ABSTRACT
The next milestone of synthetic biology research relies on the development of customized microbes for specific industrial purposes. Metabolic pathways of an organism, for example, depict its chemical repertoire and its genetic makeup. If genes controlling such pathways can be identified, scientists can decide to enhance or rewrite them for different purposes depending on the organism and the desired metabolites. The lignocellulosic biorefinery has achieved good progress over the past few years with potential impact on global bioeconomy. This principle aims to produce different bio-based products like biochemical(s) or biofuel(s) from plant biomass under microbial actions. Meanwhile, yeasts have proven very useful for different biotechnological applications. Hence, their potentials in genetic/metabolic engineering can be fully explored for lignocellulosic biorefineries. For instance, the secretion of enzymes above the natural limit (aided by genetic engineering) would speed-up the down-line processes in lignocellulosic biorefineries and the cost. Thus, the next milestone would greatly require the development of synthetic yeasts with much more efficient metabolic capacities to achieve basic requirements for particular biorefinery. This review gave comprehensive overview of lignocellulosic biomaterials and their importance in bioeconomy. Many researchers have demonstrated the engineering of several ligninolytic enzymes in heterologous yeast hosts. However, there are still many factors needing to be well understood like the secretion time, titter value, thermal stability, pH tolerance, and reactivity of the recombinant enzymes. Here, we give a detailed account of the potentials of engineered yeasts being discussed, as well as the constraints associated with their development and applications.
GRAPHICAL ABSTRACT
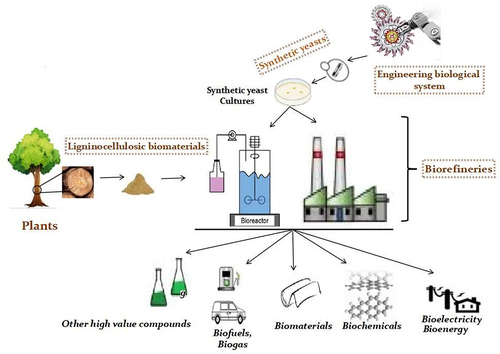
Highlights
Metabolic pathways of an organism depict its chemical repertoire and its genetic makeup.
Autonomous synthetic microbes can be developed for lignocellulose biorefinery (LCB).
LCBs can be harnessed with synthetic microbes to boost global bioeconomy.
Yeasts can be engineered to enhance downstream process of LCB.
1. Introduction
All sectors that are contributing to global economy, be it energy, agriculture, food, or industry, are facing regulatory challenges to safeguard their energy generation, usage, and their rippling effects on the environment in terms of climate change. Modern society is mostly reliant on energy sources that contribute to greenhouse gas emission, which in turn negatively affects the environment [Citation1,Citation2]. Nevertheless, improvement of living standards requires sustainable energy for meeting the world’s ever-increasing energy consumption. Hence, the quest for an energy generation/industrialization that would be environmentally friendly, renewable, and sustainable. This requires transitioning from the use of fossil-based to renewable biological resources that do not contribute to the greenhouse effect. Indeed, the attention of governments, industries, and energy sectors across the globe is now shifting to the application of renewable natural resources for energy generation and refineries with more public acceptance resulting in a boom for bio-refineries over the past few years with interesting development in renewable energy technologies and hence bioeconomy [Citation3,Citation4]. Bio-industrialization and bio-energy are thought to contribute to the reduction in the emission of greenhouse gases, create new job opportunities, and enhance regional development and energy supply chains [Citation5]. For example, the European Union (EU) set up a low carbon economy goal, for long-term environmental and economical sustainability, to be achieved by 2050. As such, the bioeconomy has taking new shape over the past few decades.
Vital bio-based compounds; biofuels and bioenergy have contributed immensely to many nations’ wealth. It is expected that in the future a larger percentage of chemicals produced globally would be derived from biological sources [Citation6]. The bioeconomy relies on a collection of industrial processes and products that make use of microorganisms that break down woody plants to produce biofuels and bioproducts. Microorganisms, specifically white-rot fungi, play a number of roles in many biotechnological applications including biorefineries [Citation7] and the degradation of lignocellulosic materials with successive product utilization to give rise to a value-added biofuel and other bio-goods. In fact, there has been an upsurge in the application of bio-based materials, such as the plant’s lignocellulosic biomass/biomaterials (LCBs), in biorefineries. The lignocellulose feed-stocks including barley straw, corn stover, wheat straw, corn fiber, switch-grass, and many other plant-derived materials have been successfully used as substrates for the production of various viable chemicals and biofuels [Citation8,Citation9]. The high levels of lignin, cellulose, hemicellulose, and sugars make them suitable for microbial growths since they are good substrates for the fermentation process that is very important for biorefineries [Citation10,Citation11]. The conversion of LCBs to valuable products often follows this sequence of processes: (i) biomass pretreatment, (ii) enzymatic hydrolysis, (iii) microbial fermentation, and (iv) purification (also called downstream process). Many innovative technologies have been developed to enhance the first and second stages of biomass conversion to enhance and hasten the downstream process.
With the advancement of modern biotechnological techniques, the application of synthetically/genetically engineered organisms for enhanced bio-refineries of plant biomass has been viewed with great potentials [Citation12]. Through synthetic biology and genome engineering tools, it is now possible to develop synthetic enzymes, like those expressed in white-rot yeasts [Citation13], that efficiently break down lignin and other components of cellulosic materials. Genetically engineered yeast strains host enzymes that confer properties such as enhanced tolerance, increased metabolic flexibility, and fermentation efficiency [Citation14,Citation15]. The conversion of LCB into bioproducts by synthetic yeasts with enhanced enzyme properties has led to more efficient lignocellulose biorefineries (LB) [Citation16–18]. However, the majority of these laudable findings on the engineering of yeast strains for LB are limited to the laboratory and not many of these new strains have been exploited industrially. The optimization of microbial whole-cell biocatalyst has contributed to the success in biorefineries. Many yeast cells have become essential components in food industries, be it in bread, wine, beer, or beverage making. Also, yeasts have proven valuable in many biotechnological applications and play vital roles in the overall biorefineries [Citation7]. The conversion of LCBs by microbes that are more tolerant to hash environment, with wide substrate specificity and well-structured metabolic mechanism is now desired for achieving more efficient LB [Citation19]. This is possible through synthetic biology and genome engineering tools applied to yeasts that are the most suited for this purpose due to their genetic diversity [Citation12]. Great success has been made in biofuel and biodiesel production by increasing the metabolic flexibility and fermentation efficiency of many yeast strains like the popular the baker’s yeast (Saccharomyces cerevisiae) [Citation14–18]. S. cerevisiae is now being employed in different bioprocesses for the generation of food, biochemicals/biofuels, while other yeasts have also been exploited. Candida guilliermondii, for instance, has been used for the synthesis of xylitol; Yarrowia lipolytica for lipid production; and Ashbya gossypii for generating riboflavin (Vitamin B2) [Citation20–22].
This review aims to discuss in depth lignocellulose and its biorefinery that have led to the synthesis of many important bio-based products. We also highlight the roles of lignocellulolytic enzymes in biorefineries and the various metabolic engineering strategies of white-rot yeasts. Hence, we advocate the exploitation of new biotechnological tools for the creation of new synthetic white-rot yeasts. The ability to use them on a large scale industrially might be a primary driver of a sustainable biorefinery and bioeconomy.
2. Lignocellulose biomass for production of bio-based products
2.1. Structure and properties of lignocellulose biomass
The LCBs are plant-based raw and renewable natural resources (grasses, energy-rich crops, forest residues, and agricultural residues/by-products) used for the production of biofuels, such as bioethanol [Citation23,Citation24]. The structure of plant biomass is dominated by cellulose, hemicellulose, and lignin macromolecules. Reports on the relative distribution of lignocellulose components in various plants revealed some differences. This distribution varies depending on environmental factors such as water availability and temperature [Citation25], plant ontogenesis and plant type [Citation26], and the adopted quantitative method [Citation27]. For instance, Rabemanolontsoa et al. [Citation27] found 579 and 681 g of Holocellulose (Cellulose and hemicellulose) per kg of corn leaves and cob, respectively. They also found that these components vary to 513 and 667 g/kg holocellulose in corn leaves and cob analyzed differently. Cellulose content was shown to be different in Saccharum spontaneum and Arundo donax (approximately 35 and 39%, respectively [Citation28]). Comparative studies on S. spontaneum biomass components revealed varying cellulose contents of 14% [Citation28] and 20% [Citation29]. The biomass of Saccharum spontaneum L. was characterized for bioethanol production by Scordia et al. [Citation29]. They found differences in its components as 40% cellulose, 30% hemicellulose, and 25% lignin.
Cellulose, discovered by French chemist, Anselme Payen in 1838 is the most abundant form of polysaccharide molecule made of D-glucose units and reaches a length of 10,000 daltons [Citation30–32]. Among the major cellulose polymers found in wood plants, there are glucose, xylose, arabinose, galactose, and mannose. Cellulose (along with hemicellulose) is a major structural component of plant cell walls. Hemicellulose is made of many different molecules, the majority of which have a carbohydrate structure. It is a single, branched-chain amorphous non-homogeneous polysaccharide attached with fibrils by non-covalent bond cross-links [Citation33–36]. Hemicellulose, xylose, and arabinose are the most common molecules found in plant cell walls. The major components in wood are xylose and arabinose. Recent studies have shown that the amorphous form of cellulose can be easily decomposed by enzymes and chemical (re)agents due to a poor bonding network [Citation37–39]. Hemicellulose is the second most abundant carbohydrate in plant LCBs having non-crystalline and random structures [Citation40,Citation41]. Lignin is as an aromatic biopolymer that is renewable and abundant in the world (just after cellulose) [Citation26,Citation27]. It is a cross-linked hard, heavy polymer composed of various chemical compounds that are bound together [Citation39].
Lignin is an irregular and non-crystalline three-dimensional structure consisting of phenylpropane units randomly and non-linearly linked together. The three major monomeric units in lignin are sinapyl, coniferyl, and coumaryl alcohol [Citation42,Citation43]. It consists of propane units (hydroxylyzaldehyde propionic acid and phenolic propionaldehyde), syringol, and guaiacyl. Lignin accounts for approximately 25–30% of lignocellulose. The average amount of lignin in grasses, eucalyptus, and other cellulose-rich crops is 28% (by weight), whereas it is lower (20%) in wood. Previous studies have shown that hemicellulose and cellulose in LCB account for approximately 70% of the total biomass and contribute to biomass recalcitrance through the formation of covalent and hydrogen bonds with lignin [Citation43]. The effective isolation of lignin from the LCB depends on their structure and function [Citation44], this normally starts the pre-treatment process, then lignin isolation for manufacturing adhesive and/or bio-based materials. Lignin depolymerization (secondary refining) is often done by hydrothermal liquefaction to further products. In Europe, the lignin-based products accounts for 34.0% of the total plant-based-materials market share, while the global lignin market size is predicted to increase drastically by 2027. The market size is more than 954.5 million USD presently [Citation44], thus innovations in innovation in lignin bio-refinery would play an important role toward the utilization of greener renewable energy.
