ABSTRACT
Intestinal ischemia-reperfusion (I/R) injury is a condition in which tissue injury is aggravated after ischemia due to recovery of blood supply. Bone marrow mesenchymal stem cell-derived exosome (BMSC-exo) showed a protective effect on I/R injury. This study aimed to investigate the possible mechanisms by which BMSC-exos ameliorate intestinal I/R injury. We isolated mouse BMSC-exos by super-centrifugation and found that they effectively increased cell viability in a cell model, alleviated intestinal barrier injury in a mouse model, and downregulated the expression of inflammatory cytokines and pyroptosis-related proteins, suggesting that BMSC-exos may alleviate intestinal I/R injury in vitro and in vivo by regulating pyroptosis. We identified miR-143-3p as a differentially expressed miRNA by microarray sequencing. Bioinformatic analysis predicted a binding site between miR-143-3p and myeloid differentiation factor 88 (MyD88); a dual-luciferase reporter assay confirmed that miR-143-3p could directly regulate the expression of MyD88. Our findings suggest that miR-143-3p regulates pyroptosis by regulating NOD-like receptor thermal protein domain associated protein 3 (NLRP3) through the toll-like receptor (TLR)-4/MyD88/nuclear factor kappa-B (NF-кB) pathway. This study describes a potential strategy for the treatment of intestinal I/R injury using BMSC-exos that act by regulating pyroptosis through the miR-143-3p mediated TLR4/MyD88/NF-кB pathway.
GRAPHICAL ABSTRACT
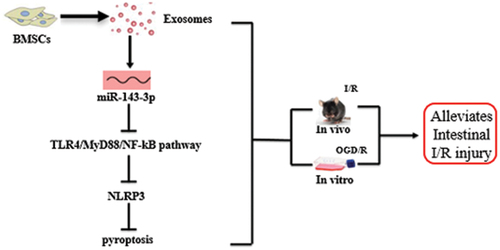
HIGHLIGHTS
BMSC-exos ameliorate intestinal ischemia/reperfusion (I/R) injury
miR-143-3p levels were reduced in I/R injury and increased with BMSC-exo treatment
miR-143-3p directly targeted and downregulated the expression of MyD88
BMSC-exos regulate pyroptosis in intestinal I/R injury via the miR-143-3p-MyD88 axis
1. Introduction
Ischemia/reperfusion (I/R) injury is a common pathophysiological process in many clinical diseases and is caused by the interruption and subsequent recovery of blood supply to the ischemic site. Reperfusion can not only fail to restore the structure and function of tissues or organs, but also aggravate the injury [Citation1,Citation2]. The gastrointestinal tract is highly sensitive to I/R injury, which can cause substantial damage to local mucosal tissues, even in cases of transient ischemia [Citation3]. Intestinal I/R injury is caused by various pathogenic factors. Its pathogenesis is complex and, given the lack of effective therapeutic strategies, mortality rates increase annually [Citation4].
Stem cell technology has developed rapidly in recent years. Mesenchymal stem cells (MSCs) have shown therapeutic effects on inflammatory response, immune regulation, tissue perfusion, and mucosal recovery in intestinal I/R injury [Citation5], which are mainly mediated by paracrine mechanisms [Citation6]. During I/R injury, the damaged tissue releases pro-inflammatory signals that activate MSCs to release beneficial paracrine factors [Citation7]. Exosomes are important paracrine components of stem cells that mediate communication between cells and determine the state of recipient cells [Citation8]. Bone marrow MSC-derived exosomes (BMSC-exos) have been shown to alleviate multiple organ dysfunction syndromes, such as acute lung injury caused by intestinal I/R injury [Citation9], though the mechanism remains unclear.
Pyroptosis, characterized by cleavage of the amino terminal of gasdermin-D (GSDMD), is a form of programmed cell death and an important mechanism of inflammation that controls the secretion of inflammasome-dependent cytokines [Citation10]. Inflammation induced by excessive pyroptosis can cause serious damage to tissues and organs [Citation11]. Pyroptosis and its related proteins occur commonly in I/R injury [Citation12]. Activation of the NOD-like receptor thermal protein domain-associated protein 3 (NLRP3) inflammasome can recruit and activate caspase-1 to induce pyroptosis [Citation13], and inhibition of pyroptosis can alleviate I/R injury in various vital organs [Citation14,Citation15]. BMSC-exos can attenuate pyroptosis induced by oxygen-glucose deprivation/reoxygenation [Citation16] and cerebral I/R injury-induced neuroinflammation and pyroptosis [Citation17].
We hypothesized that BMSC-exos alleviate intestinal I/R injury by acting on pyroptosis. Accordingly, this study evaluates the possible mechanism of BMSC-exos in the alleviation of intestinal I/R injury in vitro and in vivo. Based on microRNA (miRNA) microarray sequencing, we confirmed that BMSC-exos regulate pyroptosis and alleviate intestinal I/R injury through the miR-143-3p/myeloid differentiation factor 88 (MyD88)/nuclear factor kappa-B (NF-кB)/NLRP3 pathway. Our study provides an empirical basis for the clinical evaluation of BMSC-exos in the treatment of intestinal I/R injury.
2. Materials and methods
2.1. Animals
Specific-pathogen-free 8-week-old male C57BL/6 mice (n = 80) weighing 18–22 g were purchased from Chengdu Dossy Experimental Animals (license number: SCXK [Sichuan] 2020–030; Chengdu, China). Mice were kept at 25°C and 50–60% humidity in well-ventilated cages under a 12-h light/dark cycle, and were provided with food and water ad libitum. This study was performed in strict accordance with the Guide for the Care and Use of Laboratory Animals of the National Institutes of Health. All experimental protocols were approved by the First Hospital of Lanzhou University (No. LDYYLL2022–27). All efforts were made to minimize animal suffering and reduce the number of animals used.
2.2. Isolation and culture of BMSCs
The isolation and culture of BMSCs were performed as previously described [Citation18]. A subset of C57BL/6 mice (n = 20) were sacrificed by cervical dislocation and the bone marrow of the femur and tibia was extracted. The bone marrow fluid was centrifuged at 450 × g at room temperature for 20 min and then cultured in Dulbecco’s modified Eagle’s medium (DMEM, Cytiva, Utah, USA) containing 20% fetal bovine serum (FBS, Gibco, Grand Island, USA) at 37°C with 5% CO2. After 24 h, the suspended cells were removed, and the medium was replaced every 3 d until the cells reached ~ 80% confluence. The morphology of third-generation BMSCs was observed under a light microscope (Olympus, Tokyo, Japan).
2.3. Osteogenic and adipogenic induction of BMSCs
BMSCs at the third passage were inoculated onto 6 well plates at a density of 1 × 105 cells/well. After cells reached 80% confluence, the original medium was replaced with osteogenic (DMEM including 10% FBS, 5 μg/mL insulin, 0.1 μM dexamethasone, 0.2 mM vitamin C, and 10 mM β-glycerophosphate [Citation19]) or adipogenic induction medium (DMEM including 10% FBS, 10 μg/mL insulin, 1 μM dexamethasone, 0.5 mM 1-methyl-3-isobutylxanthine (IBMX), and 0.1 mM indomethacin [Citation20]) for two weeks to induce the differentiation of BMSCs into osteoblasts or adipocytes. The medium was replaced every 3 d. Osteogenic and adipogenic differentiation were assessed by alizarin red S staining and oil red O staining, respectively [Citation21,Citation22]. BMSCs were rinsed with phosphate buffered saline (PBS, Solarbio, Beijing, China), immobilized with 4% paraformaldehyde (Solarbio) at room temperature for 20 min, and stained with alizarin red S (Sigma-Aldrich, St. Louis, MO, USA) at room temperature for 5 min or oil red O (Sigma-Aldrich) at room temperature for 30 min. The cells were washed with PBS, stained with hematoxylin for 1 min, and washed with PBS. Images were recorded using an inverted microscope (Olympus).
