymer membrane surfaces resulting in chemical oxygen demand removal of up to 98.1% from wastewater [Citation74]. Employing the immersion and precipitation method, methylcellulose as a pore-forming agent and activated carbon as an adsorbent were utilized for membrane modification, increasing water permeability by 34% for removing paracetamol. By adding 0.5% of methylcellulose and 2.5% of activated carbon, the porosity and the rejection rate achieved up to 85.38% and 41.57% for 10 ppm of paracetamol. By using the inversion technique, ZnO can be attached to the polyethersulfone resulting in C=C bond breakage and 90.24% of paracetamol degradation for 5 mL concentration and 86.18% for 10 mL concentration that the transformation of paracetamol is possible drawn as seen in [Citation75]. In the sole solution, the nanofiltration NF50 membrane removed 36% of paracetamol at pH 12 and 12.2% at pH 3 [Citation76]. It increased to 37.59% at pH 3 for a mixture of drug solutions containing paracetamol, ibuprofen, and diclofenac. These results indicated that paracetamol can be removed under acidic conditions and has higher removal for a mixture of drug solutions. Another modification membrane study employed 2% wt of SiO2 for surface coating polyimide membrane to remove 99.9% of paracetamol with 50 ppm of initial paracetamol concentration at natural pharmaceutical [Citation77].
Similarly, 2% wt of SiO2 was utilized for decorating polysulfone/polyvinylpyrrolidone membrane and reached 79.82% of amoxicillin removal percentage and getting higher up to 89.81% for 4% wt of SiO2 that its transformation is possible drawn as seen in [Citation78]. As well as SiO2, TiO2 can be utilized for modifying several membranes such as polysulfone, PVDF, and PTFE with removal efficiencies of estrogen (17α-ethinylestradiol) up to 96%, 94%, and 92% for 24 h under ultraviolet irradiation [Citation79]. A similarly modified membrane, PVDF-TiO2, can remove 96% of 17β-estradiol with 6.5% wt TiO2, so its transformation is possibly drawn as seen in [Citation80]. It indicated that modified polymer membranes have different abilities to eliminate estrogen compounds from wastewater. In addition, in the case of similarly modified membranes, they have a specific ability to remove different types of estrogen. Moreover, it is possible to obtain higher removal efficiencies for eliminating 17β-estradiol using a polysulfone membrane since the highest efficiency of 17α-ethinylestradiol removal was attained by polysulfone compared to PVDF.
Aside from the efficiency, it needs to assess pure water flux and pore size distribution, which are the key factors for porous membranes. Pure water flux of the membrane attained up to 105.1 L m−2 h−1 for PVDF/TiO2 at 0.45 wt% from 63.4 L m−2 h−1 for pristine PVDF [Citation81]. The presence of TiO2 in the PVDF membrane was well distributed at 0.45 wt% with a pore density of 21.17%. It was relatively higher compared to the non-presence of TiO2, with a pore density of 15.5%. Higher pure water flux tends to have higher pore density and relatively large pore size. It also increased the porosity by up to 70% and the roughness of the membrane’s upper surface. The contact angle of the PVDF/TiO2 membrane, prepared by the thermally induced phase separation method, ranged from 105° to 125° and was symmetric, indicating a hydrophobic membrane. Even though nanoparticles can increase the hydrophilicity, the combined materials with nanoparticles and preparation technique also affect hydrophilicity characteristics, such as a dimethyl phthalate as a combined material that is insoluble in water and thermally induced phase separation as a preparation technique. Another study evidenced that polyethyleneimine affected the contact angle characteristics [Citation82]. The presence of polyethyleneimine that was combined with TiO2 had a contact angle of less than 50°. It remained lower than the sole presence of polyethyleneimine (near 90°) and TiO2 (near 75°) in the PVDF membrane surface. Furthermore, the stability of the membrane is required to be characterized, such as thermal and mechanical stability. The combination of polyethyleneimine and TiO2 in the PVDF membrane surface had good mechanical stability since the permeate fluxes (~210 LMH) did not show significant changes in five cycles of utilization.
4.0 Mass transfer mechanism of pharmaceutical active compounds through modified polymer membrane
Modified membranes by coating and depositing particles on the polymer surface layer can improve membrane performance and provide valuable properties [Citation83]. Modified membrane using nanoparticles has been noted as a great strategy to eliminate the pharmaceutical active compounds from wastewater. Numerous nanoparticles have been proposed to be deposited on the membrane surface, such as Ag, Pd, TiO2, and SiO2, by coating or grafting technique [Citation84]. Nanoparticles can be applied to modify polymer membranes since they can enhance hydrophilicity, anti-fouling properties, self-cleaning properties, and increase removal percentage [Citation85]. In addition, nanoparticles on the membrane surface yielded contaminants’ resistance and antimicrobial properties [Citation86; Citation87]. These valuable properties are appropriate to be presented on modified polymer membranes for the degradation and removal process of pharmaceutical active compounds contained in pharmaceutical wastewater that are harmful to human health and the environment.
The ability of modified polymer membranes that employ nanoparticles to cover their surface can be reflected in . Modified polymer membrane for eliminating pharmaceutical active compounds from pharmaceutical wastewater has semi-permeable characteristics that allow excess water to leave the membrane while retaining pharmaceutical active compounds on its surface [Citation88]. This separation process correlates to physicochemical properties, including size, charge, solubility, and diffusivity of solutes as well as pore size, pore size distribution, geometry, wettability, hydrophilicity, membrane thickness, and transmembrane pressure [Citation89]. Fundamentally, the separation of the non-porous membrane plays a role in the solution diffusion [Citation90]. The molecular diffusion model can express the transport of particles or solutes through the membrane. Mass transfer through a membrane correlates with the barrier structure of the membrane, causing a solution-diffusion mechanism that refers to the concentration gradient of permeate and driving force. It has been noted that a penetrant molecule adsorbs, dissolves, and diffuses into the upstream side of a membrane (i.e. the high concentration side), moves over the membrane along a concentration gradient, and is ultimately free or desorbs from the downstream side of the membrane, which is the permeate side. The different solubility and diffusivity of the solutes on the membrane surface mainly led to this mechanism.