In summary, the cellulose, hemicellulose, and lignin are mixed together (heteromixed) in plant biomass while their proportion/amount may vary depending on the type, source, or plant species [Citation45]. show both the structures of these three polymers and their compositions in popular plant materials.
Table 1. Chemical composition and structure of lignin, hemicellulose, and cellulose in plant’s cell walls.
Table 2. Chemical composition and/or concentration of cellulose, lignin, and hemicellulose in some lignocellulose plant materials.
3. Roles of lignocellulose biomass in global bioeconomy
The production and supply of fossil-based hydrocarbon fuels, chemicals, and additives are under pressure in many parts of the world due to the limited sustainable petroleum resources, the rising costs, and the need for less harmful alternatives. One of the several trends in the development of value-added bio-based products is the use of LCB feedstocks as a source of industrial chemicals [Citation37,Citation38].
he LCBs are renewable low-cost materials, classified as follows: 1) agricultural residues (e.g. sugarcane bagasse, wheat and oak straw, rice husk and straw, barley straw, corn stover, and corn cobs), 2) hard and soft wood residues (pinewood, spruce and poplar, eucalyptus, Douglas fir), and 3) dedicated energy crops (switch and timothy grass), with the potential as a feedstock for bio-based chemical production (biofuels) as shown in [Citation57,Citation58]. Among the reported applications of LCB there are liquid transportation fuels [Citation59], gasoline additives [Citation60], plasticizers or additives to plastics [Citation61], feedstock for commodity and bio-chemicals [Citation62–64], raw materials for food or nutraceuticals [Citation65].
3.1. Biofuel
Biofuels are major products of LCB with a great potential to address the global challenge of energy generation due to decreasing fossil fuels [Citation66]. Biofuels can be generated from energy feedstocks from food-based crops (first generation) and non-food-based crops (second generation). While the food-based feedstocks provide sugar, starch, and vegetable oils, the non-food-based feedstocks mainly comprise LCBs with high carbohydrate composition. As the world is moving toward a carbon-neutral environment, biofuels will serve the purpose of reducing the atmospheric carbon emission. The relatively small amount of nitrogen, sulfur, and ash in LCB makes biofuel cleaner compared to the fossil one and other non-conventional oils.
Because plant biomass is abundant all over the world, biofuels from LCBs are renewable energy sources. Due to its high hydrogen content, a biofuel is clean for transportation and has a low flammability [Citation67]. However, there are several limitations in the process of converting lignocellulose into fuel. One of the most widely used techniques to transform lignocellulose into sugars, which are used to produce biofuels, is chemical hydrolysis with high acid concentration. According to Zhou et al. [Citation68], this hydrolysis process has several drawbacks such as a high temperature reaction and a high energy consumption.
The goal of the biorefinery of biofuels from LCBs is to reduce the consumption of synthetic chemical compounds that harm the environment, humans, and animals. Ideally, they are replaced with similar compounds that perform the same functions but are more environmental-friendly and less expensive to use in manufacturing and industrial processes. Eco-friendly and sustainable research is being conducted with biological agents capable of converting lignocellulose into other valuable products. Biological treatments using Coniophora puteana, Postia placenta [Citation69], and Trametes versicolor [Citation70] achieved an improved hydrolysis by breaking lignocellulose covalent bonds into simpler molecular compounds. This procedure only requires a moderate temperature and a low acid concentration.
3.2. Bioethanol
Bioethanol is a biomass-based renewable fuel that does not require combustion. Bioethanol is made by fermenting sugar derived from sugar crops or sugar-rich biomass [Citation71]. Wheat, sugar beet, and sugar cane are currently the primary sources of bioethanol, accounting for more than 760, 252, and 1869 million tons of global consumption, respectively [Citation72]. Annual bioethanol production is increasing, and global bioethanol production and consumption are expected to exceed the 134.5 billion liters projected for 2024 [Citation73]. In recent years, various applications of bioethanol have been researched. Studies have shown that using bioethanol as a fuel additive improves the performance, emission, and combustion of common diesel engines, which can result in significant reductions in the emission of either smoke and carbon monoxide [Citation74] or smoke and nitrous oxide [Citation75] compared to diesel fuels. With an increasing population and a scarcity of fossil fuels, bioethanol can help to make fuels more abundant and affordable.
Bioethanol is the fastest growing renewable-energy sector, where production is increasing at a rate of nearly 10% per year [Citation76]. Global bioethanol production is more than doubled between 1990 and 2003, and it is expected to be on a steady increase yearly [Citation77]. This increase is on the rise as both the global economy shifts toward, and the world focuses on, sustainable energy sources. On the whole, bioethanol production can help reduce greenhouse gas emissions [Citation78], preserve natural habitats, improve air quality, and even lower energy bills [Citation79].
3.3. Bioplastics
As the world becomes more aware of the danger of plastic, the search for plastic alternatives becomes more important. The use of biodegradable plastic obtained from LCBs has been identified as a potential solution to this problem [Citation80]. The scientific community is currently focused on the process of converting plants [Citation81] and food wastes [Citation82] into plastic. This is no longer a pipe dream but is quickly emerging as the most sustainable option for plastic production. The ability to get plastic from lignocellulose resources is changing the way plastic is synthesized by utilizing natural materials – ranging from barley to silvergrass [Citation81]. According to Bhatia et al. [Citation81], LCBs is first turned into sugars that are fermented into chemicals and finally converted into biodegradable plastic. In theory, this kind of plastic would break down after decomposition. Using the most recent technology, renewable plastic with the required properties is likely to be available in the near future. It will be produced at a faster rate and at a lower cost than petroleum-based plastic, opening up an entirely new market for plastics within the next few years. Companies are already studying these processes and are only a few years away from putting this technology to use in industries [Citation81].
3.4. Bio-based chemicals
The LCBs are a renewable resource for making a variety of bio-based materials, chemicals, or energy [Citation83]. Lignocellulose plant biomass is thought to be a potential alternative to the fossil or petroleum-based chemicals for the production of biological-based chemicals. Many commonly used and industrially relevant chemicals are derived from nonrenewable fossil resources and are associated with serious environmental concerns. The primary goal of biorefinery is to produce chemicals that are more sustainable than traditional chemicals with various technologies [Citation83]. From LCBs, biorefineries can directly produce a range of diverse chemicals. The discovery of enzymes and microorganisms (bacteria, yeast, or fungi) that efficiently degrade lignocellulose into simple sugars for bioproduction of bio-based chemicals is driving this technology.
Few studies have recently demonstrated the use of various LBs for production of bio-based products like glucose (from cellulose/hemicellulose) [Citation84], mannose, galactose, and xylose (from cellulose) [Citation85], as well as phenolic compounds (from lignin) [Citation86]. Researchers have also reported some polymeric contents in hemicellulose component of some agricultural products such as rice husk and corn stover these components can be used to obtain a variety of marketable and renewable bio-based chemicals like benzene, biphenyls, catechol, cyclohe, guaiacols, phenols, syringaldehyde, vanillic acid, vanillin, and xylitol [Citation87,Citation88].
3.5. Pulp and paper making
The LCBs can also be used to make pulp and paper. Recent research evaluated and validated the suitability of LCB as a raw material applicable in the pulp and paper industries [Citation89]. As a result, many discoveries revealed that the process of producing pulp and paper involves three steps: pulping, bleaching, and paper production [Citation90,Citation91]. Chemical pulping is the separation of lignin from fibers using alkaline chemicals at high temperatures. A large amount of toxic chemicals is released into the environment at this stage [Citation92,Citation93]. However, an alternative process known as bio-pulping can be used to reduce the toxic effects of the chemicals. Bio-pulping provides a cost-effective and environmental-friendly alternative to overcome challenges associated with pulp and paper manufacturing. Bio-treatment with white rot fungi to metabolize lignin and separate fibers for paper production has been reported [Citation93]. Bio-pulping, which involves the introduction of specific LMEs at various steps in making pulp making before bio-bleaching enhances significant energy savings while also lowering pollution [Citation90,Citation94,Citation95].
3.6. Animal feed production
Enzymatic production of feed by solid-state fermentation using microbes and a variety of LCBS has been recognized as a potential and adequate way to enhance animal rations [Citation92,Citation93]. According to Jabri et al. [Citation93,Citation94], the integrated bioprocessing of partially digested and enzyme-enriched sunflower heads proved to be a valuable feed in animal food rations. Furthermore, Abid et al. [Citation92] concluded that the treatment of crude olive cake and leaves by a fungus by Trichoderma longibrachiatum enhances them with an exogenous fibrolytic enzyme and turns to a low-cost feedstock for animal nutrition. According to Yang et al. [Citation96], most significant changes in the composition of spent straw substrate after treatment for animal feed are a reduction of 17% in hemicelluloses, 15% in cellulose, 4% in lignin, and 60% in gossypol [Citation97,Citation98]. However, recent research has shown that with some notable polysaccharides, vitamins, and trace elementsfor example, Fe, Se, Mo, Ca, Zn, and Mg, they are valuable components that can be added to help animals digest feedstuffs [Citation97].
4. Schemes for obtaining lignocellulose-based products
Since the last decade, various researchers have described the conventional method for producing biofuels – and other value-added chemical products – from plant biomass in three stages, namely: (a) pre-treatment of crop plant material for lignocellulose; (b) enzymatic decomposition of lignocellulose into sugars; and (c) conversion of monosaccharides or disaccharides into products.
4.1. Pre-treatment methods
In order to release fermentable sugars from LCBs, highly efficient pre-treatment methods are required prior to enzymatic hydrolysis. Lignin is a cross-linked polymer that physically connects hemicellulose and cellulose. The chemical structure of various lignins can differ. Hence, pre-treatments tailored to the specific chemical structure of lignin are necessary [Citation96,Citation99]. Moreover, to obtain maximum yields of fermentable sugars from lignocellulose under different conditions, a pre-treatment method shall be optimized. The primary goals of pre-treatment are improving solubilization, modifying accessible surface area, and increasing biodegradability [Citation100].