2.4. Flow cytometry assays
The surface markers of BMSCs were assessed by flow cytometry as previously described [Citation23]. Approximately 1 × 106 third-passage BMSCs were collected and resuspended in 100 μL of PBS; 5 μL of antibodies, including CD29 (cat. no. 102222), CD34 (cat. no. 343527), CD45 (cat. no. 157212), and CD105 (cat. no. 323205; 1:20; BioLegend, San Diego, CA, USA) was added and incubated at 4°C for 30 min. After incubation, the cells were rinsed with PBS and centrifuged at 1000 rpm for 5 min at room temperature, and the supernatant was discarded. The cells were suspended in PBS and analyzed using a FACSCalibur flow cytometer (BD Biosciences, San Jose, CA, USA).
2.5. BMSC transfection
Third-passage BMSCs were inoculated into 6 well plates at a density of 1 × 105 cells/well and cultured at 37°C overnight. We transfected the BMSCs with 50 pmol/mL miR-143-3p mimic, negative control mimic (NC mimic), miR-143-3p inhibitor, and NC inhibitor (Guangzhou RiboBio, Guangdong, China) using Lipofectamine 2000 (Invitrogen, Thermo Fisher Scientific, Waltham, MA, USA) according to the manufacturer’s instructions. Follow-up experiments were performed 48 h after transfection.
2.6. Extraction and identification of exosomes
Exosomes of normal or transfected BMSCs (BMSC-exo, miR-143-3p mimic-exo, NC mimic-exo, miR-143-3p inhibitor-exo, and NC inhibitor-exo) were extracted using a super-centrifugation method [Citation24]. Normal or transfected BMSCs were cultured for 24 h in culture medium without exosomes (both the medium and FBS were removed by ultracentrifugation in advance), 50 mL of supernatant was collected, centrifuged at 4°C for 30 min at 2000 × g, and centrifuged again at 10 000 × g at 4°C for 45 min to remove large cell fragments and microbubbles. The supernatant was filtered through a 0.45 μm filter and the filtrate was centrifuged at 4°C and 100 000 × g for 70 min. The precipitate was dissolved in 10 mL PBS, centrifuged at 100 000 × g and 4°C for 70 min, and resuspended in 100 µL PBS. Exosomes were stained with 10 μL working solution PKH-67 fluorescence dye (cat. no. UR52303; Umibio, Shanghai, China) at room temperature for 10 min in the dark. Exosome morphology was observed by transmission electron microscopy (TEM, HT-7700, Hitachi, Tokyo, Japan); the particle size of the exosomes was determined by nanoparticle tracking analysis (NTA, N30E; NanoFCM, Xiamen, China); and the markers CD9, CD63, and TSG101 were determined by western blot analysis.
2.7. In vitro experiment
IEC-6 cells (American Type Culture Collection, Rockville, MD, USA) were inoculated onto 96-well culture plates at a density of 5 × 103 cells/well and cultured in DMEM (Hyclone) supplemented with 10% FBS (Gibco) and 1% streptomycin and penicillin (Sigma-Aldrich) at 37°C with 5% CO2. Two separate cell experiments were performed. The first experiment was divided into a control, oxygen-glucose deprivation/reoxygenation (OGD/R), and BMSC-exo group. The second experiment was divided into a control, OGD/R, WT-exo, NC mimic-exo, miR-143-3p mimic-exo, NC inhibitor-exo, and miR-143-3p inhibitor-exo group. The OGD/R cell model was established by incubating the cells with D-Hanks’ buffer (OGD medium) with 1% O2, 5% CO2, and 94% N2 for 4 h, followed by oxygenation for 6 h in normal medium at 37°C with 5% CO2 [Citation25]. After the cell model was established, each group was treated with 5 µg/mL BMSC-exo for 24 h.
2.8. Cell viability assay
Cell viability for the different IEC-6-cell groups was detected using a Cell Counting Kit-8 (CCK-8; Beyotime, Shanghai, China) at 37°C for 1 h. Absorbance was measured at 450 nm using a microplate spectrophotometer (cat. no. 1681150; Bio-Rad Laboratories, Hercules, CA, USA), to calculate cell viability.
2.9. In vivo experimental design
Two separate animal experiments were performed. First, 25 male C57BL/6 mice were randomly divided into a control, I/R model, BMSC, BMSC-exo, and BMSC-GW4869 group (n = 5 per group). GW4869 (MCE, New Jersey, USA) is a noncompetitive neutral sphingomyelinase inhibitor that acts as an inhibitor of exosome biogenesis and release. Second, 35 mice were randomly divided into a control, I/R, WT-exo, NC mimic-exo, miR-143-3p mimic-exo, NC inhibitor-exo, and miR-143-3p inhibitor-exo group (n = 5 per group). With the exception of the control group, an I/R model was established for each group. Mice were anesthetized by intraperitoneal injection of 50 mg/kg pentobarbital sodium, the superior mesenteric artery was isolated, clipped, and clamped for 45 min; the vascular clamp was relaxed, the incision was closed, and reperfusion was initiated [Citation26]. In the control group, the superior mesenteric artery was isolated, but not clipped. Mice in the BMSC and BMSC-GW4869 groups were intraperitoneally injected with 2 × 106 BMSCs suspended in 200 μL PBS or BMSCs pretreated with GW4869. Mice in the BMSC-exo, WT-exo, NC mimic-exo, miR-143-3p mimic-exo, NC inhibitor-exo, and miR-143-3p inhibitor-exo groups were intraperitoneally injected with 50 µg of BMSC-exo, NC mimic-exo, miR-143-3p mimic-exo, NC inhibitor-exo, or miR-143-3p inhibitor-exo suspended in 200 μL PBS. The control and I/R groups were intraperitoneally injected with 200 μL PBS without exosomes. All treatments were performed once after 45 min of ischemia and 2 h of reperfusion. After 24 h, the mice were sacrificed by cervical dislocation and intestinal tissues and blood were collected.
2.10. Hematoxylin – eosin (H&E) staining
Intestinal tissues were fixed in 4% paraformaldehyde at room temperature for 24 h, embedded in paraffin, and sliced. The slices were dewaxed with xylene and an ethanol gradient and then stained with H&E. Each section of tissue was evaluated by light microscopy (Olympus) using Chiu’s scoring criteria [Citation27]: 0 represents normal mucosa; 1 represents the occurrence of Gruenhagen’s gap under the mucosal epithelium in the central axis of the villi; 2 represents the elevation of the mucosal epithelium from the membrane propria and the expansion of the subepithelial gap; 3 represents the elevation of large tracts of mucosal epithelium, with most villi collapsing and some being shed; 4 represents shedding of villi and propria, dilation of capillaries, and increasing propria cell composition; 5 represents decay or digestion of propria, bleeding, or ulceration. The score was averaged over five animals per group.
2.11. Immunofluorescent staining
Intestinal tissue slices were antigen-repaired and blocked with bovine serum albumin for 1 h. Primary anti-ASC (1:100, cat. no. sc -514,414) or anti-Caspase-1 (1:100, cat. no. sc -392,736) antibodies were co-incubated with anti-NLRP3 antibody (1:100; cat. no. PA5–88709; Invitrogen) at 4°C overnight. We added the fluorescent secondary antibodies Alexa Fluor 488-labeled goat anti-mouse IgG(H+L) (1:500; cat. no. A0428) and Alexa Fluor 647-labeled goat anti-rabbit IgG(H+L) (1:500; cat. no. A0468; Beyotime Biotechnology, China) for incubation at room temperature in the dark for 1 h. Finally, nuclei were re-stained with 4,’6-diamidino-2-phenylindole (DAPI, Sigma-Aldrich) for 5 min and observed under a microscope (Olympus).