Figure 3. Mass transfer mechanism of water and pharmaceutical active compounds molecules through membrane surface.

As seen in , paracetamol, amoxicillin, and estrogen molecules are retained on the surface of the membrane as well as water molecules pass the membrane via diffusion as a mass transfer mechanism indicating the membrane is permeable for water, while nonpermeable for pharmaceutical active compounds molecules. As a consequence, a pharmaceutical active compound that is retained in the membrane surface is possible to form a cake layer. However, nanoparticles such as TiO2 and SiO2 have excellent self-cleaning properties, which can reduce or detach pharmaceutical active compounds particles from the membrane surface [Citation91]. As in the case of particle cakes, detached pharmaceutical active compounds particles on the membrane surface cannot further deepen the polymer membrane. These properties allow the modified polymer membrane to be an excellent semi-permeable membrane that can separate pharmaceutical active compounds from pharmaceutical wastewater. Given this, the membrane properties regarding water permeability, hydrophilicity, contaminant resistance ability, anti-fouling ability, and self-cleaning ability are well thought-out to synthesize and apply modified membranes with barrier structure to eliminate pharmaceutical active compounds from pharmaceutical wastewater.
According to a previous study, the complexity of particle transport can be defined using mass transfer models [Citation92]. There are three determinations regarding the mass transfer mechanism model: global mass transfer, external mass transfer, and internal mass transfer [Citation93]. Determining global mass transfer, external mass transfer, and internal mass transfer prescribes an external factor of mass transport and an internal one, including kinetic mass transport globally [Citation94]. Global mass transfer, external mass transfer, and internal mass transfer reflect three stages of the solute diffusion process onto a non-porous surface. Global mass transfer reflects the factor of total mass transport of material which determines the solute’s adsorption to attach onto the membrane surface’s acceptor sites [Citation95]. External mass transfer expresses the external transport of solute materials from the bulk liquid to the membrane surface. Internal mass transfer manifests a solute’s diffusion toward the membrane surface’s acceptor sites. The correlation of global mass transfer, external mass transfer, and internal mass transfer can be defined mathematically as:
where [kLa]d define as the internal mass transfer factor of pharmaceutical active compounds sorption, [kLa]g defines as the global mass transfer factor of pharmaceutical active compounds sorption, and [kLa]f defines as the external mass transfer factor of pharmaceutical active compounds sorption.
with
and
where B and β defines as the potential mass transfer constant correlated with the driving force as well as the intracellular accumulation of mass transfer during the time of adsorption (mg g−1) and the affinity of absorbate-adsorbent (g d mg−1).
Several studies presented that GMT can analyze the accumulation of adsorbate onto adsorbent surface as seen in . It had been evidenced that the mathematical model of GMT had good fit for understanding the adsorbate movement, such as PO43-, NH4+, Pb2+, Mn2+, Al3+, Cu2+, Fe2+, Cr(IV), and Hg0 ions as well as Remazol Brilliant Blue R and Methyl Orange dyes, onto the acceptor sites of the adsorbents which relates to R2 ranged from 0.970 to 0.999. Further, these adsorbates had β value for instance 1.123–1.336 g h mg−1 for PO43-and 1.146–1.686 g h mg−1 for Remazol Brilliant Blue R dye that followed by B value for instance ranged from −9.913 to −4.276 mg g−1 for PO43- and ranged from 0.220 to 1.651 mg g−1 for Remazol Brilliant Blue R dye. According to these studies, GMT is applicable for inorganic and organic compounds including pharmaceutical compounds such as amoxicillin, paracetamol, and estrogen. Therefore, it is possible to apply GMT to analyze the accumulation of pharmaceutical compounds onto membrane that play role as adsorbents.
Table 1. Overview potential mass transfer constant for removing adsorbate.
5.0 Challenge and future recommendation
The application of polymer membrane technology for pharmaceutical wastewater remains good performance. Nonetheless, earlier studies on polymer membranes suggested that the anti-fouling properties must be considered since particle pore blocking, pore constriction, and cake formation by particles easily block the membrane surface, especially pharmaceutical active compounds [Citation105]. Consequently, numerous studies assessed polymer membrane modification to diminish membrane fouling using grown materials on the membrane surface. Mostly, polymer membrane modification uses the chemical method since it is simple and provides desirable advantages on the membrane surface. By coating or grafting technique, several nanoparticles have been proposed to be deposited on the membrane surface, such as Ag, Pd, TiO2, and SiO2 [Citation106; Citation107]. Their presence on the membrane surface provides membrane fouling mitigation and offers other properties, such as antimicrobial properties [Citation86; Citation108] and self-cleaning properties [Citation109]. Besides deposited nanoparticles in the membrane surface, using ultraviolet pre-treatment for the membrane before applying it to the separation process is believed to enhance membrane performance regarding fouling mitigation. In addition, as well as membrane modification, pre-treatment techniques as fouling mitigation strategies, for instance, coagulation, electrocoagulation, adsorption, oxidation, filtration, and flocculation, are also effective approaches for lessening membrane fouling by removing foulants and their precursors. A previous study proved that coagulation could reduce fouling resistance, create larger flocs that are possibly eliminated before passing the membrane surface, and increase permeability [Citation110]. This technique can increase removal efficiencies in the range of 17–23% for handling several pharmaceutical active compounds, including mefenamic acid, atenolol, tetracycline, furosemide, atenolol, diclofenac, and ketoprofen. As well as coagulation, electrocoagulation applied in a membrane reactor can enhance nearly 20–30% removal efficiency of ofloxacin and paracetamol [Citation111]. Electrocoagulation can neutralize the solute charge and allow electrochemically adsorb or oxidate the foulants. In addition, the electrochemical technique provides reactive oxygen species that can clean the foulants in in-situ ways and exert repulsive force. Another study combined activated carbon as a pre-treatment or post-treatment technique and a nanofiltration membrane for removing diclofenac, caffeine, and octyl phenol, which achieved more than 95% of removal percentages [Citation112]. It has been noted that the application of activated carbon as a pre-treatment technique remained better than that of activated carbon as a post-treatment technique.