A number of pre-treatment techniques to alter the structure of lignin have been developed for different purposes. There are three types of pre-treatment technology: physical, chemical, and biological. An ideal pre-treatment method should be simple, quick, and energy-efficient, with a high enzymatic hydrolysis yield. Chemical treatments based on acetic acid, steam explosion [Citation101,Citation102], dilute sulfuric acid, and alkaline peroxide [Citation103] have been reported to be commonly used. According to Cebreiros et al. [Citation101], steam explosion of Eucalyptus grandis biomass resulted in approximately 80% glucan enzymatic hydrolysis from pre-treated solids to fermentable sugars. Furthermore, biological treatment has been widely reported as an environmentally friendly pre-treatment method. Certain microorganisms found in nature have cellulolytic and hemicellulolytic abilities. According to research conducted with Aspergillus niger, A. sojae, and A. terreus, the fungal strains ensure biodegradability (about 16.6%) of rice straw for bio-methane while protecting the environment and requiring less energy [Citation104].
4.2. Enzymatic hydrolysis
Several studies have emphasized the significance of the enzymatic hydrolysis process in converting the sugars in LCBs into fermentable sugars that can be used directly as a fuel or in the synthesis of bio-based chemicals [Citation105,Citation106]. Enzymatic hydrolysis of lignocellulose into fermentable sugars enables the production of bio-based by-products in an environmental-friendly and cost-effective manner. The fermentability of the pre-treated plant biomass determines the economic attractiveness of this process. Clostridium thermocellum is well known for its efficient and robust ability to hydrolyze a wide range of plant polysaccharides [Citation107–109]. In addition to the high cellulolytic efficiency, C. Thermocellum has demonstrated the ability to use plant-derived carbohydrate complex polymers in industrial processes [Citation110,Citation111]. A variety of enzymes, including glucanases, glycosidases, chitinases, amylases, and xylanase, have been discovered in the genome of C. thermocellum [Citation112]. Other research has found that exoglucanase and xylanases, among other enzymes, help the conversion of plant cell wall to sugars [Citation113,Citation114]. These enzymes are modular glycosyl hydrolases with a four-domain architecture that share a common structural core [Citation11,Citation115]. Basit et al. [Citation116] described the catalytic domains of xylanases. They include a β-barrel domain that can bind cellulose/hemicellulose and an adjacent catalytic domain that binds to the terminal regions of a polysaccharide chain to break glycosidic bonds. The linker region between the domains contains conserved segments with glycosyl hydrolase-like motifs. C. thermocellum enzymes contain several different modules that facilitate interactions with substrates [Citation117,Citation118]. According to another study, these enzymes have three types of domains: a catalytic domain, a carbohydrate-binding module, and a docker in domain [Citation119].
Fermented sugars produced by enzymatic hydrolysis are important monomers used in producing biofuels and other industrial important products.
4.3. Conversion of biomass
The different conversions of LCBs are solely determined by the desired bio-based products [Citation58]. Until recently, two distinct methods for converting sugars into useful bio-based products were used (): the thermochemical and biochemical methods [Citation58]. Thermochemical conversion of biomass involves heating the biomass in the absence of oxygen (anaerobic conditions), which causes significant side reactions such as the oxidation of sugars to less valuable compounds. Typically, thermochemical degradation of biomass has been associated with a very limited set of products, primarily simple and highly oxygenated hydrocarbons [Citation120].
Figure 1. Thermochemical and biochemical conversion route of lignocellulosic biorefinery to value added bio-based products. SSF: simultaneous saccharification and fermentation, SHF: separate hydrolysis and fermentation, SSCF: simultaneous saccharification and co-fermentation, CBP: consolidated bioprocessing.

Combustion, carbonization/torrefaction, gasification, pyrolysis, and liquefaction are all examples of thermochemical processes [Citation58]. Nanda et al. [Citation57] demonstrated the conversion of wheat straw into hydrogen fuel in the context of gasification. To drive the reactions, the biomass is heated in the presence of a hot, inert gas. However, the design of the required reactor is difficult [Citation121]. Biomethanation has recently been demonstrated as an alternative method. Previous research has provided insight into how solid biomasses are exposed to high H2 (injected pressure) and the CO2 generated from the anaerobic digestion process are converted into biogas [Citation122,Citation123]. However, Dar et al. [Citation124] identified some drawbacks to this process, including the energy-intensive, costly, and polluting substrate pre-treatment. Liquefaction is simpler and more robust in converting solid biomass to liquid (BTL). This technology enables biomass liquefaction in the presence of water at high pressure [Citation125]. It is also an example of an in situ bioconversion process for liquid fuel production [Citation126].
The biochemical method is the use of microorganisms to convert sugars into useful chemicals such as ethanol, lactic acid, or butyric acid, which can then be used to produce a variety of value-added bio-based products [Citation127]. This conversion necessitates the use of biomass free of inhibitory components, such as those found in the lignocellulose fraction. Lignin is thought to be important in inhibiting lignocellulose hydrolysis [Citation24].
Biochemical conversion methods include a fermentation method, which uses microorganisms capable of converting LCBs into useful substances [Citation128], and a hydrolytic method, which uses a chemical reaction achieved by exploiting chemical acids to hydrolyze components in the biomass feedstock and a microorganism capable of reducing these acids [Citation129]. In each case, sugars are converted into chemical intermediates, such as alcohols. In comparison, the intermediates of the biochemical method are less expensive than those of the thermochemical method [Citation130], which makes biochemical conversion more appealing. The biomass components (cellulose and hemicellulose) are first broken down into component sugars by cellulolytic enzymes which are further fermented to bioethanol by a series of biochemical processes [Citation58]. Furthermore, biochemical methods have low efficiencies with longer reaction times, and some organic compounds, such as lignin, cannot be completely decomposed.
Various techniques (including biochemical, thermochemical, biotechnological, and physiochemical methods) have recently been used to convert biomass into various energy forms (e.g. fuel and heat), bio-based chemicals, and other value-added products [Citation58]. These according to Cheah et al [Citation127] include different steps with different importance as highlighted in .
Table 3. Pre-treatment methods for lignocellulosic biomass conversion to bio-based products advantage and disadvantages as adapted from Cheah et al. [Citation66].
Good or bad performance regarding cost, toxic byproduct formation, and applicability are marked by √ and x, respectively
Several studies have used fermentation to convert biomass carbohydrates into bioethanol and/or other chemicals. For example, glucose-containing biomasses are fermented to ethanol, which is then combined with other materials in downstream tanks to form solutions with higher ethanol concentrations [Citation131]. One or more of these solutions can then be extracted as ethanol, or the ethanol product can react further to form additional chemicals. To produce chemicals, a variety of biochemical conversion technologies such as gas fermentation, gas-solid fermentation, heterogeneous liquid phase fermentation, and solid-phase fermentation can be used. The best that has been reported is the combination of various biological – chemical/physicochemical pre-treatment strategies. Meenakshisundaram et al. [Citation130] gave a full compilation of the importance of this combined treatments as shown in .
Table 4. Overview of production of bio-based ethanol from lignocellulose biomaterials, combined treatment strategies, and yield efficiency as compiled by Meenakshisundaram et al. [Citation130].
5. Lignocellulosic biorefineries and the roles of lignocellulolytic enzymes
Biorefinery is regarded as one of the most important green chemistry and bio-based solution for sustainable development strategies. The goal of bioprocesses is to convert lignocellulosic waste into energy-producing products while also reducing their environmental impact. Enzymes are macromolecules produced by microorganisms that can speed up biological/biochemical reactions when added to a system. They facilitate and encourage the conversion of substrates into useful end products by providing the right circumstances for reactions to occur. Hydrolases, isomerases, lyases, ligases, oxidoreductases, and transferases are the six primary types of enzymes. The lignin modifying enzymes (LMEs) or simply ligninolytic enzymes (LE) are oxidoreductases, whereas the hydrolytic enzymes are hydrolases [Citation90].
Many microorganisms revealed to have a great potential in depolymerizing cellulose, hemicellulose, lignin, and other plant materials. They can be exploited for LBs due to their capability to produce oxidoreductase enzymes. The carbohydrate active enzymes of the CAZy family (CAZy; http://www.cazy.org/) are a group of lignin-depolymerizing auxiliary activity (AA) enzymes that are regarded as the most powerful natural oxidative enzymes (see supplementary Table S1). The most common LMEs are those in 1) the multicopper oxidase superfamily (AA1), which laccases (Lac) belong to, and 2) the auxiliary activity family (AA2) that includes lignin peroxidase (LiP), manganese peroxidase (MnP), and versatile peroxidase (VP). Another group is the peroxidase superfamily that contains dye decolorizing peroxidases (DyPs) and heme-thiolate peroxidases (HTPs). The auxiliary active enzyme family AA3 includes cellobiose dehydrogenases (AA3_1) and aryl alcohol oxidases/dehydrogenases (AAO), galactose oxidases (GAO), glucose dehydrogenases, glucose oxidases, and pyranose dehydrogenases (PDH – all AA3_2). AA3_3 comprises alcohol oxidases (AOx), like vanillyl alcohol oxidase (VAO), and benzoquinone reductase, while AA3_4 consists of pyranose oxidase and methanol oxidase (MOx). The superfamily AA4 is made of vanillyl alcohol oxidases; AA5 corresponds to galactose oxidase (GOX), raffinose oxidase, glyoxal oxidase (GLOX), and alcohol oxidase enzymes. These enzymes have different characteristic reactions in diverse media (see supplementary Table S2). The most common LMEs secreted by fungi are the AA1 and AA2 superfamilies, i.e. the heme-containing peroxidases that are capable of catalyzing the oxidation of lignin, complex hydrocarbons, and phenolics with hydrogen peroxides as co-substrate.
Many microorganisms have been reported to utilize and degrade lignocellulosic waste into energy-yielding products in order to reduce or eliminate their harmful environmental impacts [Citation132–134]. Microorganisms that are used in lignocellulose biodegradation are classified according to the lignocellulose component they can degrade while the majority of cellulolytic enzymes identified to date come from bacteria, fungi, or yeast. Fungi are the most well-known organisms for degrading lignocellulose into its three main polymers. Due to the insoluble nature of the substrates, both bacterial and fungal breakdown must take place outside the cell [Citation135]. This has been well established by many groups of higher fungi, like the white rot fungi (WRF) or brown rot fungi (BRF) which are from the group; Ascomycetes, Basidiomycetes, and Deuteromycetes. Many of these fungi are involved in the degradation and/or fermentation of hemicellulose, cellulose, and/or lignin was reported [Citation136]. Schizophyllum, Penicillium, Aspergillus, Trichoderma, Sclerotium, and Phanerochaete are among the lignocellulolytic fungi that have been found to be capable of producing large amounts of enzymes extracellularly [Citation137]. It has been perceived that producing lignocellulolytic bacterial enzymes has several advantages: bacteria grow faster than fungal equivalents, they have more specialized and functional multi-enzyme complexes and can adapt to a wider range of environmental challenges [Citation138]. Bacteria are regarded as key providers of important enzymes and metabolites as well as being genetically versatile. However, many uncommon bacterial genera are yet to be exploited for their industrial and biotechnological abilities. As a consequence, further research into certain ecological conditions may lead to the discovery of novel groups that produce metabolites that could one day be the key contributors in green technology [Citation139]. Extracellular enzymatic systems are known to exist in microorganisms (). They have a hydrolytic system that produces hydrolases to break down hemicellulose and cellulose. They also have a lignin depolymerization machinery that is both oxidative and extracellular [Citation135]. Furthermore, several studies have found that Sphingomonas [Citation140], Acinetobacter [Citation141], and Cellvibrio [Citation142] form monophyletic groups in terms of xylan degradation ability. Some yeasts have been shown to degrade cellulose and xylan [Citation143].