2.12. Western blot analysis
We detected the expression of marker proteins CD9, CD63, and TSG101 in BMSC-exo and claudin 1, occludin, Bcl-2, Bcl-2-associated X protein (Bax), NLRP3, apoptosis-associated speck-like protein containing (ASC), Caspase-1, cleaved-N-GSDMD, toll-like receptor (TLR)-4, p-NF-κB, NF-κB, and MyD88 in IEC-6 cells or intestinal tissues by western blot analysis [Citation28]. Total protein was isolated using radioimmunoprecipitation assay buffer (RIPA, Beyotime) and detected using a BCA protein assay kit (Beyotime). Protein samples were separated using 10% SDS-PAGE and transferred onto a PVDF membrane (EMD Millipore, Boston, MA, USA). The membranes were blocked for 1 h at room temperature in TBST containing 5% skim milk and then incubated overnight at 4°C with primary antibodies (CD9, 1:2,000, cat. no. ab263019; CD63, 1:2,000, cat. no. ab134045; TSG101, 1:2,000, cat. no. ab125011; Claudin 1, 1:2,000, cat. no. ab180158; Occludin, 1:1,000, cat. no. ab216327; Bax, 1:2,000, cat. no. ab32503; Bcl-2, 1:1,000, cat. no. ab194583; MyD88, 1:1,000; cat. no. ab219413 [Abcam, Cambridge, UK]; Cleaved-N-GSDMD, 1:1,000; cat. no. 10137; phospho-NF-κB, 1:1,000, cat. no. 3033; NF-κB, 1:1,000, cat. no. 8242 [Cell Signaling Technology, Danvers, MA, USA]; TLR4, 1:1,000, cat. no. sc -293,072; ASC, 1:1,000, cat. no. sc -514,414; Caspase-1, 1:1,000, cat. no. sc -392,736 [Santa Cruz Biotechnology, Dallas, TX, USA]; NLRP3, 1:1,000; cat. no. PA5–88709 [Invitrogen]). After rinsing with TBST three times, the membrane was incubated with HRP-conjugated secondary antibody (ab205719 or ab205718, 1:10,000; Abcam) for 1 h at room temperature. The protein bands were analyzed with an enhanced chemiluminescence detection kit (Bio-Rad) and ChemiDoc MP imaging system (Bio-Rad).
2.13. Enzyme-linked immunosorbent assay (ELISA)
The expression levels of TNF-α, IL-1β, IL-18, IL-10, TGF-β1, diamine oxidase (DAO), and myeloperoxidase (MPO) in IEC-6 cells or mouse sera (from blood centrifuged at 1500 × g for 15 min) were examined by ELISA. The ELISA detection procedure was carried out in strict accordance with the manufacturer’s instructions (Shanghai Kalang, Shanghai, China) using a double-antibody sandwich ELISA.
2.14. Dual-luciferase reporter assay
MyD88 wild-type and its corresponding mutant were built into a pGL3-basic luciferase vector (2 μg/mL; Shanghai GenePharma, Shanghai, China) to generate the luciferase reporter plasmids. Cells at the third passage were inoculated onto 24 well plates at a density of 4 × 104 cells/well and cultured at 37°C. When the cells reached ~ 70% confluence, they were co-transfected with luciferase reporter plasmids, miR-143-3p mimic, or NC mimic using Lipofectamine 2000 (Invitrogen; Thermo Fisher Scientific) according to the manufacturer’s instructions. Luciferase activity was examined using a dual-luciferase reporter assay kit (cat. no. E1910; Promega Corporation, Madison, WI, USA) following transfection for 24 h [Citation29].
2.15. Real-time quantitative polymerase chain reaction (qPCR)
Total RNA from cells or mouse samples was prepared using an RNA Sample Total RAN kit (Tiangen Biotech, Beijing, China), and cDNA was obtained using the PrimeScript RT reagent kit (Takara Bio, Beijing, China). qPCR was carried out on an Applied Biosystems 7500 Real-Time PCR system (Thermo Fisher Scientific) using SYBR Premix Ex Taq II (Takara Bio). For quantification of miRNA levels, qPCR was performed using the BulgeLoop miRNA RTqPCR Primer and BulgeLoop miRNA RTqPCR Starter kit (cat. no. C102111; Guangzhou RiboBio). Relative gene expression levels were determined using the 2−ΔΔCt method, with actin or U6 small nuclear RNA genes as endogenous controls [Citation30]. The primer sequences were as follows: MyD88 (forward: 5′-TCGACGCCTTCATCTGCTAC-3′, reverse: 5′- CCATGCGACGACACCTTTTC-3′), actin (forward: 5′- GAAGATCAAGATCATTGCTCC-3′, reverse: 5′-TACTCCTGCTTGCTGATCCA-3′), miR-143-3p (forward: 5′- GTGAGATGAAGCACTGTAGC-3′, reverse: 5′-GTGCAGGGTCCGAGGT-3′), and U6 (Bulge-Loop U6 qPCR Primer Set, cat. no. MQPS0000002-1-100; RiboBio).
2.16. miRNA microarray sequencing and analysis of differential expression
We extracted total RNA from the intestinal tissues of the control, model, and BMSC-exo groups and determined the concentration and purity of total RNA using agarose gel electrophoresis on an Agilent 2100 Bioanalyzer (Agilent Technologies, Santa Clara, CA, USA). miRNA libraries were constructed using an Illumina TruSeq Small RNA Sample Prep Kit (Illumina, San Diego, California, USA), the enrichment library was amplified by PCR, sequencing joint and index were added, and the purified library was selected by gel electrophoresis. An Agilent 2100 Bioanalyzer (Agilent) was used to compare library quality using a High Sensitivity DNA Kit (Agilent), and the purified library was quantified using a Quant-iT PicoGreen dsDNA assay kit (Invitrogen). Sequencing was performed with a HiSeq Single-End Illumina platform (USA) [Citation31]. The original sequencing data were de-jointed and quality-filtered, and the filtered sequences were de-processed. We further analyzed miRNA characteristics and expression levels. Bidirectional clustering analysis and target gene prediction were performed on the differentially expressed miRNAs using the pheatmap package (version 1.0.8) in R software (version 3.0.1; The R Foundation, Vienna, Austria); clustering was performed according to the expression level of the same miRNA in different samples and the expression patterns of different miRNAs in the same sample. The distance was calculated using the Euclidean method, and complete linkage was used for clustering. DESeq (version 1.18.0) was used to analyze the differential expression of miRNA in each sample, and the differences in conserved miRNAs were screened according to the expression of multiple differences (|log2 fold change |>1), and the significance of differences in expression (P < 0.05). Bioinformatic analysis was conducted for the predicted target genes.
2.17. Statistical analysis
All data were analyzed using SPSS 22.0 software (SPSS IBM, Chicago, IL, USA) and are presented as the mean ± standard deviation (SD). Differences between two groups were determined by an unpaired Student’s t-test, and differences among multiple groups were analyzed using a one-way analysis of variance (ANOVA) with Dunnett’s post hoc test. Statistical significance was set at P < .05.
3. Results
Using an I/R mouse model and an OGD/R cell model, we evaluated the role and potential mechanism of BMSC-exos in intestinal I/R injury based on pyroptosis. We identified miR-143-3p by miRNA microarray sequencing as a differentially expressed miRNA in the alleviation of I/R by BMSC-exos. Our in vitro and in vivo experiments indicate that the therapeutic effect of BMSC-exos is derived from the amelioration of pyroptosis through the miR-143-3p-MyD88 axis.
3.1. Extraction and identification of BMSCs and BMSC-exos
BMSCs were isolated from mouse bone marrow and cultured using the whole-cell adherent method. The third generation of cells was fusiform, fibrous, and irregular in shape (Supplementary figure S1a). Mouse-derived BMSCs had a multidirectional differentiation potential for osteogenesis (Supplementary figure S1b) and adipogenesis (Supplementary figure S1c). Expression of the markers CD29 and CD105 were positive and CD34 and CD45 were negative in the isolated BMSCs (Supplementary figure S1d). These results confirmed that the isolated cells were BMSCs. BMSC-exos were obtained by super-centrifugation; the morphology of the exosomes is shown in Supplementary figure S1e. The concentration of exosomes was 7.86 × 1010 particles/mL and the average particle size was 80.53 nm (Supplementary figure S1f). The protein concentration of the exosome solution was 2.50 μg/μL, indicating that 1 μg of exosomes contained 3.144 × 107 particles. In addition, the exosome surface markers TSG101, CD63, and CD9 were positively expressed in BMSC-exos (Supplementary figure S1g), confirming the successful isolation of exosomes from BMSCs.