Similarly, a previous study presented that activated carbon as the pre-treatment technique can enhance the removal performance of the membrane by up to 80% compared to non-activated carbon as a pre-treatment technique for eliminating pharmaceutical active compounds that included paracetamol, triamterene, atrazine, nonylphenol, carbamazepine, simazine, Dilantin, and diuron [Citation113]. These pre-treatments can anticipate membrane fouling by eliminating larger particles before the separation process and causing lower flux than non-pre-treatments addition. However, as previously mentioned, the surface of polymer membranes, coated or grafted using nanoparticles such as TiO2 and SiO2, is believed to resist membrane fouling by particles and microbes.
As well as the pre-treatment or modification needs to avoid membrane fouling, the cleaning process of polymer membrane surface is still questionable. The cleaning process is required to provide sustainable membrane performance for the period that correlates with regeneration and recyclable properties. Several cleaning strategies have been proposed, including chemical and physical cleaning for removing pharmaceutical active compounds, as foulants, from pharmaceutical wastewater and recovering the foulants for further use [Citation114]. Chemical cleaning employs chemical compounds called alkaline agents, acids agents, and water. Alkaline solutions are particularly beneficial against organic foulants since the alkaline solution can break down the chemical bonds within the foulant, making it easier to remove and recovery the foulants.
It should be noted that strong alkaline compounds such as sodium hypochlorite (NaOCl) are also effective in removing and recovering biofouling from a vessel. In the event of frequent use, however, the membrane layer can also corrode and cause damage to the membrane as well [Citation115]. An acid compound such as vinegar is possible to remove the foulants. Nonetheless, it is still possible to see remnants on the membrane surface and a challenge to recovery the foulants, pharmaceutical active compounds, from membrane surface [Citation116]. Consequently, it also aggravated the scaling of the membrane due to the increased concentrations and temperature polarization effects. It is also possible to remove an adequate portion of the foulant layer with deionized water for water, which can achieve a high recovery of the permeate flux when used properly [Citation117]. The problem is that cleaning with deionized water can cause irreversible surface fouling. For physical cleaning strategies, there are air backwashing, aeration, and water flushing techniques that earlier studies have explored. Air backwashing for cleaning membrane surface requires homogeneous crystallization, meaning it arose in the bulk feed; the flux decrement was steady and started instantly. A previous study showed that air backwashing increased permeate productivity by up to 230% and crystal production by up to 32% [Citation118]. However, air backwashing seemed ineffective since it had no outcome in reducing flux decrement. Another technique is aeration, which can reduce flux by up to 95% [Citation119]. Nonetheless, it is noteworthy that aeration can remove inorganic material, which modifies the membrane surface, indicating a risk to the membrane’s performance. As well as a chemical technique using deionized water, the cleaning membrane using water flushing must frequently be done to prevent critical scaling.
6.0 Conclusion
Membrane technology is the most suitable pharmaceutical wastewater remediation technology due to its low energy requirement and simplicity of operation. Although membrane technology has advanced progress for wastewater application nowadays, there is still a required modification of membranes to attain high-performance membranes. As part of gaining an in-depth insight into membrane applications for removing pharmaceutical active compounds from pharmaceutical wastewater, this prospective review set out to explore the biotoxicity of pharmaceutical active compounds contaminants in pharmaceutical wastewater, the potential membrane modification for eliminating pharmaceutical active compounds contaminants in pharmaceutical wastewater, and the behavior pharmaceutical active compounds as well as diffusion mechanism of how pharmaceutical active compounds can be transferred onto the membrane surface. Furthermore, concisely, the diffusion mechanism is reflected using the mass transfer factor model comprising global mass transfer, external mass transfer, and internal mass transfer index, which refer to the adsorption of solute to attach onto acceptor sites of the membrane surface, external mass transport of solute materials from the bulk liquid to the membrane surface, and internal mass transfer to diffuse a solute toward acceptor sites of the membrane surface. Ultimately, challenges and future recommendations have been presented to understand the further need for enhancing membrane performance and applying modified membranes in the future.
CRediT authorship contribution statement
Anisa Ratnasari: Writing – original draft, review, editing & revising, Conceptualization
Acknowledgments
The author thanks Department of Environmental Engineering in Institut Teknologi Sepuluh Nopember for facilitating this work. A grateful thanks is also extended to the Indonesia Endowment Fund for Education, also known as LPDP (Lembaga Pengelolaan Dana Pendidikan), for supporting the present work. A special thanks is due to Mrs. Suparmi and Mr. Kusnul Yakin for their technical assistance.
Disclosure statement
No potential conflict of interest was reported by the author(s).
Data availability statement
No data was used for the research described in the article.