Many fungal cellulases have been proposed for cellulose degradation as the most active cellulases are found in filamentous microorganisms. The cellulose component of plant cell walls and vegetable stalks, for example, can be completely degraded by fungi. A cellulase enzyme isolated and purified from Rhizopus oryzae has been reported to be useful for the enzymatic digestion, dissolution, and hydrolysis of cellulose materials [Citation144]. Bacteria from the phyla Actinobacteria [Citation145,Citation146], Proteobacteria [Citation147], and Bacillota also produce many cellulases [Citation148]. Cellulases isolated from bacteria include β-1,4-endoglucanase from Cellulomonas telluris [Citation149], Bacillus and γ-Protobacteria [Citation133]; Cellulose 1,4-beta-cellobiosidase from Sporocytophaga myxococcoides and Cohnella sp [Citation150]; and xylanase from Pseudomonas mohnii [Citation151].
There are three distinct stages in the transformation of lignocellulose into polymers: pre-treatment, enzymatic hydrolysis by cellulolytic enzymes, and saccharification through fermentation. Lignocellulose must be pre-treated such that it is degraded by various classes of enzymes into fermentable sugars. Eventually, lignocellulose is broken down into simple sugars that can be easily fermented to ethanol [Citation152]. The release of fermentable sugars is the primary goal of lignocellulosic substrate biorefining. This process is primarily accomplished through lignocellulose depolymerization, which is catalyzed by proper enzymes. Cellulases are the most common enzymes used in industry [Citation153]. They are classified into five major groups based on the type of reaction catalyzed:
Endocellulases, which break down the β-1,4-polysaccharide linkages in cellulose to release cellodextrins [Citation154]:
Exocellulases, which depolymerize amorphous regions of cellulose, resulting in the formation of various oligomers, such as cellobiose [Citation155];
β-glycosidases, which hydrolyze β-glycosidic bonds in one of the intermediates formed by the action of exoglucanases and lead to the synthesis of D-glucose and D-glucose 6-phosphate [Citation156];
Oxidative cellulases, which depolymerize cellulose;
Cellulose phosphorylases, which depolymerize cellulose by using phosphates [Citation157].
The main functions of lignocellulolytic enzymes in lignocellulose biotransformation include polysaccharide degradation, lignin depolymerization, cellulose, and hemicellulose degradation. Future research might look at how the combination of lignocellulose-degrading enzyme complexes enhances bioconversion. Because of the complexity of lignocellulolytic enzyme complexes, combining lignocellulolytic enzymes with different functions could speed up lignocellulose bioconversion.
6. Metabolic engineering expression of lignocellulolytic enzyme consortia in yeast hosts
Various strategies have been reported as a way forward toward the development of yeast strains for sustainable biorefineries using cost-effective biomass. They include: randomized mutagenesis, genome editing, QTL analysis, whole genome (re)sequencing, evolutionary engineering, promoter, and protein engineering. However, genetic and metabolic engineering have been mostly used [Citation158]. The goal of metabolic engineering is to systematically investigate – via molecular biology experiments and computational analysis – metabolic and other pathways in order to enhance cellular properties for the synthesis of desired products, which demands to carry out proper genetic alterations (see supplementary Table S3). Engineering the yeast secretory route, by using system-wide metabolic studies, for improved cellulase gene expression is required to ameliorate lignocellulosic breakdown [Citation159]. Recently, emphasis has been placed on the discovery of novel sugar transporters that do not require glucose repression and enhance non-glucose utilization, together with cellulase and xylanase secretion. This can be achieved by improving the native xylose-utilizing microbes, through consolidated bioprocessing (CBP), division of labor, and most importantly re-engineering of the metabolic pathways. Additionally, microbial consortium fermentation has been reported to outperform pure culture fermentation in terms of resistance to environmental perturbations and microbial invasion [Citation160]. Microbial consortia may utilize renewable and complicated feedstocks with greater bioconversion efficiency than pure culture, resulting in lower purifying costs. As such, the creation of microbial consortia for improving the utilization of mixed-sugar or lignocellulosic components is of utmost importance. For LBs, this can be achieved through some precise selection/engineering some model strains [Citation158,Citation161].
6.1. Metabolic engineering strategy
Microorganisms are required in industrial-scale bioconversion of lignocellulosic biomass to sugars and to economically viable products, and metabolic engineering via fermentation pathway modifications has been reported as a way forward. Reconstruction of native metabolic mechanism of the pentose phosphate pathway (PPP) has led to efficient xylose fermentation, it has also led to better assimilation of heterogeneous xylose metabolism, and co-fermentation of mixed sugar derivative of LCBs in S. cerevisiae [Citation4,Citation162,Citation163]. Despite the fact that xylose, a component of hemicellulose, is the second most common sugar in LCB, many microbes can hardly used/mineralize it either for cellular growth or for production of methanol/ethanol (Metanogenesis). Hence, a considerable amount of lignocellulosic hydrolyzates (LHs) is wasted [Citation4]. The effective and quick use of lignocellulosic sugars by fermenting microorganisms is required for the industrial-scale bioconversion to be economically viable [Citation162]. The versatility of S. cerevisiae for conversion of xylulose (which is an isomerized product of xylose) to ethanol has been reported even though S. cerevisiae cannot utilize xylose directly. Engineering and expression of important LEs into synthetic yeast can help the lignocellulose biorefineries. The expression/engineering of xylose reductase enzyme (XR), xylose isomerase enzyme (XI), xylitol dehydrogenase enzyme (XDH) or xylulokinase enzyme (XK) have been done in S. cerevisiae for xylose utilization [Citation164]. Here, the metabolism of phosphorylated xylulose by xylulokinase was channeled into glycolysis via the PPP.
Based on numerous studies on the utilization of extracellular xylose via the xylulose isomer for efficient fermentation, six pathways have been identified and engineered in S. cerevisiae for xylose metabolism; introduction of xylose transporter into the cell, efficient xylose utilization through iterative cycles before selection [Citation165], expression of xylose or xylitol dehydrogenase (XDH) or only the xylose isomerase gene to bring the intracellular xylose and xylulose [Citation166], increasing the xylulose stage to xylulose-5-phosphate via the expression of endogenous xylulokinase gene [Citation167], correction of redox imbalance by introduction of xylose reductase and xylitol dehydrogenase to change either their co-factor specificity or adjusting their NADH-/NADPH-producing steps [Citation97], and lastly, expansion of PPP flux by over-expression of ribulose-5-phospho-3-epimerase enzyme (RPE), ribulose-5-phosphate isomerase enzyme (RPI), transketolase enzyme (TKL), and transaldolase enzyme (TAL) [Citation168,Citation169].
Yeast cannot utilize the Entner – Doudoroff pathway (EMB) pathway for xylose metabolism. In contrast, yeast makes use of PPP that is essential for the release of D-ribose 5-Phosphate and D-erythrose 4-P for the biosynthesis of nucleic acid, aromatic amino acids, and NADPH (the latter necessary for anabolic reactions) [Citation158,Citation170]. Oxidative and non-oxidative phases have been recognized in PPP. The former converts the phosphorylated hexose sugar (glucose 6-P) into pentose sugar (ribulose 5-P), CO2, and NADPH. The latter converts ribulose 5-P into ribose 5-P, xylulose 5-P, glyceraldehyde 3-P, sedoheptulose 7-P, erythrose 4-P, and fructose 5-P. Both xylose and L-arabinose enter the PPP via D-xylulose () [Citation170]. Yeast and bacteria have been engineered for over-expression of D-xylose reductase (XYL1), xylitol dehydrogenase (XYL2), D-xylulokinase (XYL3), xylose isomerase (xylA), L-arabinose isomerase (araA), L-arabinose isomerase (araD) to increase the assimilation of D-xylose and L-arabinose. Phosphoketolase (XFP) is a bypass enzyme for the PPP, which converts inorganic phosphate to xylulose-5-phosphate and produces acetyl phosphate and phosphoenolpyruvate [Citation90]. Acetate kinase (ackA) converts acetyl phosphate to acetate and ATP, whereas alcohol dehydrogenase (ADH1), acetaldehyde dehydrogenase (adhE), and phosphotransacetylase (PTA) convert it to ethanol. Acetaldehyde is produced by pyruvate decarboxylase (PDC1) during normal glycolysis. Aldehyde dehydrogenase (ALD6) converts acetaldehyde to acetate and NADPH [Citation170].
Figure 2. Engineered yeast cells (S. cerevisiae) with enhanced pathways by using systems and synthetic biology principles can act as cell factory platforms for the utilization of LCB in the production of various bio-based chemicals and biofuels. Black arrows depict enzymes involved in lignocellulose degradation; blue arrows represent the pentose phosphate pathway (PPP); green arrows describe the Embden meyerhof pathway (EMP). shows some examples of yeasts that have been engineered for efficient biofuel production.

Table 5. Yeasts that have been genetically engineered for improving biofuel production.
Various genetic and metabolic engineering modification strategies have been used to develop strains that are capable of fermenting xylose to generate ethanol even under unfavorable substrates. For instance, Ma et al. [Citation171] reported the engineering of S. cerevisiae strain NRRLY-50049 that can utilize xylose under industrial inhibitors, the strain was engineered with codon-optimized XK, XDH, XI together with some xylose transporter genes. This ensures its growth in xylose and generation of 38.6 g/L ethanol. In another study, Selim et al. [Citation172] reported a recombinant S. cerevisiae that was engineered with XYL1 and XYL2 from P. stipites. XYL1 has a stronger affinity for NADPH than for NADH, but XYL2 solely utilizes NAD+, resulting in an excess of NADH that cannot be recycled by respiration under oxygen-depleted circumstances. S. cerevisiae generally compensates by generating glycerol, but this is insufficient during the absorption of xylose such that xylitol accumulates [Citation172]. In another development, S. cerevisiae NAPX37 was made from industrial xylose-fermenting S. cerevisiae KF-719 for more adequate xylose fermentation and resistance to a synthetic inhibitor cocktail [Citation173,Citation174].