3.2. Effects of BMSC-exos in the OGD/R cell model
The IEC-6 cells maintained the characteristics of intestinal epithelial stem cells and were no different from proliferative crypt cells in histology and immunology. To understand the pathogenesis of I/R, it is of great significance to study the pathophysiological changes in the intestinal mucosa under the action of drugs and various pathogenic factors. We established an IEC-6 OGD/R cell model to simulate intestinal I/R in vitro and explore the effect and possible mechanism of action of BMSC-exos. Fluorescence images of PKH-67-labeled BMSC-exos co-cultured with IEC-6 cells are shown in . Compared with the control group, cell viability was reduced in the OGD/R model (P < .001, ); the opposite trends were observed following the BMSC-exo treatment (P < .01, ). The ELISA of inflammatory cytokines showed that the expression of IL-1β, IL-18, and TNF-α in the OGD/R group was significantly higher than that in the control group; BMSC-exos reduced the expression of these indicators in the OGD/R group (P < .05, ). The western blot analysis showed that, compared with the control group, the expression of NLRP3, ASC, caspase-1, and cleaved-N-GSDMD increased in the OGD/R group, while BMSC-exos reversed the change in protein expression (P < .001, ). These results suggest that BMSC-exos can alleviate the inflammatory response of OGD/R cells, reduce pyroptosis, and ameliorate intestinal I/R injury.
Figure 1. Effects of BMSC-exo on OGD/R cell model. (a) Representative images of PKH-67 fluorescence-labeled BMSC-exo co-cultured with IEC-6 cells for 6 h,12 h, and 24 h. Magnification, ×100. (b) cells viability was detected by CCK-8 assay. (c) the expressions of inflammatory cytokines IL-1β, IL-18 and TNF-α were detected by ELISA. (d) protein expressions of NLRP3, ASC, caspase-1 and cleaved-N-GSDMD were detected by western blot analysis. (e) Densitometry analysis of the protein expression. Data were shown as mean ± SD. *P < .05, **P < .01 and ***P < .001, compared with the control group; #P < .05, ##P < .01 and ###P < .001, compared with the OGD/R model group.

3.3. Effects of BMSC-exos in I/R model mice
Histopathological results () showed that the intestinal tissue of mice in the I/R model group had significant levels of injury, BMSC, BMSC-exo, and BMSC-GW4869 alleviated intestinal injury to a certain extent, while BMSC-GW4869 inhibited the effect of BMSC (P < .05, ). The activity of DAO in the blood reflects the damage to the intestinal barrier mucosa. We found that the expression of DAO in the serum of I/R mice increased significantly, while BMSCs and BMSC-exos reversed the increase in DAO, and GW4869 inhibited the effects of BMSCs (P < .05, ). The western blot analysis showed that, compared with the control group, the expression of claudin 1, occludin, and Bcl-2 decreased, while the expression of Bax increased in the I/R group. BMSCs and BMSC-exos reversed these trends, and the effect of BMSCs was inhibited by GW4869 (P < .01, . These results suggest that BMSC-exos alleviate intestinal barrier injury and reduce cell apoptosis.
Figure 2. Effects of BMSC-exo on intestinal barrier mucosal injury in I/R model mice. (a) Hematoxylin and eosin staining of mouse intestinal tissue. Magnifications, ×400. (b) Chiu’s score of HE staining was used to evaluate pathological lesions. (c) the expression level of DAO in serum was examined by ELISA. (d) protein expressions of claudin 1, occludin, Bax and Bcl-2 were detected by western blot analysis. (e) Densitometry analysis of the protein expression. Data were shown as mean ± SD. *P < .05, **P < .01 and ***P < .001, compared with the control group; #P < .05, ##P < .01 and ###P < .001, compared with the I/R model group; &P < .05, &&P < .01 and &&&P < .001, compared with the BMSC group.

According to the ELISA, the expression of the pro-inflammatory cytokines IL-1β, IL-18, TNF-α, and MPO increased, while that of the anti-inflammatory cytokines IL-10 and TGF-β1 decreased in the I/R compared to the control group (P < .01, ). BMSCs and BMSC-exos reversed these changes in the I/R group, and GW4869 inhibited the effects of BMSCs (P < .05, ). We found that, compared with the control group, the expression of the pyroptosis-associated proteins NLRP3, ASC, caspase-1 and cleaved-N-GSDMD increased in the I/R group, whereas BMSCs, BMSC-exos, and BMSC-GW4869 reversed this increase in expression, and the regulation of BMSC was inhibited by GW4869 (P < .05, ).
Figure 3. Effects of BMSC-exo on cytokines and pyroptosis-related proteins in I/R model mice. (a-c) the expression levels of IL-1β, IL-18, TNF-α, IL-10, TGF-β1, and MPO in serum were examined by ELISA. (d) protein expressions of NLRP3, ASC, Caspase-1 and cleaved-N-GSDMD were detected by western blot analysis. (e) Densitometry analysis of the protein expression. Data were shown as mean ± SD. *P < .05, **P < .01 and ***P < .001, compared with the control group; #P < .05, ##P < .01 and ###P < .001, compared with the I/R model group; &P < .05, &&P < .01 and &&&P < .001, compared with the BMSC group.

3.4. Effects of BMSC-exos on the miRNA expression profile in intestinal tissues of I/R mice
Exosomes generally act by targeting genes via miRNAs. We determined the miRNA expression profile in intestinal tissues using a miRNA microarray. Based on the strong positive Pearson correlation coefficients of the miRNA expression levels between samples (0.8–1, ), the experiment produced reliable data and the sample selection was reasonable. The results for the clustering analysis of differentially expressed miRNAs are shown in . A principal component analysis (PCA) showed that the samples had high within-group similarity, with a discrete distribution observed for the three separate groups of samples (). According to the DESeq analysis of differentially expressed miRNA, there were 5 upregulated and 13 downregulated miRNAs between the control and I/R groups, 15 upregulated and 27 downregulated miRNAs between the control and BMSC-exo groups, and 5 upregulated and 4 downregulated miRNAs between the I/R and BMSC-exo groups (). The overlapping miRNA in the three groups was miR-143-3p (), which was significantly reduced in the I/R compared with the control group and significantly increased in the BMSC-exo group. Therefore, miR-143-3p was identified as the key miRNA for our follow-up studies. qPCR results showed that the expression of miR-143-3p decreased in both the I/R animal and OGD/R cell models, and increased in the BMSC-exo group (P < .05, ), consistent with the sequencing results.
Figure 4. The miRNA expression profile of intestinal tissues in mice was detected by miRNA microarray. (a) Pearson correlation coefficient showed the correlation between miRNA expression levels among samples. The left and upper sides were sample clustering, the right and lower sides of the figure were sample names, and different colors represent the correlation between the two samples. (b) Cluster analysis of differentially expressed miRNA. Horizontal represents miRNA and each column represents one sample. Red represents miRNA with high expression and green represents miRNA with low expression. (c) principal components analysis. The abscissa was the first principal component and the ordinate was the second principal component. Different shapes in the figure indicate different samples, and different colors indicate different groups. (d) Statistical analysis results of miRNA expression differences. The abscissa represents the comparison group for differential analysis, the ordinate represents the number of differential miRnas, the red represents up-regulated, and the green represents down-regulated. (e) Venn diagram of miRNA differential expression. The overlapped miRNA was miR-143-3p. (f-g) the mRNA expression of miR-143-3p was detected by qPCR, and the relative expression was normalized to U6 expression. Data were shown as mean ± SD. *P < .05, **P < .01 and ***P < .001, compared with the control group; #P < .05, ##P < .01 and ###P < .001, compared with the I/R or OGD/R model group.