Additional information
Funding
References
- Hamad MTMH, El-Sesy ME. Adsorptive removal of levofloxacin and antibiotic resistance genes from hospital wastewater by nano-zero-valent iron and nano-copper using kinetic studies and response surface methodology. Bioresour Bioprocess. 2023;10(1):1. doi: 10.1186/s40643-022-00616-1
- González Peña OI, Zavala MÁ L, Cabral Ruelas H. Pharmaceuticals market, consumption trends and disease incidence are not driving the pharmaceutical research on water and wastewater. Int J Environ Res Public Health. 2021;18(5):2532. doi: 10.3390/ijerph18052532
- Hawash HB, Moneer AA, Galhoum AA, et al. Occurrence and spatial distribution of pharmaceuticals and personal care products (PPCPs) in the aquatic environment, their characteristics, and adopted legislations. J Water Process Eng. 2023;52:103490. doi: 10.1016/j.jwpe.2023.103490
- González-González RB, Sharma P, Singh SP, et al. Persistence, environmental hazards, and mitigation of pharmaceutical active residual contaminants from water matrices. Sci Total Environ. 2022;821:153329. doi: 10.1016/j.scitotenv.2022.153329
- Andrzejak T, Raje H, LaFleur G, et al. Water quality and antibiotic resistance in the recreational waters. Bioresour Technol. 2023;370:128546. doi: 10.1016/j.biortech.2022.128546
- Quadra GR, Silva PSA, Paranaíba JR, et al. Investigation of medicines consumption and disposal in Brazil: A study case in a developing country. Sci Total Environ. 2019;671:505–17. doi: 10.1016/j.scitotenv.2019.03.334
- Karungamye P, Rugaika A, Mtei K, et al. The pharmaceutical disposal practices and environmental contamination: A review in East African countries. HydroResearch. 2022;5:99–107. doi: 10.1016/j.hydres.2022.11.001
- Ariffin M, Zakili TST. Household pharmaceutical waste disposal in Selangor, Malaysia—policy, public perception, and current practices. Environ Manage. 2019;64(4):509–519. doi: 10.1007/s00267-019-01199-y
- Ratnasari A, Syafiuddin A, Zaidi NS, et al. Bioremediation of micropollutants using living and non-living algae - current perspectives and challenges. Environ Pollut. 2022;292:118474. doi: 10.1016/j.envpol.2021.118474
- Subrahmanya TM, Arshad AB, Lin PT, et al. A review of recent progress in polymeric electrospun nanofiber membranes in addressing safe water global issues. RSC Adv. 2021;11(16):9638–9663. doi: 10.1039/D1RA00060H
- Lu X, Elimelech M. Fabrication of desalination membranes by interfacial polymerization: history, current efforts, and future directions. Chem Soc Rev. 2021;50(11):6290–6307. doi: 10.1039/D0CS00502A
- Ravula T, Hardin NZ, Ramamoorthy A. Polymer nanodiscs: Advantages and limitations. Chem Phys Lipids. 2019;219:45–49. doi: 10.1016/j.chemphyslip.2019.01.010
- Song Y, Wang Y, Zhang N, et al. Quaternized carbon-based nanoparticles embedded positively charged composite membranes towards efficient removal of cationic small-sized contaminants. J Membr Sci. 2021;630:119332. doi: 10.1016/j.memsci.2021.119332
- Ahsani M, Hazrati H, Javadi M, et al. Preparation of antibiofouling nanocomposite PVDF/Ag-SiO2 membrane and long-term performance evaluation in the MBR system fed by real pharmaceutical wastewater. Sep Purif Technol. 2020;249:116938. doi: 10.1016/j.seppur.2020.116938
- Singh A, Ramachandran SK, Gumpu MB, et al. Titanium dioxide doped hydroxyapatite incorporated photocatalytic membranes for the degradation of chloramphenicol antibiotic in water. J Chem Technol Biotechnol. 2021;96(4):1057–1066. doi: 10.1002/jctb.6617
- Chijioke-Okere MO, Adlan Mohd Hir Z, Ogukwe CE, et al. TiO2/Polyethersulphone films for photocatalytic degradation of acetaminophen in aqueous solution. J Mol Liq. 2021;338:116692. doi: 10.1016/j.molliq.2021.116692
- Patala R, Mahlangu OT, Nyoni H, et al. In situ generation of fouling resistant Ag/Pd modified PES membranes for treatment of pharmaceutical wastewater. Membranes (Basel). 2022;12(8):762. doi: 10.3390/membranes12080762
- Rosman N, Norharyati Wan Salleh W, Aqilah Mohd Razali N, et al. Ibuprofen removal through photocatalytic filtration using antifouling PVDF- ZnO/Ag2CO3/Ag2O nanocomposite membrane. Mater Today Proc. 2021;42:69–74. doi: 10.1016/j.matpr.2020.09.476
- Yadav A, Yadav P, Labhasetwar PK, et al. CNT functionalized ZIF-8 impregnated poly(vinylidene fluoride-co-hexafluoropropylene) mixed matrix membranes for antibiotics removal from pharmaceutical industry wastewater by vacuum membrane distillation. J Environ Chem Eng. 2021;9(6):106560. doi: 10.1016/j.jece.2021.106560
- Zakeritabar SF, Jahanshahi M, Peyravi M, et al. Photocatalytic study of nanocomposite membrane modified by CeF3 catalyst for pharmaceutical wastewater treatment. J Environ Health Sci Eng. 2020;18(2):1151–1161. doi: 10.1007/s40201-020-00534-4
- Sawunyama L, Oyewo OA, Seheri N, et al. Metal oxide functionalized ceramic membranes for the removal of pharmaceuticals in wastewater. Surf Interfaces. 2023;38:102787. doi: 10.1016/j.surfin.2023.102787
- Kaczorowska MA, Bożejewicz D, Witt K. The application of polymer Inclusion membranes for the removal of emerging contaminants and synthetic dyes from aqueous solutions—A mini review. Membranes (Basel). 2023;13(2):132. doi: 10.3390/membranes13020132
- Li X, Bao D, Zhang Y, et al. Development and application of membrane aerated biofilm reactor (MABR)—A review. Water. 2023;15(3):436. doi: 10.3390/w15030436
- Majumder S, Dhara B, Mitra AK, et al. Applications and implications of carbon nanotubes for the sequestration of organic and inorganic pollutants from wastewater. Environ Sci Pollut Res. 2023. doi: 10.1007/s11356-023-25431-9