6.2. Engineering of modular co-culture strategy
The co-culture engineering approach is based on advanced metabolic engineering designed to improve the bioproduction performance of several microbial strains in co-culture for specific biosynthesis [Citation175,Citation176]. The knowledge of microbial metabolic pathways has enabled the biosynthesis of value-added metabolites using microbial biocatalysts. Monoculture is a variation of the co-culture approach and contains a cell population from a unique microbial strain. This technique has been utilized for the efficient exploitation of complex substrates, such as lignocellulose, for the biosynthesis of desired products. Distinct from the usual co-culture studies, modular co-culture engineering is a more sophisticated biosynthetic approach that encourages the adoption of modular and spatial organization for the design, construction, and manipulation of a target biosynthetic pathway or biosynthetic gene clusters (BGCs) for improved microbial performance.
Therefore, modular co-culture engineering, aims to design biosynthetic pathway for targeted bioprocess in a mixed culture of evolved microbial strains with a distinctive approach and modules. This includes the separation of a biosynthetic pathway into serial modules and the usage of designated microbial strains to tackle each module for biosynthesis. Compared to the conventional monoculture approach, Zhang et al. [Citation175] indicates six important advantages of the modular engineering approach, including specific support and efficiency of various pathway modules for the target products, efficient utilization of complex substrates in a co-cultivation of microbial strains, flexibility in the balancing of modular biosynthetic pathways, drastic reduction of undesirable interference of individual pathway modules, diversified cellular environment that encourages unrestricted functional expression of different pathway genes, and most importantly highly efficient bioconversion performance due to reduction in metabolic burden of any individual strain. An interesting overlap between modular co-culture and a consolidated bioprocess for the utilization of LCB was reported by Zuroff et al. [Citation177]. The approach was employed in bioethanol production by co-culture of Clostridium phytofermentans and S. cerevisiae using cellulose substrate. Two modules were employed for this task: a pathway for cellulose hydrolysis in C. phytofermentans and the ethanol production system in S. cerevisiae. Connection between the individual modules were facilitated by cellodextrin transporter intermediate expressed in S. cerevisiae. Similarly, for efficient cellodextrin bioconversion, β-glucosidase was engineered in S. cerevisiae and an improved production of bioethanol (22–100 g/L) was achieved.
6.3. Consolidated bioprocessing (CBP) strategy
Alternative to metabolic and modular engineering strategies, CBP is a more simplified technique through consolidated bioprocessing that focuses on addressing the efficient utilization of cellulose components of LCB [Citation178]. The combined activity of major cellulolytic enzymes like cellulolases is required to be consolidated by some other auxiliary enzymes for cellulose hydrolysis. As a result many S. cerevisiae strains have been designed with range of enzymes for this bioprocess [Citation4,Citation174]. The CBP strategy was designed to hasten, simplify, and reduce biorefining costs for LCBs for instance, efficient breakdown of lignocellulose biomass and sugar fermentation to desired products even without the pretreatment procedures [Citation179]. This process (CBP) requires a microbial host such as S. cerevisiae that can degrade and oxidize lignocellulose biomass and also producing desirable products in high yields and titers. Unfortunately, finding a single natural host capable of doing both jobs has been difficult. To build a host for such requirement, two key methodologies have been developed: (i) Engineering an organism that can consume/degrade the biomass in its complex form to less complex bioavailable form for example, the Aspergillus, Penicillium, Clostridium, or Cellulomonas and, (ii) engineering a fuel or chemical-producing hosts that can use the less complex plant biomaterials as substrate for example, S. cerevisiae and E. coli. [Citation161]. Recombinant production of heterologous cellulase genes in natural ethanologens has been the main technique in the great bulk of research toward generating a CBP [Citation158,Citation180]. Multiple cellulases at least one from each class must be expressed by the host cell to accomplish full cellulose hydrolysis. The budding yeast, S. cerevisiae and E. coli are the most often employed host for heterologous expression of cellulase genes for CBP [Citation82,Citation181,Citation182]. Presently, there are many native and synthetic promoters discovered for expression of cellulase genes, these promoters can be inducible or constitutive, strong or weak, synthetic or natural depending on the design and choice of the gene expression. Popular examples are the constitutive ADH1, constitutive pGPD, galactose-inducible promoter pGAL1 and copper-inducible promoter pCUP1 in S. cerevisiae cell [Citation182].
6.4. Division of labor (DoL)
Metabolic pathways are often altered in isolated microbial communities. The introduction of heterologous circuits into the host, on the other hand, might impose a significant metabolic cost on the system, limiting its total production [Citation183]. This constraint might be solved via metabolic division of labor (DOL), in which various populations undertake different phases in a metabolic process, lowering the load on each population [Citation184]. DOL has been found in a variety of metabolic pathways in nature, and various synthetic systems have demonstrated its viability. Division of Labor (DoL) using natural and synthetic microbial consortia is another option for addressing metabolic burden. DOL has its own limitations, as one or more intermediates may be shared by two or more populations in some instances. However, by limiting the effective quantities of enzymes or substrates, setting constraints in molecular transport across the cell membrane and making a dilution of the intermediate(s) in the extracellular space, the efficiency of metabolic processes can be impaired [Citation183]. In both single-cell and DOL environments, metabolic pathways may frequently be tuned to reduce intermediate losses to meet this issue. Evolution of mutualistic growth, nutritional divergence and crossover feeding, strain inoculation optimization, cell immobilization, and biosensors have all been documented as cell population management tactics to generate stable consortia [Citation184]. The utilization of both xylose and glucose from LCB source provides a cost-effective bioprocess.
Various genes encoding cellulases from fungi and bacteria strains have been successfully expressed yeast, these yeast can secret the enzyme extracellularly into their medium or displayed on the yeast surface as cellulolytic yeast strains [Citation185]. S. cerevisiae, which has a high potential for ethanol synthesis, is one of the most promising CBP candidates. Most importantly, a key aspect of CBP’s cellulosic ethanol production is the development of microbial strains with traits such as efficient cellulose breakdown and ethanol generation. Yanase et al. [Citation185] furthermore constructed an S. cerevisiae strain that was able to co-express three cellulolytic enzymes for ethanol production. These enzymes include endoglucanases (EG), cellobiohydrolases (CBH) both from Trichoderma reesei, and β-glucosidases (BGL) from Aspergillus aculeatus. The cellulosic components were first digested by both EG and CBH, while BGL converted the resulting sugars to glucose. Apart from the successful expression of all the three enzymes in yeast, the strain gave about 3 to 5 folds increment in cellulose degradation. In another development, Baek et al. [Citation186] demonstrated co-expression of two fungal endoglucanases, EGI and EGII from Thermoascus aurantiacus and Trichoderma reesei, respectively, and two bacterial endoglucanases CelA and CelD from Clostridium thermocellum in S. cerevisiae EBY100 strain. These enzymes were engineered under the control of constitutive yeast promoter pGAL. The author rather than using single yeast cell for the expression of these enzymes, they use different yeast cell expressing each enzyme and they were all employed for ethanol production. Chen et al. [Citation187] as well as Xiao et al. [Citation188] also reported the synergistic action of cellulase and xylanase-producing yeast for degradation of LCBs such as Pasc, Birchwood and maize stover.
In developing a potential strain for simultaneous utilization of hemicellulolytic biomass, there is a need for several genes encoding xylanase to be inserted in the host organism [Citation158]. However, the insertion of multiple genes into a single yeast host has been challenging due to the genetic instability of the engineered cells [Citation158]. Lee et al. [Citation180] devised a one-by-one yeast system to address some of these challenges, particularly in the synergistic hydrolysis of cellulase enzyme system. The effectiveness of combining four cellulosic strains of S. cerevisiae was shown to be effective, the strains are expressing important cellulases such as endoglucanase (TrEGL2), cellobiohydrolase (CtCBH1), cellobiohydrolase (ClCBH2), and β-glucosidase (SfBGL1) obtained from T. reesei, Chaetomium thermophilum, Chrysosporium lucknowense, and Saccharomycopsis fibuligera, respectively.
7. Applications of engineered yeast in lignocellulosic biorefineries, limitation, and potentials of metabolic engineering in yeast cells
Many yeast strains have been developed for the generation of biofuel. The S. cerevisiae is most commonly used for LB processes at industrial-scale production of biochemicals and biofuels [Citation189]. It is the most widely used at the industrial scale for converting sugar to ethanol, due to its ability to withstand low pH values, ferment under anaerobic conditions, tolerance to high osmotic pressures, and low propensity to bacteriophage infections [Citation190]. However, yeast exhibits a weak expression of the pentose pathway and has poor/no xylose uptake ability. Mussatto et al. [Citation191] reported that only some yeasts from genera Candida, Pichia, Pachysolen, and Schizosaccharomyces have the capacity to ferment pentoses into ethanol. To overcome this limitation, a technique that incorporates co-fermentation of two yeast strains can be used to produce a good amount of bioethanol, thus, allowing for increased use of LCB in biorefineries. Hybrid strains of S. cerevisiae have been developed by employing the fusion of the protoplasts of S. cerevisiae and other xylose-fermenting yeasts like P. tannophilus, C. shehatae, and P. stipites [Citation192]. Genetic engineering has helped to develop synthetic yeasts that can produce bioethanol from xylose at high yields (). This has been achieved through the use of recombinant DNA technology for up-regulation of the stress tolerance genes that can overcome inhibitory situations [Citation219].
The use of S. cerevisiae in the production of bioethanol has been expanded using genetic engineering, recombinant yeasts have been made, proven, and characterized for industrial feasibility [Citation163,Citation220]. Furthermore, there have been success on drawbacks with the use of yeast using engineered yeasts like many inhibitory situations associated with the utilization of LCB for the industrial production of bioethanol, such yeasts are increasingly been developed and adopted in recent years. In a study by Tanimura et al. [Citation220], the ability of S. cerevisiae to ferment xylose was developed through the introduction of xylose reductase and xylitol dehydrogenase genes from S. stipites. The engineered yeast showed the ability to convert cellulose to ethanol more rapidly in comparison to unmodified yeast strains [Citation163,Citation176,Citation219–221]. For instance, in yeasts, xylose metabolism cannot happen simultaneously together with glucose fermentation due to glucose inhibition of xylose metabolic pathway (). However, this problem has been avoided with the use of transporter engineering to import xylose into cells via heterologous PPP or via the over-expression of switchable homologous pentose-hexose transporter mechanism [Citation222].