3.5. miR-143-3p directly targeted and downregulated the expression of MyD88
Bioinformatic analysis revealed that there was a binding site between the 3′-UTR of MyD88 and miR-143-3p due to complementary base pairing (). The dual-luciferase reporter assay revealed direct binding between miR-143-3p and MyD88: The relative luciferase activity of co-transfection of MyD88 (wt) and miR-143-3p mimic was significantly decreased compared to that of MyD88 (wt) and NC mimic (P < 0.001), and no differences were observed in the co-transfection of MyD88 (mut) and miR-143-3p mimic or NC mimic (). Further analysis of the regulatory relationship between miR-143-3p and MyD88 showed that both the relative gene and protein expression of MyD88 decreased after miR-143-3p mimic treatment compared with NC mimic treatment, and its expression increased after miR-143-3p inhibitor compared with NC inhibitor treatment (P < 0.01, ). These results suggest that miR-143-3p directly targets MyD88 and negatively modulates its expression.
Figure 5. miR-143-3p directly targeted MyD88 and negatively modulated the expression of MyD88. (a) the target sites between miR-143-3p and 3‘‑UTR of MyD88 were predicted by bioinformatics websites. (b) the relative luciferase activity was detected by dual-luciferase reporter assay after transfection with miR-143-3p mimic or NC mimic. ***P < .001. (c) the mRNA expression of MyD88 was detected by qPCR, and the relative expression was normalized to actin expression. (d) protein expression of MyD88 was detected by western blot analysis. Data were shown as mean ± SD. *P < .05, **P < .01 and ***P < .001, compared with the NC-mimic or NC-inhibitor group.

3.6. BMSC-exos regulated pyroptosis in OGD/R cell models through the miR-143-3p-MyD88 axis
We treated OGD/R model cells with miR-143-3p mimic-exo or miR-143-3p inhibitor-exo and detected the mRNA expression of miR-143-3p using qPCR. The results showed that the expression of miR-143-3p increased after miR-143-3p mimic-exo treatment and decreased after miR-143-3p inhibitor-exo treatment (P < .05, ). The viability of exo-treated cells increased after miR-143-3p mimic-exo treatment and decreased after miR-143-3p inhibitor-exo treatment (P < .05, ). Similarly, the expression of IL-1β, IL-18, and TNF-α in the exo-treated cells was decreased by miR-143-3p mimic-exos, and the expression of IL-1β and IL-18 was increased by miR-143-3p inhibitor-exos (P < .05, ). miR-143-3p mimic-exos and inhibitor-exos decreased and increased the rate of pyroptosis, respectively (P < .001, ). In addition, miR-143-3p mimic-exos decreased the expression of TLR4, MyD88, p-NF-кB/NF-кB, NLRP3, ASC, caspase-1, and cleaved-N-GSDMD compared to the NC mimic-exo group, and miR-143-3p inhibitor-exos increased the expression of these proteins compared to the NC inhibitor-exo group(P < .001, ). These results suggest that BMSC-exos regulate pyroptosis in vitro through the miR-143-3p/MyD88/NF-кB/NLRP3 pathway.
Figure 6. Effects of miR-143-3p in BMSC-exo on OGD/R cell model. (a) the mRNA expression of miR-143-3p was detected by qPCR, and the relative expression was normalized to U6 expression. (b) cells viability was detected by CCK-8 assay. (c) the expressions of IL-1β, IL-18 and TNF-α in cells were detected by ELISA. (d) cell pyroptosis rate was detected by flow cytometry assay. (e) protein expressions of TLR4, MyD88, p-NF-кB, NF-кB, NLRP3, ASC, caspase-1 and cleaved-N-GSDMD were detected by western blot analysis. Data were shown as mean ± SD. *P < .05, **P < .01 and ***P < .001, compared with the control group; #P < .05, ##P < .01 and ###P < .001, compared with the OGD/R model group. &P < .05, &&P < .01 and &&&P < .001, compared with the NC mimic-exo or NC inhibitor-exo group.

3.7. BMSC-exos alleviated intestinal I/R injury in the I/R mouse model through the miR-143-3p-MyD88 axis
In vivo, the H&E results showed that miR-143-3p mimic-exos alleviated I/R injury, and the effect was greater than that of NC mimic-exos, while miR-143-3p inhibitor-exos were less effective than NC inhibitor-exos (. ASC, caspase-1, and NLRP3 fluorescence double staining showed that the expression of ASC and caspase-1 in the miR-143-3p mimic-exo group was lower than that in the NC mimic-exo group, and miR-143-3p inhibitor-exos increased their expression compared with the NC inhibitor-exo group (P < .05, , suggesting that miR-143-3p was involved in the regulation of pyroptosis by BMSC-exos. The expression of IL-1β, IL-18, and TNF-α was decreased by miR-143-3p mimic-exos and increased by miR-143-3p inhibitor-exos (P < .05, ). Furthermore, miR-143-3p mimic-exos decreased the expression of TLR4, MyD88, p-NF-кB/NF-кB, NLRP3, ASC, caspase-1, and cleaved-N-GSDMD in I/R compared with NC mimic-exos, and the regulatory effects of miR-143-3p inhibitor-exos were inferior to those of NC inhibitor-exos (P < 0.01, ).
Figure 7. Effects of miR-143-3p in BMSC-exo on I/R model mice. (a) Hematoxylin and eosin staining of mouse intestinal tissue. Magnifications, ×400. (b) Chiu’s score of HE staining was used to evaluate pathological lesions. (c) Representative images showing immunofluorescence staining of ASC (green) and NLRP3 (red) in intestinal tissues of mice. Nuclei were stained with 4,’6-diamidino-2-phenylindole (blue). Magnification, ×400. (d) Representative images showing immunofluorescence staining of caspase-1 (green) and NLRP3 (red) in intestinal tissues of mice. Nuclei were stained with 4,’6-diamidino-2-phenylindole (blue). Magnification, ×400. (e) mean fluorescence intensity in C. (f) mean fluorescence intensity in D. Data were shown as mean ± SD. *P < .05, **P < .01 and ***P < .001, compared with the control group; #P < .05, ##P < .01 and ###P < .001, compared with the I/R model group; &P < .05, &&P < .01 and &&&P < .001, compared with the NC mimic-exo or NC inhibitor-exo group.

Figure 8. Effects of miR-143-3p in BMSC-exo on pyroptosis pathway in I/R model mice. (a) the expressions of IL-1β, IL-18 and TNF-α were detected by ELISA. (b) protein expressions of TLR4, MyD88, p-NF-кB, NF-кB, NLRP3, ASC, caspase-1 and cleaved-N-GSDMD were detected by western blot analysis. (c) Densitometry analysis of the protein expression. Data were shown as mean ± SD. *P < .05, **P < .01 and ***P < .001, compared with the control group; #P < .05, ##P < .01 and ###P < .001, compared with the I/R model group; &P < .05, &&P < .01 and &&&P < .001, compared with the NC mimic-exo or NC inhibitor-exo group.

4. Discussion
Intestinal I/R injury is a common disease-related pathology that can lead to injury of distant organs, with high morbidity and mortality [Citation32]. MSCs have proven to be a powerful tool in repairing organs after I/R injury [Citation5]. The immunomodulatory, anti-inflammatory, angiogenic, and antioxidative activity of MSCs occur through paracrine signaling [Citation33]. MSCs secrete a variety of nutrient mediators, such as growth factors, immune regulatory factors, and extracellular vesicles, to promote tissue recovery and alleviate intestinal epithelial injury [Citation34,Citation35]. The paracrine mediators of MSCs are also conducive to the treatment of radiation-induced intestinal injury [Citation36,Citation37]. Exosomes, another paracrine mediator, are derived from a variety of cell types and mediate the transport of bioactive constituents between cells through endocytosis, ligand – receptor-, and plasma membrane fusion, thus facilitating intercellular communication and influencing the physiology and pathology of recipient cells [Citation38]. Compared to cellular therapy, MSC-exos are a promising cell-free alternative with reduced immunogenicity, carcinogenic potential, and excellent biocompatibility [Citation39]. BMSC-exos have shown therapeutic effects in various I/R injuries [Citation40,Citation41]. We confirmed that BMSC-exos isolated from mouse bone marrow promoted cell proliferation and reduced apoptosis in the OGD/R cell model and reduced intestinal mucosal injury and cell apoptosis in an I/R mouse model, thus alleviating intestinal I/R injury. In addition, BMSC-exos regulated inflammation and pyroptosis in intestinal I/R injury both in vitro and in vivo.