- Sodhi KK, Kumar M, Singh DK. Insight into the amoxicillin resistance, ecotoxicity, and remediation strategies. J Water Process Eng. 2021;39:101858. doi: 10.1016/j.jwpe.2020.101858
- Dai Y, Peng Y, Hu W, et al. Prenatal amoxicillin exposure induces developmental toxicity in fetal mice and its characteristics. J Environ Sci. 2024;137:287–301. doi: 10.1016/j.jes.2023.02.021
- Qin J, Yao B, Xie L, et al. Impact of prenatal amoxicillin exposure on hippocampal development deficiency. Neuropharmacology. 2023;223:109331. doi: 10.1016/j.neuropharm.2022.109331
- Yisa AG, Chia MA, Gadzama IMK, et al. Immobilization, oxidative stress and antioxidant response of Daphnia magna to amoxicillin and ciprofloxacin. Environ Toxicol Pharmacol. 2023;98:104078. doi: 10.1016/j.etap.2023.104078
- Prato E, Biandolino F, Grattagliano A, et al. Individual and combined effects of amoxicillin and carbamazepine to the marine copepod tigriopus fulvus. Environ Sci Pollut Res. 2023;30(22):61672–61681. doi: 10.1007/s11356-023-26498-0
- Umamaheswari S, Renuka SS, Ramesh M, et al. Chronic amoxicillin exposure affects Labeo rohita: assessment of hematological, ionic compounds, biochemical, and enzymological activities. Heliyon. 2019;5(4):e01434. doi: 10.1016/j.heliyon.2019.e01434
- Gomes MP, Moreira Brito JC, Cristina Rocha D, et al. Individual and combined effects of amoxicillin, enrofloxacin, and oxytetracycline on Lemna minor physiology. Ecotoxicol Environ Saf. 2020;203:111025. doi: 10.1016/j.ecoenv.2020.111025
- Singh V, Pandey B, Suthar S. Phytotoxicity of amoxicillin to the duckweed Spirodela polyrhiza: Growth, oxidative stress, biochemical traits and antibiotic degradation. Chemosphere. 2018;201:492–502. doi: 10.1016/j.chemosphere.2018.03.010
- Rede D, LHMLM S, Ramos S, et al. Individual and mixture toxicity evaluation of three pharmaceuticals to the germination and growth of Lactuca sativa seeds. Sci Total Environ. 2019;673:102–109. doi: 10.1016/j.scitotenv.2019.03.432
- Lebeaux RM, Madan JC, Nguyen QP, et al. Impact of antibiotics on off-target infant gut microbiota and resistance genes in cohort studies. Pediatr Res. 2022;92(6):1757–1766. doi: 10.1038/s41390-022-02104-w
- Garnier A-S, Lagarce L, Augusto J-F. Amoxicillin-induced crystal nephropathy: only a French touch? J Nephrol. 2022;35(3):1015–1016. doi: 10.1007/s40620-021-01240-6
- Benyekkou N, Ghezzar MR, Abdelmalek F, et al. Elimination of paracetamol from water by a spent coffee grounds biomaterial. Environ NanotechnolMonitmanag. 2020;14:100396. doi: 10.1016/j.enmm.2020.100396
- Veras TB, Luiz Ribeiro de Paiva A, Duarte MMMB, et al. Analysis of the presence of anti-inflammatories drugs in surface water: A case study in Beberibe river - PE, Brazil. Chemosphere. 2019;222:961–969. doi: 10.1016/j.chemosphere.2019.01.167
- Do Nascimento RF, de Carvalho Filho JAA, Napoleão DC, et al. Presence of non-Steroidal anti-Inflammatories in Brazilian Semiarid waters. Water Air Soil Pollut. 2023;234(4):225. doi: 10.1007/s11270-023-06239-2
- Koagouw W, Arifin Z, Olivier GWJ, et al. High concentrations of paracetamol in effluent dominated waters of Jakarta Bay, Indonesia. Mar Pollut Bull. 2021;169:112558. doi: 10.1016/j.marpolbul.2021.112558
- Shigei M, Assayed A, Hazaymeh A, et al. Pharmaceutical and antibiotic Pollutant levels in wastewater and the waters of the Zarqa River, Jordan. Appl Sci. 2021;11(18):8638. doi: 10.3390/app11188638
- Borrull J, Colom A, Fabregas J, et al. Presence, behaviour and removal of selected organic micropollutants through drinking water treatment. Chemosphere. 2021;276:130023. doi: 10.1016/j.chemosphere.2021.130023
- Nogueira AF, Pinto G, Correia B, et al. Embryonic development, locomotor behavior, biochemical, and epigenetic effects of the pharmaceutical drugs paracetamol and ciprofloxacin in larvae and embryos of danio rerio when exposed to environmental realistic levels of both drugs. Environ Toxicol. 2019;34(11):1177–1190. doi: 10.1002/tox.22819
- Fatima S, Asif N, Ahmad R, et al. Toxicity of NSAID drug (paracetamol) to nontarget organism—nostoc muscorum. Environ Sci Pollut Res. 2020;27(28):35208–35216. doi: 10.1007/s11356-020-09802-0
- Sousa AP, Nunes B. Dangerous connections: biochemical and behavioral traits in Daphnia magna and Daphnia longispina exposed to ecologically relevant amounts of paracetamol. Environ Sci Pollut Res. 2021;28(29):38792–38808. doi: 10.1007/s11356-021-13200-5
- Koagouw W, Ciocan C. Effects of short-term exposure of paracetamol in the gonads of blue mussels Mytilus edulis. Environ Sci Pollut Res. 2020;27(25):30933–30944. doi: 10.1007/s11356-019-06861-w
- Perussolo MC, Guiloski IC, Lirola JR, et al. Integrated biomarker response index to assess toxic effects of environmentally relevant concentrations of paracetamol in a neotropical catfish (Rhamdia quelen). Ecotoxicol Environ Saf. 2019;182:109438. doi: 10.1016/j.ecoenv.2019.109438
- Zhang S, Hagstrom D, Hayes P, et al. Multi-behavioral endpoint testing of an 87-chemical compound library in freshwater planarians. Toxicol Sci. 2018;167(1):26–44. doi: 10.1093/toxsci/kfy145
- Nogueira AF, Nunes B. Effects of paracetamol on the polychaete hediste diversicolor: occurrence of oxidative stress, cyclooxygenase inhibition and behavioural alterations. Environ Sci Pollut Res. 2021;28(21):26772–26783. doi: 10.1007/s11356-020-12046-7
- Mercado SAS, Galvis DGV. Paracetamol ecotoxicological bioassay using the bioindicators lens culinaris med. And pisum sativum L. Environ Sci Pollut Res. 2023;30(22):61965–61976. doi: 10.1007/s11356-023-26475-7