Ko et al. [Citation223] reported adequate conversion of various LCBs into bioethanol by creating a yeast strain XUSE capable of inhibition of acetic-acid stress with adequate xylose utilization. They also made more strains from XUSE by adaptive laboratory evolution, for example, the XUSAE57. The development of engineered yeasts, such as XUSAE57, with the ability to convert LCB into bioethanol has great potential for increased usage of this biomass in LB for the production of bioethanol and other bioproducts. The strain XUSAE57 has the ability to efficiently convert xylose into ethanol with high yields. It was discovered that the XUSAE57 strain not only achieved two-fold higher ethanol yields but also improved the xylose utilization rate by more than two-fold compared to that of XUSE in the presence of 4 g/L of acetic acid [Citation224]. Similarly, during the fermentation of LHs, XUSAE57 was able to simultaneously convert glucose and xylose while recording the highest ethanol yield reported to date (0.49 g ethanol/g sugars). This shows that the use of LHs also increase industrial ethanol bio-production; however, the fermentation temperature and reinforcement of the xylose catabolism pathway are also important parameters to consider for efficient production in any LB. By incorporating engineered yeast such as XUSEA, it is expected that there would be increased bioethanol production in LB alongside alterations in the fermentation temperature and xylose catabolism pathway as shown in the study conducted by Tran et al. [Citation225]. In another study, Tran et al. [Citation225] developed a mixed-sugar co-fermenting strain of S. cerevisiae, XUSEA, to expand the LB product profile. This strain has the capacity to convert xylose during simultaneous glucose/xylose co-fermentation, it can withstand varying fermentation temperature, can co-ferment plant biomass with LHs and requires less process time as compared to XUSE.
8. Conclusion
Application of microbes in industrial biotechnology process is a key factor shaping biorefinery and bioeconomy. However, challenges remain with adequate strain characterization, growth-culture dynamics, and toxicity issues especially with metabolites generated from biomass utilization. A particular example is ‘methylotrophy/methalotrophy,’ which is the utilization of reduced single carbon (C1) substrate (like methylamine and methanol) as sole carbon and energy source. The production of formaldehyde, a single carbon toxic hydrocarbon during methy/methalotrophy growth/transition has been a major problem. This problem can be easily bypassed with engineered yeast strains. Here, the metabolic oxidation of C1 and regeneration of pentose like in XuMp metabolism which is the main constraint in bacteria, can be enhanced using genetic engineering tools.
Nevertheless, biorefinery requires choosing of suitable microbes for specific bioprocess while the basic mechanisms underlining their metabolic actions should be well understood and predictable. Native microbes are not as efficient as synthetic organisms for some important fermentation process. Many synthetic yeasts have been designed and are claimed to show the desired biomass utilization, and production capacity with desired time, quality, and quantity. However, many of such yeasts have not been utilized industrially for mass production. This review has highlighted the importance and several successes recorded in laboratory-scale experiments. It established that laboratory successes do not automatically translate to success in their application at industrial scales, and advocates that the science community still need more convincing facts when it comes to the release of engineered strains either into the environment or applying them in real time outside the laboratory scale.
As highlighted in this review article, the basic research question on the use of engineered yeasts should be on their metabolic requirements, their performance under different carbon and energy sources, and their response to different cultural conditions. It is important to determine which synthetic design would be the best for particular bioprocesses and how efficiently they can process carbon substrates to products. On the question of which synthetic design to use, the advancement in molecular and synthetic biology tools has made it simple or rather easy to achieve many things. The utilization of peroxisomal signal peptides, and genetic engineering tools like the CRISPR-Cas (Clustered Regularly Interspaced Short Palindromic Repeats and CRISPR-associated proteins) have helped considerably. However, the growth of engineered yeasts as pointed out in this article, can be improved by using different carbon and nitrogen sources for solid state fermentation of LCBs. In addition, the use of in silico metabolic modeling, 13C-metabolic flux analysis, and some systems biotechnology pipeline tools have reduced this constraint. The goal of methylotrophy although still need more extensive research but holds the greatly innovative use of C1 feedstock for the enhancement of diversified process. Taken together, we conclude that the adoption of engineered yeasts in biorefinery would establish a twin benefit of a sustainable global economy and a reduced climate change associated with the nonrenewable fossil resources.
List of Abbreviations
AA | = | Auxiliary activity |
AA | = | Auxiliary activity; |
AAO | = | Aryl-alcohol oxidase |
AAO | = | Arylalcohol oxidases/dehydrogenases |
Ack | = | Acetate kinase |
Adh | = | Acetaldehyde dehydrogenase |
ADH | = | Alcohol dehydrogenase |
ALD | = | Aldehyde dehydrogenase |
AOx | = | Alcohol oxidase |
BGCs | = | Biosynthetic gene clusters |
BGL | = | β-glucosidases |
BRF | = | Brown rot fungi |
CBH | = | Cellobiohydrolases |
CBP | = | Consolidated bioprocessing |
CMC | = | Carboxymethyl cellulose |
CRISPR-Cas | = | Clustered Regularly Interspaced Short Palindromic Repeats and CRISPR-associated |
DoL | = | Division of labor |
DyPs | = | Dye decolorizing peroxidases |
XYL1 | = | D-xylose reductase |
EG | = | Endoglucanases |
EMB | = | Entner–Doudoroff pathway |
EMP | = | Emden Meyerhof Pathway |
GAO | = | Galactose oxidase |
GLOX | = | Glyoxal oxidase |
GOX | = | Glucose oxidase |
HTPs | = | Heme-thiolate peroxidases |
Lac | = | Laccase |
LB | = | Lignocellulose biorefineries |
LCBs | = | Lignocellulosic biomass/biomaterials |
LE | = | Ligninolytic enzymes |
LHs | = | Lignocellulosic hydrolysates |
LiP | = | Lignin peroxidase |
LMEs | = | Lignin modifying enzymes |
MnP | = | Manganese peroxidase |
MOX | = | Methanol oxidase |
PASC | = | Phosphoric acid swollen cellulose |
PDC | = | Pyruvate decarboxylase |
PDH | = | Pyranose dehydrogenase/oxidase |
PTA | = | Phosphotransacetylase |
PPP | = | Pentose phosphate pathway |
RPE | = | Ribulose-5-phosphate 3-epimerase |
RPI | = | Ribulose-5-phosphate isomerase |
TAL | = | Transaldolase |
TFP | = | Translational fusion partner |
TKL | = | Transketolase |
VAO | = | Vanillyl-alcohol oxidase |
VP | = | Versatile peroxidase |
WRF | = | White rot fungi |
XDH | = | Xylitol dehydrogenase |
XI | = | Xylose isomerase |
XK | = | Xylulokinase |
XFP | = | Phosphoketolase |
XR | = | Xylose reductase |
XYL | = | Xylitol dehydrogenase |
Author Contribution Statement
Michael D. Asemoloye: Conceptualization, Validation, visualization, Methodology, Formal analysis, Project administration, Writing – original draft, Investigation, Writing – review & editing. Mario Andrea Marchisio and Wolfgang Wanek: Conceptualization, Funding acquisition, Supervision, Project administration, Resources, Instrumental software, Validation, Writing – review & editing. Tunde Sheriffdeen Bello, Peter Olusakin Oladoye, Muideen Gbadamosi, Segun Oladiran Babarinde, Gboyega Adebami, Olumayowa Mary Olowe, and Marta Elisabetta Eleonora Temporiti: Writing – review & editing, Validation, Visualization, Methodology, Formal analysis.
Supplemental Material
Download MS Word (31.6 KB)Acknowledgments
Dr. Asemoloye thanks the OeAD – Austria’s Agency for Education and Internationalization, Mobility Programmes, Bilateral and Multilateral Cooperation and Federal Ministry of Education, Science and Research (BMBWF), Austria for having financed a period of his research in the Department of Microbiology and Ecosystem Science, Division of Terrestrial Ecosystem Research University of Vienna through the Ernst Mach Grant, Eurasia-Pacific Uninet. MPC-2021-00421
Disclosure statement
No potential conflict of interest was reported by the author(s).
Data availability statement
The authors confirm that no new data were created or analyzed in this study Data sharing is not applicable to this article.