Pyroptosis, also known as inflammatory cell necrosis, is a caspase-dependent programmed cell death [Citation42]. Under the stimulation of pathogens or inflammatory factors, pattern recognition receptors (PRRs) promote the combination of ASC and pro-caspase-1 to form an inflammasome, thereby activating caspase-1 [Citation43]. Activated caspase-1 cleaves GSDMD to form a peptide containing an active N-terminal domain and cleaves the precursors of IL-1β and IL-18, resulting in pyroptosis [Citation13]. Excessive pyroptosis may aggravate disease progression [Citation44]. Indeed, pyroptosis is a potential therapeutic target for many human diseases, and the blocking of pyroptosis has been shown to alleviate inflammatory diseases [Citation45]. Inflammation and pyroptosis are closely related to the pathogenesis of I/R injury [Citation46,Citation47], suggesting that pyroptosis may play an important role in the treatment of intestinal I/R injury. In this study, we found that BMSC-exos reduced the expression of the pro-inflammatory cytokines IL-1β and IL-18 as well as that of the pyroptosis-related NLRP3, ASC, caspase-1, and cleaved-N-GSDMD in intestinal I/R injury in vitro and in vivo, confirming the therapeutic action of BMSC-exos through pyroptosis.
Exosomes are extracellular vesicles with a double lipid structure that carry RNAs (mRNA, miRNA, lncRNA, and rRNA), lipids, proteins, etc [Citation48]. miRNAs are a conserved class of small coding RNAs that elicit important physiological effects through post-transcriptional regulation of their messenger RNA targets [Citation49]. miRNAs are involved in various I/R injuries and may be key therapeutic targets [Citation50]. We found that the differentially expressed miR-143-3p was decreased in the I/R model group and increased in the BMSC-exo group. The in vitro and in vivo results were consistent with the sequencing results. A previous study confirmed that MSC-derived exosomal miR-143-3p inhibited myocardial I/R injury by regulating autophagy [Citation51]. We showed that miR-143-3p could regulate pyroptosis in vitro and in vivo to alleviate intestinal I/R injury. Bioinformatic analysis revealed a binding site between miR-143-3p and MyD88. Myd88—a core component of innate immune signaling – is a key connector molecule of the TLR signaling pathway and participates in the transmission of upstream information as well as pathogenic processes [Citation52]. We confirmed that miR-143-3p directly targeted the MyD88 promoter and downregulated its expression, suggesting that miR-143-3p regulates pyroptosis through the upstream TLR pathway.
Intestinal I/R injury mainly involves inflammation and the activation of thrombotic cascade reactions [Citation53]. I/R injury of the intestinal epithelium results in the accumulation of damage-associated molecular patterns (DAMPs) in the lamina propria [Citation54]. When DAMPs bind to TLR on the cell surface, they activate transcription factors, such as NF-κB, to promote the upregulation of proteins associated with the activation of innate immune responses [Citation55]. Many studies have demonstrated that regulation of the TLR4/MyD88/NF-κB pathway can alleviate I/R injury [Citation56–58]. We found that the protein expression of TLR4, MyD88, and p-NF-кB/NF-кB in the OGD/R and I/R model groups increased, which was reversed by the BMSC-exo treatment. NF-κB actively participates in the regulation of various cytokines during inflammation [Citation59]and is an essential transcription factor in pyroptosis [Citation60,Citation61]. NF-κB is usually isolated in the cytoplasm and binds to the regulatory protein inhibitor NF-κB (IkB). Activation of IkB by phosphorylation of IkB kinase leads to the release of NF-κB, which then translocates to the nucleus and ultimately regulates the transcription of downstream genes, including NLRP3 [Citation62–64]. Similarly, it has been proved that the transcription factor NF-κB is involved in the regulation of NLRP3 transcription in TLR-stimulated macrophages by binding the NF-κB sequence in NLRP3 promoter [Citation65]. NLRP3, a member of the inflammasome NLR family, is at the core of the inflammatory response [Citation66]. Activated NLRP3 promotes the maturation and release of IL-1β and IL18, leading to pyroptosis [Citation67]. Therefore, the TLR4/MyD88/NF-кB pathway may regulate NLRP3 to promote pyroptosis in intestinal I/R injury. Indeed, the TLR4/NF-кB signaling pathway can induce GSDMD-mediated pyroptosis of tubule cells in diabetic kidney disease [Citation68]. The present study found that the changes in miR-143-3p expression altered the expression of the TLR4/MyD88/NF-кB and pyroptosis pathways, suggesting that BMSC-exos regulate pyroptosis to alleviate intestinal I/R injury through the miR-143-3p-mediated TLR4/MyD88/NF-кB pathway.
5. Conclusions
BMSC-exos demonstrated profound therapeutic effects in intestinal I/R injury, and were associated with increased cell viability and reduced apoptosis in vitro and improved mucosal barrier integrity in vivo. BMSC-exos can relieve the inflammatory response and regulate the NLRP3-related pyroptosis pathway both in vitro and in vivo. miR-143-3p plays an important role in BMSC-exo-regulated intestinal I/R injury by directly targeting and downregulating MyD88 expression. Collectively, our findings suggest that BMSC-exos regulate pyroptosis through miR-143-3p mediated TLR4/MyD88/NF-кB pathway.
We acknowledge some limitations to our study. For example, exosomes contained not only miRNAs but also various mediators, such as lipids, proteins, total RNA, and DNA. miRNAs are intrinsic components of exosomes, which complicates the translation of effects related to increasing or decreasing levels of miRNAs. Subsequent studies will further explore the key role of different levels of miRNAs in exosomes.
Author contributions
All authors contributed to the study conception and design. WZH and ZGR conceived and designed the research. WZH and ZGR performed most of the experiments. ZY, LJP and GWW performed parts of the experiments. WZH and YY analyzed the data. WZH, ZGR and LYF wrote the manuscript. All authors agree to be held accountable for all aspects of the research and confirm that the data are accurate. All authors reviewed and approved the manuscript.
Ethics approval
All experimental protocols of the present study were approved by the First Hospital of Lanzhou University (No. LDYYLL2022–27) and all efforts were made to minimize animal suffering and reduce the number of animals used.
Supplemental Material
Download MS Word (1.1 MB)Disclosure statement
No potential conflict of interest was reported by the author(s).
Data availability statement
The datasets used in this study are available upon reasonable request to the corresponding author.