- Ratnasari A, Syafiuddin A, Kueh ABH, et al. Opportunities and challenges for sustainable bioremediation of natural and synthetic estrogens as emerging water contaminants using bacteria, fungi, and algae. Water Air Soil Pollut. 2021;232(6):242. doi: 10.1007/s11270-021-05183-3
- Tang Z, Z-H L, Wang H, et al. Occurrence and removal of 17α-ethynylestradiol (EE2) in municipal wastewater treatment plants: Current status and challenges. Chemosphere. 2021;271:129551. doi: 10.1016/j.chemosphere.2021.129551
- Moreira IS, Lebel A, Peng X, et al. Sediments in the mangrove areas contribute to the removal of endocrine disrupting chemicals in coastal sediments of Macau SAR, China, and harbour microbial communities capable of degrading E2, EE2, BPA and BPS. Biodegradation. 2021;32(5):511–529. doi: 10.1007/s10532-021-09948-9
- Huang Y, Xie X, Zhou LJ, et al. Multi-phase distribution and risk assessment of endocrine disrupting chemicals in the surface water of the Shaying River, -Huai River Basin, China. Ecotoxicol Environ Saf. 2019;173:45–53. doi: 10.1016/j.ecoenv.2019.02.016
- Nasri A, Mezni A, Lafon P-A, et al. Ethinylestradiol (EE2) residues from birth control pills impair nervous system development and swimming behavior of zebrafish larvae. Sci Total Environ. 2021;770:145272. doi: 10.1016/j.scitotenv.2021.145272
- Rehberger K, Wernicke von Siebenthal E, Bailey C, et al. Long-term exposure to low 17α-ethinylestradiol (EE2) concentrations disrupts both the reproductive and the immune system of juvenile rainbow trout, oncorhynchus mykiss. Environ Int. 2020;142:105836. doi: 10.1016/j.envint.2020.105836
- Kuo Y-H, How CM, Huang C-W, et al. Co-contaminants of ethinylestradiol and sulfamethoxazole in groundwater exacerbate ecotoxicity and ecological risk and compromise the energy budget of C. elegans. Aquat Toxicol. 2023;257:106473. doi: 10.1016/j.aquatox.2023.106473
- Djebbi E, Yahia MND, Farcy E, et al. Acute and chronic toxicity assessments of 17β-estradiol (E2) and 17α-ethinylestradiol (EE2) on the calanoid copepod acartia clausi: Effects on survival, development, sex-ratio and reproduction. Sci Total Environ. 2022;807:150845. doi: 10.1016/j.scitotenv.2021.150845
- Cunha M, Silva MG, De Marchi L, et al. Toxic effects of a mixture of pharmaceuticals in Mytilus galloprovincialis: The case of 17α-ethinylestradiol and salicylic acid. Environ Pollut. 2023;324:121070. doi: 10.1016/j.envpol.2023.121070
- Castro-Muñoz R, González-Melgoza LL, García-Depraect O. Ongoing progress on novel nanocomposite membranes for the separation of heavy metals from contaminated water. Chemosphere. 2021;270:129421. doi: 10.1016/j.chemosphere.2020.129421
- Akter M, Uddin MH, Anik HR. Plant fiber-reinforced polymer composites: a review on modification, fabrication, properties, and applications. Polym Bull. 2023. doi: 10.1007/s00289-023-04733-5
- Kloos J, Joosten N, Schenning A, et al. Self-assembling liquid crystals as building blocks to design nanoporous membranes suitable for molecular separations. J Membr Sci. 2021;620:118849. doi: 10.1016/j.memsci.2020.118849
- El-Mossalamy EH, Batouti MEL, Fetouh HA. The role of natural biological macromolecules: Deoxyribonucleic and ribonucleic acids in the formulation of new stable charge transfer complexes of thiophene schiff bases for various life applications. Int j biol macromol. 2021;193:1572–1586. doi: 10.1016/j.ijbiomac.2021.10.220
- Elbatouti M, Fetouh HA. Extraction of eco-friendly and biodegradable surfactant for inhibition of copper corrosion during acid pickling. Adsorpt Sci Technol. 2019;37(7–8):649–663. doi: 10.1177/0263617419865130
- Kim S, Wang H, Lee YM. 2D nanosheets and their composite membranes for water, gas, and Ion separation. Angew. Chem. Int. Ed. 2019;58(49):17512–17527. doi: 10.1002/anie.201814349
- Huang Y, Xiao C, Huang Q, et al. Progress on polymeric hollow fiber membrane preparation technique from the perspective of green and sustainable development. Chem Eng J. 2021;403:126295. doi: 10.1016/j.cej.2020.126295
- Gouveia ASL, Malcaitè E, Lozinskaya EI, et al. Poly(ionic liquid)–ionic liquid membranes with fluorosulfonyl-derived anions: Characterization and biohydrogen separation. ACS Sustain Chem Eng. 2020;8(18):7087–7096. doi: 10.1021/acssuschemeng.0c00960
- Hu Y, Wu W. Application of membrane filtration to cold sterilization of drinks and establishment of aseptic workshop. Food Environ Virol. 2023;15(2):89–106. doi: 10.1007/s12560-023-09551-6
- Wang Z, Chen Z, Zheng Z, et al. Nanocellulose-based membranes for highly efficient molecular separation. Chem Eng J. 2023;451:138711. doi: 10.1016/j.cej.2022.138711
- Karami P, Khorshidi B, McGregor M, et al. Thermally stable thin film composite polymeric membranes for water treatment: A review. J Clean Prod. 2020;250:119447. doi: 10.1016/j.jclepro.2019.119447
- DuChanois RM, Porter CJ, Violet C, et al. Membrane materials for selective ion separations at the water–energy nexus. Adv Mater. 2021;33(38):2101312. doi: 10.1002/adma.202101312
- Apel P, Koter S, Yaroshchuk A. Time-resolved pressure-induced electric potential in nanoporous membranes: Measurement and mechanistic interpretation. J Membr Sci. 2022;653:120556. doi: 10.1016/j.memsci.2022.120556
- Li B, Qi B, Guo Z, et al. Recent developments in the application of membrane separation technology and its challenges in oil-water separation: A review. Chemosphere. 2023;327:138528. doi: 10.1016/j.chemosphere.2023.138528