Supplementary material
Supplemental data for this article can be accessed online at https://doi.org/10.1080/21655979.2023.2269328
Additional information
Funding
References
- Amponsah NY, Troldborg M, Kington B, et al. Greenhouse gas emissions from renewable energy sources: a review of lifecycle considerations. Renew Sust Energ Rev. 2014;39:461–31. doi: 10.1016/j.rser.2014.07.087
- Karan H, Funk C, Grabert M, et al. Green bioplastics as part of a circular bioeconomy. Trends Plant Sci. 2019;24(3):237–249. doi: 10.1016/j.tplants.2018.11.010
- Martinez-Hernandez E, Campbell G, Sadhukhan J. Economic value and environmental impact (EVEI) analysis of biorefinery systems. Chem Eng Res Des. 2013;91(8):1418–1426. doi: 10.1016/j.cherd.2013.02.025
- Liu G, Qu Y. Integrated engineering of enzymes and microorganisms for improving the efficiency of industrial lignocellulose deconstruction. Eng Microbiol. 2021;1:100005. doi: 10.1016/j.engmic.2021.100005
- Santos VEN, Magrini A. Biorefining and industrial symbiosis: a proposal for regional development in Brazil. J Clean Prod. 2018;177:19–33. doi: 10.1016/j.jclepro.2017.12.107
- Amin L, Hashim H, Mahadi Z, et al. Attitudes towards biodiesel. Biotechnol Biofuels. 2017;10(1):219. doi: 10.1186/s13068-017-0908-8
- Moore RH, Thornhill KL, Weinzierl B, et al. Biofuel blending reduces particle emissions from aircraft engines at cruise conditions. Nature. 2017;543(7645):411–415. doi: 10.1038/nature21420
- Vendamme R, Behaghel de Bueren J, Gracia-Vitoria J, et al. Aldehyde-assisted lignocellulose fractionation provides unique lignin oligomers for the design of tunable polyurethane bioresins. Biomacromolecules. 2020;21(10):4135–4148. doi: 10.1021/acs.biomac.0c00927
- Raj T, Chandrasekhar K, Naresh Kumar A, et al. Recent advances in commercial biorefineries for lignocellulosic ethanol production: current status, challenges and future perspectives. Bioresour Technol. 2022;344:126292. doi: 10.1016/j.biortech.2021.126292
- Silveira MHL, Morais ARC, da Costa Lopes AM, et al. Current pretreatment technologies for the development of cellulosic ethanol and biorefineries. ChemSuschem. 2015;8(20):3366 3390. doi: 10.1002/cssc.201500282
- Kumar K, Correia MAS, Pires VMR, et al. Novel insights into the degradation of β-1,3-glucans by the cellulosome of Clostridium thermocellum revealed by structure and function studies of a family 81 glycoside hydrolase. Int j biol macromol. 2018;117:890–901. doi: 10.1016/j.ijbiomac.2018.06.003
- Smit AT, van Zomeren A, Dussan K, et al. Biomass pre-extraction as a versatile strategy to improve biorefinery feedstock flexibility, sugar yields, and lignin purity.ACS sustain. 2022. ACS Sustainable Chem Eng. 2022;10(18):6012–6022. doi: 10.1021/acssuschemeng.2c00838
- Asemoloye MD, Ahmad R, Jonathan SG. Transcriptomic responses of catalase, peroxidase and laccase encoding genes and enzymatic activities of oil spill inhabiting rhizospheric fungal strains. Environ Pollut. 2018;235:55–64. doi: 10.1016/j.envpol.2017.12.042
- Brandt BA, García-Aparicio MDP, Görgens JF, et al. Rational engineering of Saccharomyces cerevisiae towards improved tolerance to multiple inhibitors in lignocellulose fermentations. Biotechnol Biofuels. 2021;14(1):173. doi: 10.1186/s13068-021-02021-w
- Zhang M-M, Xiong L, Tang Y-J, et al. Enhanced acetic acid stress tolerance and ethanol production in Saccharomyces cerevisiae by modulating expression of the de novo purine biosynthesis genes. Biotechnol Biofuels. 2019;12(1):116. doi: 10.1186/s13068-019-1456-1
- Ko JK, Lee JH, Jung JH, et al. Recent advances and future directions in plant and yeast engineering to improve lignocellulosic biofuel production. Renew Sust Energ Rev. 2020;134:110390. doi: 10.1016/j.rser.2020.110390
- Majidian P, Tabatabaei M, Zeinolabedini M, et al. Metabolic engineering of microorganisms for biofuel production. Renew Sust Energ Rev. 2018;82:3863–3885. doi: 10.1016/j.rser.2017.10.085
- Spagnuolo M, Yaguchi A, Blenner M. Oleaginous yeast for biofuel and oleochemical production. Curr Opin Biotechnol. 2019;57:73–81. doi: 10.1016/j.copbio.2019.02.011
- Asemoloye MD, Marchisio MA. Synthetic Saccharomyces cerevisiae tolerate and degrade highly pollutant complex hydrocarbon mixture. Ecotoxicol Environ Saf. 2022;241:113768. doi: 10.1016/j.ecoenv.2022.113768
- Rodrigues RC, Sene L, Matos GS, et al. Enhanced xylitol production by precultivation of Candida guilliermondii cells in sugarcane bagasse hemicellulosic hydrolysate. Curr Microbiol. 2006;53(1):53–59. doi: 10.1007/s00284-005-0242-4
- Revuelta JL, Ledesma-Amaro R, Lozano-Martinez D, et al. Bioproduction of riboflavin: a bright yellow history. J Ind Microbiol Biotechnol. 2017;44(4–5):659–665. doi: 10.1007/s10295-016-1842-7
- Niehus X, Crutz-Le Coq AM, Sandoval G, et al. Engineering yarrowia lipolytica to enhance lipid production from lignocellulosic materials. Biotechnol Biofuels. 2018;11(1):11. doi: 10.1186/s13068-018-1010-6
- Patinvoh RJ, Osadolor OA, Chandolias K, et al. Innovative pretreatment strategies for biogas production. Biores Technol. 2017;224:13–24. doi: 10.1016/j.biortech.2016.11.083
- Zheng Y, Zhao J, Xu F, et al. Pretreatment of LCB for enhanced biogas production. Prog Energ Combust Sci. 2014;42(1):35–53. doi: 10.1016/j.pecs.2014.01.001
- Titiladunayo IF, McDonald AG, Fapetu OP. Effect of temperature on biochar product yield from selected lignocellulosic biomass in a pyrolysis process. Waste Biomass Valorization. 2012;3(3):311–318. doi: 10.1007/s12649-012-9118-6
- Huang J, Fu S, Gan L. Lignin Chemistry and applications. Elsevier; 2019.
- Rabemanolontsoa H, Ayada S, Saka S. Quantitative method applicable for various biomass species to determine their chemical composition. Biomass Bioenergy. 2011;35(11):4630–4635. doi: 10.1016/j.biombioe.2011.09.014
- Komolwanich T, Tatijarern P, Prasertwasu S, et al. Comparative potentiality of Kans grass (saccharumspontaneum) and giant reed (arundodonax) as lignocellulosic feedstocks for the release of monomeric sugars by microwave/chemical pretreatment. Cellul. 2014;21(3):1327–1340. doi: 10.1007/s10570-013-0161-7
- Scordia D, Cosentino SL, Jeffries TW. Second generation bioethanol production from Saccharum spontaneum L. ssp. Aegyptiacum (Willd.) hack. Biores Technol. 2010;101(14):5358–5365. doi: 10.1016/j.biortech.2010.02.036
- Zhao X, Qi F, Liu D. Nanotechnology production and biofuel for Bioenergy. M. Rai, S. S. D S Eds. Springer; 2017. 10.1007/978-3-319-45459-7_13
- Dhyani V, Bhaskar T. Chapter 9—pyrolysis of biomass. In: Pandey A, Larroche C, Dussap C-G, Gnansounou E, Khanal SK Ricke S, editors. Biofuels: alternative feedstocks and conversion processes for the production of liquid and gaseous biofuels. Second Edition) ed. Vol. 2019. Academic Press. pp. 217–244. 10.1016/B978-0-12-816856-1.00009-9
- Somerville C. Cellulose synthesis in higher plants. Annu Rev Cell Dev Biol. 2006;22(1):53–78. doi: 10.1146/annurev.cellbio.22.022206.160206
- Davison BH, Parks J, Davis MF, et al. Plant cell walls: basics of structure, Chemistry, accessibility and the Influence on conversion. In: Wyman CE, editor. Aqueous pretreatment of plant biomass for biological and chemical conversion to fuels and chemicals. John Wiley & Sons, Ltd; 2013. pp. 23–38. DOI:10.1002/9780470975831.ch3.
- Li C, Zhao X, Wang A, et al. Catalytic Transformation of lignin for the production of chemicals and fuels. Chem Rev. 2015;115(21):11559–11624. doi: 10.1021/acs.chemrev.5b00155
- Sharma HK, Xu C, Qin W. Biological pretreatment of LCB for Biofuels and bioproducts: an overview. Waste Biomass Valorization. 2019;10(2):235–251. doi: 10.1007/s12649-017-0059-y
- Klemm D, Heublein B, Fink HP, et al. Cellulose: fascinating biopolymer and sustainable raw material. Angew Chem Int Ed. 2005;44(22):3358–3393. doi: 10.1002/anie.200460587
- Ali N, Zhang Q, Liu ZY, et al. Emerging technologies for the pretreatment of lignocellulosic materials for bio-based products. Appl Microbiol Biotechnol. 2020;104(2):455–473. doi: 10.1007/s00253-019-10158-w
- Hassan SS, Williams GA, Jaiswal AK. Lignocellulosic biorefineries in Europe: Current state and prospects. Trends Biotechnol. 2019;37(3):231–234. doi: 10.1016/j.tibtech.2018.07.002
- Wang F, Ouyang D, Zhou Z, et al. LCB as sustainable feedstock and materials for power generation and energy storage. J Energy Chem. 2021;57:247–280. doi: 10.1016/j.jechem.2020.08.060
- Isikgora FH, Becer CR. LCB: a sustainable platform for production of bio-based chemicals and polymers. Polym Chem. 2010;1(1):13. doi: 10.1039/c000660m
- Rowell R, Pettersen R, Tshabalala M. Handbook of wood Chemistry and wood composites. Handbook Of Wood Chemistry And Wood Composites, Second Edition. 2012. doi: 10.1201/b12487-5
- Zakzeski J, Bruijnincx PCA, Jongerius AL, et al. The catalytic valorization of lignin for the production of renewable chemicals. Chem Rev. 2010;110(6):3552–3599. doi: 10.1021/cr900354u
- Mielenz JR. Ethanol production from biomass: technology and commercialization status. Curr Opin Microbiol. 2001;4(3):324–329. doi: 10.1016/S1369-5274(00)00211-3
- Banu J, Preethi Kavitha S, Tyagi VK, et al. LCB based biorefinery: a successful platform towards circular bioeconomy. Fuel. 2021;302(May):121086. doi: 10.1016/j.fuel.2021.121086
- Chandra RP, Bura R, Mabee WE, et al. Substrate pretreatment: the key to effective enzymatic hydrolysis of lignocellulosics? Adv Biochem Eng Biotechnol. 2007;108:67–93.