Supplementary material
Supplemental data for this article can be accessed online at https://doi.org/10.1080/21655979.2023.2253414
Additional information
Funding
References
- Kalogeris T, Baines CP, Krenz M, et al. Ischemia/Reperfusion. Compr Physiol. 2016 Dec 6;7(1):113–20. doi: 10.1002/cphy.c160006
- Golts E, Onaitis M. Commentary: Ischemia reperfusion-looking ahead. J Thorac Cardiovasc Surg. 2021 Feb;161(2):e124–e125. doi: 10.1016/j.jtcvs.2019.12.010
- Mallick IH, Yang W, Winslet MC, et al. Ischemia-reperfusion injury of the intestine and protective strategies against injury. Dig Dis Sci. 2004 Sep;49(9):1359–1377. doi: 10.1023/B:DDAS.0000042232.98927.91
- Leenarts CA, Grootjans J, Hundscheid IH, et al. Histopathology of human small intestinal and colonic ischemia-reperfusion: Experiences from human IR-models. Histol Histopathol. 2019 Jul;34(7):711–722. doi: 10.14670/HH-18-074
- Barzegar M, Kaur G, Gavins FNE, et al. Potential therapeutic roles of stem cells in ischemia-reperfusion injury. Stem Cell Res. 2019 May;37:101421. doi: 10.1016/j.scr.2019.101421
- Miceli V, Bulati M, Iannolo G, et al. Therapeutic properties of mesenchymal stromal/stem cells: The need of cell priming for cell-free therapies in regenerative medicine. Int J Mol Sci. 2021 Jan 14;22(2):763. doi: 10.3390/ijms22020763
- Oliva J. Therapeutic properties of mesenchymal stem cell on organ ischemia-reperfusion injury. Int J Mol Sci. 2019 Nov 5;20(21):5511. doi: 10.3390/ijms20215511
- Suh JH, Joo HS, Hong EB, et al. Therapeutic application of exosomes in inflammatory diseases. Int J Mol Sci. 2021 Jan 24;22(3):1144. doi: 10.3390/ijms22031144
- Liu J, Chen T, Lei P, et al. Exosomes released by bone marrow mesenchymal stem cells attenuate lung injury induced by intestinal ischemia reperfusion via the TLR4/NF-κB pathway. Int J Med Sci. 2019;16(9):1238–1244. doi: 10.7150/ijms.35369
- Kovacs SB, Miao EA. Gasdermins: Effectors of pyroptosis. Trends cell Biol 2017 Sep;27(9):673–684. doi: 10.1016/j.tcb.2017.05.005
- Tsuchiya K. Switching from apoptosis to pyroptosis: Gasdermin-elicited inflammation and antitumor immunity. Int J Mol Sci. 2021 Jan 4;22(1):426. doi: 10.3390/ijms22010426
- Wree A, Eguchi A, McGeough MD, et al. NLRP3 inflammasome activation results in hepatocyte pyroptosis, liver inflammation, and fibrosis in mice. Hepatology. 2014 Mar;59(3):898–910. doi: 10.1002/hep.26592
- Zhao Y, Shi J, Shao F. Inflammatory caspases: Activation and cleavage of gasdermin-D in vitro and during pyroptosis. Methods Mol Biol. 2018;1714:131–148. doi: 10.1007/978-1-4939-7519-8_9
- Popov SV, Maslov LN, Naryzhnaya NV, et al. The role of pyroptosis in ischemic and reperfusion injury of the heart. J Cardiovasc Pharmacol Ther. 2021 Nov;26(6):562–574. doi: 10.1177/10742484211027405
- Xu XN, Jiang Y, Yan LY, et al. Aesculin suppresses the NLRP3 inflammasome-mediated pyroptosis via the Akt/GSK3β/NF-κB pathway to mitigate myocardial ischemia/reperfusion injury. Phytomedicine. 2021 Nov;92:153687. doi: 10.1006/j.phymed.2021.153687
- Zeng Q, Zhou Y, Liang D, et al. Exosomes secreted from bone marrow mesenchymal stem cells attenuate oxygen-glucose deprivation/Reoxygenation-induced pyroptosis in PC12 cells by promoting AMPK-Dependent autophagic flux. Front Cell Neurosci. 2020;14:182. doi: 10.3389/fncel.2020.00182
- Liu XL, Zhang MM, Liu HN, et al. Bone marrow mesenchymal stem cell-derived exosomes attenuate cerebral ischemia-reperfusion injury-induced neuroinflammation and pyroptosis by modulating microglia M1/M2 phenotypes. Exp Neurol. 2021 Jul;341:113700. doi: 10.1016/j.expneurol.2021.113700
- Zhang G, Wan Z, Liu Z, et al. Exosomes derived from BMSCs ameliorate intestinal ischemia–reperfusion injury by regulating miR-144-3p-mediated oxidative stress. Dig Dis Sci. 2022 May 27;67(11):5090–5106. doi: 10.1007/s10620-022-07546-0
- Coelho MJ, Fernandes MH. Human bone cell cultures in biocompatibility testing. Part II: effect of ascorbic acid, β-glycerophosphate and dexamethasone on osteoblastic differentiation. Biomaterials. 2000 2000 06 01;21(11):1095–1102. doi: 10.1016/S0142-9612(99)00192-1
- Yao Y, Bi Z, Wu R, et al. METTL3 inhibits BMSC adipogenic differentiation by targeting the JAK1/STAT5/C/EBPβ pathway via an m(6)A-YTHDF2-dependent manner. FASEB J. 2019 Jun;33(6):7529–7544. doi: 10.1096/fj.201802644R
- Gregory CA, Gunn WG, Peister A, et al. An alizarin red-based assay of mineralization by adherent cells in culture: comparison with cetylpyridinium chloride extraction. Anal Biochem. 2004 Jun 1;329(1):77–84. doi: 10.1016/j.ab.2004.02.002
- Deutsch MJ, Schriever SC, Roscher AA, et al. Digital image analysis approach for lipid droplet size quantitation of oil red O-stained cultured cells. Anal Biochem. 2014 Jan 15;445:87–89. doi: 10.1016/j.ab.2013.10.001
- Weickert MT, Hecker JS, Buck MC, et al. Bone marrow stromal cells from MDS and AML patients show increased adipogenic potential with reduced Delta-like-1 expression. Sci Rep. 2021 Mar 15;11(1):5944. doi: 10.1038/s41598-021-85122-8
- Wu XX, Showiheen SAA, Sun AR, et al. Exosomes Extraction and Identificatiom. Methods Mol Biol. 2019;2054:81–91. doi: 10.1007/978-1-4939-9767-5_4
- Yan X, Yu A, Zheng H, et al. Calycosin-7-O-β-D-glucoside attenuates OGD/R-Induced damage by preventing oxidative stress and neuronal apoptosis via the SIRT1/FOXO1/PGC-1α pathway in HT22 cells. Neural Plasticity. 2019;2019:8798069. doi: 10.1155/2019/8798069
- Li Y, Feng D, Wang Z, et al. Ischemia-induced ACSL4 activation contributes to ferroptosis-mediated tissue injury in intestinal ischemia/reperfusion. Cell Death Diff. 2019 Nov;26(11):2284–2299. doi: 10.1038/s41418-019-0299-4
- Chiu CJ, McArdle AH, Brown R, et al. Intestinal mucosal lesion in low-flow states. I. A morphological, hemodynamic, and metabolic reappraisal. Arch Surg (Chicago, Ill: 1960). 1970 Oct;101(4):478–483. doi: 10.1001/archsurg.1970.01340280030009
- Hirano S. Western blot analysis. Methods Mol Biol. 2012;926:87–97. doi: 10.1007/978-1-62703-002-1_6
- Gu S, Jin L, Zhang F, et al. Biological basis for restriction of microRNA targets to the 3’ untranslated region in mammalian mRnas. Nat Struct Mol Biol. 2009 Feb;16(2):144–150. doi: 10.1038/nsmb.1552
- Livak KJ, Schmittgen TD. Analysis of relative gene expression data using real-time quantitative PCR and the 2(-Delta Delta C(T)) method. Methods. 2001 Dec;25(4):402–408. doi: 10.1006/meth.2001.1262
- Thomson JM, Parker JS, Hammond SM. Microarray analysis of miRNA gene expression. Methods Enzymol. 2007;427:107–122. doi: 10.1016/s0076-6879(07)27006-5
- Chen F, Wang D, Li X, et al. Molecular mechanisms underlying intestinal ischemia/reperfusion injury: Bioinformatics analysis and in vivo validation. Med Sci Monit. 2020 Dec 8;26:e927476. doi: 10.12659/MSM.927476