- Mamah SC, Goh PS, Ismail AF, et al. Recent development in modification of polysulfone membrane for water treatment application. J Water Process Eng. 2021;40:101835. doi: 10.1016/j.jwpe.2020.101835
- Behboudi A, Jafarzadeh Y, Yegani R. Incorporation of silica grafted silver nanoparticles into polyvinyl chloride/polycarbonate hollow fiber membranes for pharmaceutical wastewater treatment. Chem Eng Res Des. 2018;135:153–165. doi: 10.1016/j.cherd.2018.03.019
- Chijioke-Okere M, Abdullah AH, Mohd Hir ZA, et al. Efficient photodegradation of paracetamol by integrated PES-ZnO photocatalyst sheets. Inorg Chem Commun. 2023;148:110377. doi: 10.1016/j.inoche.2022.110377
- Maryam B, Buscio V, Odabasi SU, et al. A study on behavior, interaction and rejection of paracetamol, diclofenac and ibuprofen (PhAcs) from wastewater by nanofiltration membranes. Environ Technol Innov. 2020;18:100641. doi: 10.1016/j.eti.2020.100641
- Kuttiani Ali J, Abi Jaoude M, Alhseinat E. Polyimide ultrafiltration membrane embedded with reline-functionalized nanosilica for the remediation of pharmaceuticals in water. Sep Purif Technol. 2021;266:118585. doi: 10.1016/j.seppur.2021.118585
- Shakak M, Rezaee R, Maleki A, et al. Synthesis and characterization of nanocomposite ultrafiltration membrane (PSF/PVP/SiO2) and performance evaluation for the removal of amoxicillin from aqueous solutions. Environ Technol Innov. 2020;17:100529. doi: 10.1016/j.eti.2019.100529
- Dekkouche S, Morales-Torres S, Ribeiro AR, et al. In situ growth and crystallization of TiO2 on polymeric membranes for the photocatalytic degradation of diclofenac and 17α-ethinylestradiol. Chem Eng J. 2022;427:131476. doi: 10.1016/j.cej.2021.131476
- Liu S, Véron E, Lotfi S, et al. Poly(vinylidene fluoride) membrane with immobilized TiO2 for degradation of steroid hormone micropollutants in a photocatalytic membrane reactor. J Hazard Mater. 2023;447:130832. doi: 10.1016/j.jhazmat.2023.130832
- Shi F, Ma Y, Ma J, et al. Preparation and characterization of PVDF/TiO2 hybrid membranes with different dosage of nano-TiO2. J Membr Sci. 2012;389:522–531. doi: 10.1016/j.memsci.2011.11.022
- Deng W, Fan T, Li Y. In situ biomineralization-constructed superhydrophilic and underwater superoleophobic PVDF-TiO2 membranes for superior antifouling separation of oil-in-water emulsions. J Membr Sci. 2021;622:119030. doi: 10.1016/j.memsci.2020.119030
- Zou D, Lee YM. Design strategy of poly(vinylidene fluoride) membranes for water treatment. Prog Polym Sci. 2022;128:101535. doi: 10.1016/j.progpolymsci.2022.101535
- Qing W, Liu F, Yao H, et al. Functional catalytic membrane development: A review of catalyst coating techniques. Adv Colloid Interface Sci. 2020;282:102207. doi: 10.1016/j.cis.2020.102207
- Liu T, Liang R, Qin W. Anti-fouling TiO2-Coated polymeric membrane ion-selective electrodes with photocatalytic self-cleaning properties. Anal Chem. 2023;95(16):6577–6585. doi: 10.1021/acs.analchem.2c05514
- Ratnasari A, Endarko E, Syafiuddin A. A green method for the enhancement of antifungal properties of various textiles functionalized with silver nanoparticles. Biointerface Res Appl Chem. 2020;10. doi: 10.33263/BRIAC106.72847294
- Fadlilah D, Endarko E, Ratnasari A, et al. Enhancement of antibacterial properties of various polymers functionalized with silver nanoparticles. Biointerface Res Appl Chem. 2020;10:5592–5598. doi: 10.33263/BRIAC0103.592598
- Yang H, Liu H-B, Tang Z-S, et al. Synthesis, performance, and application of molecularly imprinted membranes: A review. J Environ Chem Eng. 2021;9(6):106352. doi: 10.1016/j.jece.2021.106352
- Radjabian M, Abetz V. Advanced porous polymer membranes from self-assembling block copolymers. Prog Polym Sci. 2020;102:101219. doi: 10.1016/j.progpolymsci.2020.101219
- Warsinger DM, Chakraborty S, Tow EW, et al. A review of polymeric membranes and processes for potable water reuse. Prog Polym Sci. 2018;81:209–237. doi: 10.1016/j.progpolymsci.2018.01.004
- Dolatshah M, Zinatizadeh AA, Zinadini S, et al. Preparation, characterization and performance assessment of antifouling L-Lysine (C, N codoped)-TiO2/WO3-PES photocatalytic membranes: A comparative study on the effect of blended and UV-grafted nanophotocatalyst. J Environ Chem Eng. 2022;10(6):108658. doi: 10.1016/j.jece.2022.108658
- Ratnasari A, Syafiuddin A, Budiarti RPN, et al. Mass transfer mechanisms of water pollutions adsorption mediated by different natural adsorbents. Environ Qual Manag. 2022;32(1):95–104. doi: 10.1002/tqem.21849
- Fulazzaky MA, Salim NAA, Puteh MH, et al. Reliability of the mass transfer factor models to describe the adsorption of NH4+ by granular activated carbon. Int J Environ Res. 2022;16(3):30. doi: 10.1007/s41742-022-00408-7
- Pantić K, Bajić ZJ, Veličković ZS, et al. Arsenic removal by copper-impregnated natural mineral tufa part II: a kinetics and column adsorption study. Environ Sci Pollut Res. 2019;26(23):24143–24161. doi: 10.1007/s11356-019-05547-7
- Syafiuddin A, Fulazzaky MA. Decolorization kinetics and mass transfer mechanisms of Remazol Brilliant Blue R dye mediated by different fungi. Biotechnol Rep. 2021;29:e00573. doi: 10.1016/j.btre.2020.e00573
- Fulazzaky MA, Salim NAA, Khamidun MH, et al. The mechanisms and kinetics of phosphate adsorption onto iron-coated waste mussel shell observed from hydrodynamic column. Int J Environ Sci Technol. 2022;19(7):6345–6358. doi: 10.1007/s13762-021-03563-0
- Salim NAA, Fulazzaky MA, Puteh MH, et al. Mass transfer kinetics and mechanisms of phosphate adsorbed on waste mussel shell. Water Air Soil Pollut. 2022;233(6):223. doi: 10.1007/s11270-022-05693-8
- Khamidun MH, Rahman MAA, Zainorizuan MJ, et al. Analysis of mass transfer resistance for adsorption of phosphate onto industrial waste materials in plug-flow column. International Symposium on Civil and Environmental Engineering; 5-6 December 2016; Malaysia. MATEC Web conf. 2017;103:06004. doi: 10.1051/matecconf/201710306004
- Khamidun M, Ali Fulazzaky M, Al-Gheethi A, et al. Adsorption of ammonium from wastewater treatment plant effluents onto the zeolite; a plug-flow column, optimisation, dynamic and isotherms studies. Int J Environ Anal Chem. 2022;102(19):8445–8466. doi: 10.1080/03067319.2020.1849659
- Mohamed LA, Aniagor CO, Taha GM, et al. Mechanistic investigation of the mass transfer stages involved during the adsorption of aqueous lead onto scopulariopsis brevicompactum fungal biomass. Environ Challenges. 2021;5:100373. doi: 10.1016/j.envc.2021.100373
- Tran TN, Kim D-G, Ko S-O. Adsorption mechanisms of manganese (II) ions onto acid-treated activated carbon. KSCE J Civil Eng. 2018;22(10):3772–3782. doi: 10.1007/s12205-018-1334-6
- Ezechi EH, Isa MH, Muda K, et al. A comparative evaluation of two electrode systems on continuous electrocoagulation of boron from produced water and mass transfer resistance. J Water Process Eng. 2020;34:101133. doi: 10.1016/j.jwpe.2020.101133
- Salim NAA, Puteh MH, Othman MHD, et al. The adsorption of Cr(VI) from synthetic solution onto mussel shell incorporated polyethersulfone flat sheet membranes: Application of the mass transfer factor models. J Water Chem Technol. 2023;45(3):222–233. doi: 10.3103/S1063455X23030098
- Zhou Q, Zhu F, Gong R, et al. Adsorption, regeneration and kinetic of gas phase elemental mercury capture on sulfur incorporated porous carbon synthesized by template method under simulated coal-fired flue gas. Fuel. 2023;342:127925. doi: 10.1016/j.fuel.2023.127925
- Siagian UWR, Khoiruddin K, Ting YP, et al. Advances in membrane bioreactor: High performance and antifouling configurations. Curr Pollut Rep. 2022;8(2):98–112. doi: 10.1007/s40726-022-00217-8
- Heng ZW, Chong WC, Pang YL, et al. Novel visible-light responsive NCQDs-TiO2/PAA/PES photocatalytic membrane with enhanced antifouling properties and self-cleaning performance. J Environ Chem Eng. 2021;9(4):105388. doi: 10.1016/j.jece.2021.105388
- Teng L, Yue C, Zhang G. Epoxied SiO2 nanoparticles and polyethyleneimine (PEI) coated polyvinylidene fluoride (PVDF) membrane for improved oil water separation, anti-fouling, dye and heavy metal ions removal capabilities. J Colloid Interface Sci. 2023;630:416–429. doi: 10.1016/j.jcis.2022.09.148
- Ratnasari A. Antimicrobial textile modified with silver nanoparticles in-situ synthesized using weed leaves extract. Environ Toxicol Manage. 2021;1(3):15–18. doi: 10.33086/etm.v1i3.2502
- Chi L, Qian Y, Guo J, et al. Novel g-C3N4/TiO2/PAA/PTFE ultrafiltration membrane enabling enhanced antifouling and exceptional visible-light photocatalytic self-cleaning. Catal Today. 2019;335:527–537. doi: 10.1016/j.cattod.2019.02.027
- Park J, Yamashita N, Tanaka H. Membrane fouling control and enhanced removal of pharmaceuticals and personal care products by coagulation-MBR. Chemosphere. 2018;197:467–476. doi: 10.1016/j.chemosphere.2018.01.063
- Mendes Predolin L, Moya-Llamas MJ, Vásquez-Rodríguez ED, et al. Effect of current density on the efficiency of a membrane electro-bioreactor for removal of micropollutants and phosphorus, and reduction of fouling: A pilot plant case study. J Environ Chem Eng. 2021;9(1):104874. doi: 10.1016/j.jece.2020.104874
- Huang Z, Gong B, Huang C-P, et al. Performance evaluation of integrated adsorption-nanofiltration system for emerging compounds removal: Exemplified by caffeine, diclofenac and octylphenol. J Environ Manage. 2019;231:121–128. doi: 10.1016/j.jenvman.2018.09.092
- Duc Viet N, Lee H, Im S-J, et al. Fate, elimination, and simulation of low-molecular-weight micropollutants in an integrated activated carbon-fertiliser drawn osmotic membrane bioreactor. Bioresour Technol. 2022;351:126972. doi: 10.1016/j.biortech.2022.126972
- Batouti ME, Sadik W, Eldemerdash AG, et al. New and innovative microwave-assisted technology for synthesis of guar gum-grafted acrylamide hydrogel superabsorbent for the removal of acid red 8 dye from industrial wastewater. Polym Bull. 2023;80(5):4965–4989. doi: 10.1007/s00289-022-04254-7
- Zhang H, Sun M, Song L, et al. Fate of NaClO and membrane foulants during in-situ cleaning of membrane bioreactors: Combined effect on thermodynamic properties of sludge. Biochem Eng J. 2019;147:146–152. doi: 10.1016/j.bej.2019.04.016
- Heylen C, Oliveira Aguiar A, String G, et al. Laboratory efficacy of locally available backwashing methods at removing fouling in hollow-fiber membrane filters used for household water treatment. Membranes (Basel). 2021;11(5):375. doi: 10.3390/membranes11050375
- Ping M, Zhang X, Liu M, et al. Surface modification of polyvinylidene fluoride membrane by atom-transfer radical-polymerization of quaternary ammonium compound for mitigating biofouling. J Membr Sci. 2019;570-571:286–293. doi: 10.1016/j.memsci.2018.10.070
- Julian H, Ye Y, Li H, et al. Scaling mitigation in submerged vacuum membrane distillation and crystallization (VMDC) with periodic air-backwash. J Membr Sci. 2018;547:19–33. doi: 10.1016/j.memsci.2017.10.035
- Zhang W, Yu S, Zhao H, et al. Vacuum membrane distillation for seawater concentrate treatment coupled with microbubble aeration cleaning to alleviate membrane fouling. Sep Purif Technol. 2022;290:120864. doi: 10.1016/j.seppur.2022.120864