- Spees JL, Lee RH, Gregory CA. Mechanisms of mesenchymal stem/stromal cell function. Stem Cell Res Ther. 2016 Aug 31;7(1):125. doi: 10.1186/s13287-016-0363-7
- Qiu G, Zheng G, Ge M, et al. Functional proteins of mesenchymal stem cell-derived extracellular vesicles. Stem Cell Res Ther. 2019 Nov 28;10(1):359. doi: 10.1186/s13287-019-1484-6
- McCulloh CJ, Olson JK, Wang Y, et al. Treatment of experimental necrotizing enterocolitis with stem cell-derived exosomes. J Pediatr Surg. 2018 Jun;53(6):1215–1220. doi: 10.1016/j.jpedsurg.2018.02.086
- Chen H, Min XH, Wang QY, et al. Pre-activation of mesenchymal stem cells with TNF-α, IL-1β and nitric oxide enhances its paracrine effects on radiation-induced intestinal injury. Sci Rep. 2015 Mar 3;5(1):8718. doi: 10.1038/srep08718
- Gao Z, Zhang Q, Han Y, et al. Mesenchymal stromal cell-conditioned medium prevents radiation-induced small intestine injury in mice. Cytotherapy. 2012 Mar;14(3):267–273. doi: 10.3109/14653249.2011.616194
- Yu B, Zhang X, Li X. Exosomes derived from mesenchymal stem cells. Int J Mol Sci. 2014 Mar 7;15(3):4142–4157. doi: 10.3390/ijms15034142
- Phinney DG, Pittenger MF. Concise review: MSC-Derived exosomes for cell-free therapy. Stem Cells (Dayton, Ohio). 2017 Apr;35(4):851–858. doi: 10.1002/stem.2575
- Li X, Zhang Y, Wang Y, et al. Exosomes derived from CXCR4-overexpressing BMSC Promoted activation of microvascular endothelial cells in cerebral ischemia/reperfusion injury. Neural Plasticity. 2020;2020:1–13. doi: 10.1155/2020/8814239
- Chen Q, Liu Y, Ding X, et al. Bone marrow mesenchymal stem cell-secreted exosomes carrying microRNA-125b protect against myocardial ischemia reperfusion injury via targeting SIRT7. Mol Cell Biochem. 2020 Feb;465(1–2):103–114. doi: 10.1007/s11010-019-03671-z
- Shi J, Gao W, Shao F. Pyroptosis: Gasdermin-mediated programmed necrotic cell death. Trends Biochem Sci. 2017 Apr;42(4):245–254. doi: 10.1016/j.tibs.2016.10.004
- Broz P. Immunology: Caspase target drives pyroptosis. Nature. 2015 Oct 29;526(7575):642–643. doi: 10.1038/nature15632
- Jia C, Chen HW, Zhang J, et al. Role of pyroptosis in cardiovascular diseases. Int Immunopharmacol. 2019 Feb;67:311–318. doi: 10.1016/S0076-6879(07)27006-5
- Zheng Z, Li G. Mechanisms and therapeutic regulation of pyroptosis in inflammatory diseases and cancer. Int J Mol Sci. 2020 Feb 20;21(4):1456. doi: 10.3390/ijms21041456
- Qiu Z, Lei S, Zhao B, et al. NLRP3 inflammasome activation-mediated pyroptosis aggravates myocardial ischemia/reperfusion injury in diabetic rats. Oxid Med Cell Longevity. 2017;2017:9743280. doi: 10.1155/2017/9743280
- Wu MY, Yiang GT, Liao WT, et al. Current mechanistic concepts in ischemia and reperfusion injury. Cell Physiol Biochem. 2018;46(4):1650–1667. doi: 10.1159/000489241
- Pegtel DM, Gould SJ. Exosomes. Annu Rev Biochem. 2019 Jun 20;88(1):487–514. doi: 10.1146/annurev-biochem-013118-111902
- Dexheimer PJ, Cochella L. MicroRNAs: From mechanism to organism. Front Cell Dev Biol. 2020;8:409. doi: 10.3389/fcell.2020.00409
- Ghafouri-Fard S, Shoorei H, Taheri M. Non-coding RNAs participate in the ischemia-reperfusion injury. Biomedicine & Pharmacotherapy = Biomedecine & Pharmacotherapie. 2020 Sep;129:110419. doi: 10.1016/j.biopha.2020.110419
- Chen G, Wang M, Ruan Z, et al. Mesenchymal stem cell-derived exosomal miR-143-3p suppresses myocardial ischemia-reperfusion injury by regulating autophagy. Life Sci. 2021 Sep 1;280:119742. doi: 10.1016/j.lfs.2021.119742
- Deguine J, Barton GM. MyD88: a central player in innate immune signaling. F1000prime reports. F1000Prime Rep. 2014;6:97. doi: 10.12703/P6-97
- Subramanian S, Geng H, Tan XD. Cell death of intestinal epithelial cells in intestinal diseases. Sheng Li Xue Bao: [Acta Physiologica Sinica]. 2020 Jun 25;72(3):308–324.
- Donovan C, Liu G, Shen S, et al. The role of the microbiome and the NLRP3 inflammasome in the gut and lung. J Leukoc Biol. 2020 Sep;108(3):925–935. doi: 10.1002/JLB.3MR0720-472RR
- Nadatani Y, Watanabe T, Shimada S, et al. Microbiome and intestinal ischemia/reperfusion injury. J Clin Biochem Nutr. 2018 Jul;63(1):26–32. doi: 10.3164/jcbn.17-137
- Li S, Luo L, He Y, et al. Dental pulp stem cell-derived exosomes alleviate cerebral ischaemia-reperfusion injury through suppressing inflammatory response. Cell Proliferation. 2021 Aug;54(8):e13093. doi: 10.1111/cpr.13093
- Du Y, Qian B, Gao L, et al. Aloin preconditioning attenuates hepatic ischemia/reperfusion injury via inhibiting TLR4/MyD88/NF-κB signal pathway in vivo and in vitro. Oxid Med Cell Longevity. 2019;2019:3765898. doi: 10.1155/2019/3765898
- Zhang W, Song J, Li W, et al. Salvianolic acid D alleviates cerebral ischemia-reperfusion injury by suppressing the cytoplasmic translocation and release of HMGB1-Triggered NF-κB activation to Inhibit inflammatory response. Mediators Inflamm. 2020;2020:9049614. doi: 10.1155/2020/9049614
- Chawla M, Roy P, Basak S. Role of the NF-κB system in context-specific tuning of the inflammatory gene response. Curr Opin Immunol. 2021 Feb;68:21–27. doi: 10.1016/j.coi.2020.08.005
- Chen X, Liu G, Yuan Y, et al. NEK7 interacts with NLRP3 to modulate the pyroptosis in inflammatory bowel disease via NF-κB signaling. Cell Death Dis. 2019 Dec 2;10(12):906. doi: 10.1038/s41419-019-2157-1
- Xu S, Chen HW, Ni H, et al. Targeting HDAC6 attenuates nicotine-induced macrophage pyroptosis via NF-κB/NLRP3 pathway. Atherosclerosis. 2021 Jan;317:1–9. doi: 10.1016/j.atherosclerosis.2021.11.021
- Oeckinghaus A, Ghosh S. The NF-kappaB family of transcription factors and its regulation. Cold Spring Harbor Perspect Biol. 2009 Oct;1(4):a000034. doi: 10.1101/cshperspect.a000034
- Scheidereit C. IkappaB kinase complexes: gateways to NF-kappaB activation and transcription. Oncogene. 2006 Oct 30;25(51):6685–6705. doi: 10.1038/sj.onc.1209934
- He Y, Hara H, Núñez G. Mechanism and regulation of NLRP3 inflammasome activation. Trends Biochem Sci. 2016 Dec;41(12):1012–1021. doi: 10.1016/j.tibs.2016.09.002
- Qiao Y, Wang P, Qi J, et al. TLR-induced NF-κB activation regulates NLRP3 expression in murine macrophages. FEBS Lett. 2012 Apr 5;586(7):1022–1026. doi: 10.1016/j.febslet.2012.02.045
- Kelley N, Jeltema D, Duan Y, et al. The NLRP3 inflammasome: An overview of mechanisms of activation and regulation. Int J Mol Sci. 2019 Jul 6;20(13):3328. doi: 10.3390/ijms20133328
- Huang Y, Xu W, Zhou R. NLRP3 inflammasome activation and cell death. Cell Mol Immunol. 2021 Sep;18(9):2114–2127. doi: 10.1038/s41423-021-00740-6
- Wang Y, Zhu X, Yuan S, et al. TLR4/NF-κB signaling induces GSDMD-Related pyroptosis in tubular cells in diabetic kidney disease. Front Endocrinol (Lausanne). 2019;10:603. doi: 10.3389/fendo.2019.00603