- Reddy N, Yang Y. Biofibers from agricultural byproducts for industrial applications. Trends Biotechnol. 2005;23(1):22 27. doi: 10.1016/j.tibtech.2004.11.002
- Saini JK, Saini R, Tewari L. Lignocellulosic agriculture wastes as biomass feedstocks for second-generation bioethanol production: concepts and recent developments. 3 Biotech. 2015;5(4):337 353. doi: 10.1007/s13205-014-0246-5
- Buranov AU, Mazza G. Lignin in straw of herbaceous crops. Ind Crops Prod. 2008b;28(3):237–259. doi: 10.1016/j.indcrop.2008.03.008
- Yuan Z, Long J, Wang T, et al. Process intensification effect of ball milling on the hydrothermal pretreatment for corn straw enzymolysis. Energy Convers Manag. 2015;101:481–488. doi: 10.1016/j.enconman.2015.05.057
- Satpathy SK, Tabil LG, Meda V, et al. Torrefaction of wheat and barley straw after microwave heating. Fuel. 2014;124:269–278. doi: 10.1016/j.fuel.2014.01.102
- Merali Z, Marjamaa K, Käsper A, et al. Chemical characterization of hydrothermally pretreated and enzyme-digested wheat straw: an evaluation of recalcitrance. Food Chem. 2016;198:132–140. doi: 10.1016/j.foodchem.2015.07.108
- Cheng L, Adhikari S, Wang Z, et al. Characterization of bamboo species at different ages and bio-oil production. J Anal Appl Pyrolysis 2015b. 2015;116:215–222. doi: https://doi.org/10.1016/j.jaap.2015.09.008
- Buranov AU, Mazza G. Lignin in straw of herbaceous crops. Ind Crops Prod. 2008a;28(3):237–259. doi: 10.1016/j.indcrop.2008.03.008
- Si S, Chen Y, Fan C, et al. Lignin extraction distinctively enhances biomass enzymatic saccharification in hemicelluloses-rich miscanthus species under various alkali and acid pretreatments. Biores Technol. 2015;183:248–254. doi: 10.1016/j.biortech.2015.02.031
- Cheng L, Adhikari S, Wang Z, et al. Characterization of bamboo species at different ages and bio-oil production. J Anal Appl Pyrolysis 2015a. 2015;116:215–222. doi: https://doi.org/10.1016/j.jaap.2015.09.008
- Bledzki AK, Mamun AA, Volk J. Physical, chemical and surface properties of wheat husk, rye husk and soft wood and their polypropylene composites. Compos Part A Appl Sci Manuf. 2010;41(4):480–488. doi: 10.1016/j.compositesa.2009.12.004
- Nanda S, Reddy SN, Vo DSB, et al. Catalytic gasification of wheat straw in hot compressed (subcritical and supercritical) water for hydrogen production. Energy Science & Engineering. 2018;6(5):448–459. doi: 10.1002/ese3.219
- Muh E, Tabet F, Amara S. Biomass conversion to fuels and value-added chemicals: a comprehensive review of the thermochemical processes. Curr Altern Energy. 2021;4(1):3–25. doi: 10.2174/2405463103666191022121648
- Dahmen N, Abeln J, Eberhard M, et al. The bioliq process for producing synthetic transportation fuels. WIREs Energy Environ. 2017;6(3):e236. doi: 10.1002/wene.236
- Antonetti C, Gori S, Licursi D, et al. One-Pot Alcoholysis of the lignocellulosic eucalyptus nitens biomass to n-butyl levulinate, a valuable additive for diesel motor fuel. Catalysts. 2020;10(5):509. doi: 10.3390/catal10050509
- Adeleye AT, Odoh CK, Enudi OC, et al. Sustainable synthesis and applications of polyhydroxyalkanoates (PHAs) from biomass. Process Biochem. 2020;96:174–193. doi: 10.1016/j.procbio.2020.05.032
- Jae J, Tompsett GA, Lin YC, et al. Depolymerization of lignocellulosic biomass to fuel precursors: maximizing carbon efficiency by combining hydrolysis with pyrolysis. Energy Environ Sci. 2010;3(3):358–365. doi: 10.1039/B924621P
- Carrillo-Nieves D, Ruiz HA, Aguilar CN, et al. Process alternatives for bioethanol production from mango stem bark residues. Biores Technol. 2017;239:430–436. doi: 10.1016/j.biortech.2017.04.131
- Siebenhaller S, Kirchhoff J, Kirschhöfer F, et al. Integrated process for the enzymatic production of fatty acid sugar esters completely based on lignocellulosic substrates. Front Chem. 2018;6. doi: 10.3389/fchem.2018.00421. https://www.frontiersin.org/article/10.3389/fchem.2018.00421
- Øverland M, Skrede A. Yeast derived from lignocellulosic biomass as a sustainable feed resource for use in aquaculture: yeast from lignocellulosic biomass as a feed in aquaculture. J Sci Food Agric. 2017;97(3):733–742. doi: 10.1002/jsfa.8007
- Reshmy R, Philip E, Madhavan A, et al. Lignocellulose in future biorefineries: strategies for cost-effective production of biomaterials and bioenergy. Biores Technol. 2022;344:126241. doi: 10.1016/j.biortech.2021.126241
- Feser J, Gupta A. Performance and emissions of drop-in aviation biofuels in a lab-scale gas turbine combustor. J Energy Resour Technol. 2021;143(4). doi: 10.1115/1.4048243
- Zhou Z, Liu D, Zhao X. Conversion of lignocellulose to biofuels and chemicals via sugar platform: an updated review on chemistry and mechanisms of acid hydrolysis of lignocellulose. Renew Sust Energ Rev. 2021;146:111169. doi: 10.1016/j.rser.2021.111169
- Ray MJ, Leak DJ, Spanu PD, et al. Brown rot fungal early stage decay mechanism as a biological pretreatment for softwood biomass in biofuel production. Biomass Bioenergy. 2010;34(8):1257–1262. doi: 10.1016/j.biombioe.2010.03.015
- Singh T, Vaidya AA, Donaldson LA, et al. Improvement in the enzymatic hydrolysis of biofuel substrate by a combined thermochemical and fungal pretreatment. Wood Sci Technol. 2016;50(5):1003–1014. doi: 10.1007/s00226-016-0838-9
- Huang J, Khan MT, Perecin D, et al. Sugarcane for bioethanol production: potential of bagasse in Chinese perspective. Renew Sust Energ Rev. 2020;133:110296. doi: 10.1016/j.rser.2020.110296
- Food and Agriculture Organization of the United Nations. FAOSTAT statistical database. 2020. Retrieved 2022 May 17 https://www.fao.org/faostat/en/#data/QCL.
- OECD/Food and Agriculture Organization of the United Nations. OECD-FAO agricultural outlook. Paris, France: OECD Publishing; 2015
- Teoh YH, Yu KH, How HG, et al. Experimental investigation of performance, emission and combustion characteristics of a common-rail diesel engine fuelled with bioethanol as a fuel additive in coconut oil biodiesel blends. Energies. 2019;12(10):1954. doi: 10.3390/en12101954
- Shekofteh M, Gundoshmian TM, Jahanbakhshi A, et al. Performance and emission characteristics of a diesel engine fueled with functionalized multi-wall carbon nanotubes (MWCNTs-OH) and diesel–biodiesel–bioethanol blends. Energy Rep. 2020;6:1438–1447. doi: 10.1016/j.egyr.2020.05.025
- Niphadkar S, Bagade P, Ahmed S. Bioethanol production: insight into past, present and future perspectives. Biofuels. 2018;9(2):229–238. doi: 10.1080/17597269.2017.1334338
- Tse TJ, Wiens DJ, Reaney MJT. Production of Bioethanol—A Review of Factors Affecting Ethanol Yield. Fermentation. 2021;7(4):268. doi: 10.3390/fermentation7040268
- Sydney EB, Letti LAJ, Karp SG, et al. Current analysis and future perspective of reduction in worldwide greenhouse gases emissions by using first and second generation bioethanol in the transportation sector. Bioresour Technol Rep. 2019;7:100234. doi: 10.1016/j.biteb.2019.100234
- Boluk G, Koc AA. The implications of biofuel policy in Turkey. Int J Energy Econ Policy. 2013;3(4S):14–22.
- Xia Q, Chen C, Yao Y, et al. A strong, biodegradable and recyclable lignocellulosic bioplastic. Nat Sustain. 2021;4(7):627–635. doi: https://doi.org/10.1038/s41893-021-00702-w
- Bhatia SK, Gurav R, Choi TR, et al. Bioconversion of plant biomass hydrolysate into bioplastic (polyhydroxyalkanoates) using ralstonia eutropha 5119. Biores Technol. 2019;271:306–315. doi: 10.1016/j.biortech.2018.09.122
- Mostafa NA, Farag AA, Abo-Dief HM, et al. Production of biodegradable plastic from agricultural wastes. Arabian J Chem. 2018;11(4):546–553. doi: 10.1016/j.arabjc.2015.04.008
- Fitzpatrick J, Kricka W, James TC, et al. Expression of three Trichoderma reesei cellulase genes in Saccharomyces pastorianus for the development of a two-step process of hydrolysis and fermentation of cellulose. J Appl Microbiol. 2014;117(1):96–108. doi: https://doi.org/10.1111/jam.12494
- Su J, Qiu M, Shen F, et al. Efficient hydrolysis of cellulose to glucose in water by agricultural residue-derived solid acid catalyst. Cellul. 2018;25(1):17–22. doi: 10.1007/s10570-017-1603-4
- Xu Y, Wang P, Xue S, et al. Green biorefinery—the ultra-high hydrolysis rate and behavior of populus tomentosa hemicellulose autohydrolysis under moderate subcritical water conditions. RSC Adv. 2020;10(32):18908–18917. doi: 10.1039/D0RA02350G
- Soongprasit K, Sricharoenchaikul V, Atong D. Phenol-derived products from fast pyrolysis of organosolv lignin. Energy Rep. 2020;6:151–167. doi: 10.1016/j.egyr.2020.08.040
- Ji XJ, Huang H, Nie ZK, et al. Fuels and chemicals from hemicellulose sugars. Adv Biochem Engin/Biotechnol. 2012;128:199–224.
- Varanasi P, Singh P, Auer M, et al. Survey of renewable chemicals produced from LCB during ionic liquid pretreatment. Biotechnol Biofuels. 2013;6(1):14. doi: 10.1186/1754-6834-6-14
- Mamaye M, Kiflie Z, Feleke S, et al. Valorization of Ethiopian sugarcane bagasse to assess its suitability for pulp and paper production. Sugar Tech. 2019;21(6):995–1002. doi: 10.1007/s12355-019-00724-x
- Singh RS, Singh T, Pandey A. Chapter 1—microbial enzymes—an overview. In: Singh RS, Singhania RR, Pandey A Larroche C, editors. Advances in enzyme technology. Elsevier; 2019. pp. 1–40. DOI:10.1016/B978-0-444-64114-4.00001-7.
- Mongkhonsiri G, Gani R, Malakul P, et al. Integration of the biorefinery concept for the development of sustainable processes for pulp and paper industry. Comput Chem Eng. 2018;119:70–84. doi: 10.1016/j.compchemeng.2018.07.019
- Abid K, Jabri J, Yaich H, et al. In vitro study on the effects of exogenic fibrolytic enzymes produced from Trichoderma longibrachiatum on ruminal degradation of olive mill waste. Arch Anim Breed. 2022;65(1):79–88. doi: https://doi.org/10.5194/aab-65-79-2022
- Jabri J, Ammar H, Abid K, et al. Effect of Exogenous fibrolytic enzymes Supplementation or functional feed additives on in vitro ruminal fermentation of chemically pre-treated sunflower heads. Agriculture. 2022;12(5):696. doi: 10.3390/agriculture12050696
- Singh A, Bajar S, Devi A, et al. An overview on the recent developments in fungal cellulase production and their industrial applications. Bioresour Technol Rep. 2021;14:100652. doi: https://doi.org/10.1016/j.biteb.2021.100652
- Singh P, Sulaiman O, Hashim R, et al. Using biomass residues from oil palm industry as a raw material for pulp and paper industry: potential benefits and threat to the environment. Environ Develop Sustainab. 2012;15(2):1–17. doi: 10.1007/s10668-012-9390-4
- Yang H, Xie Y, Zheng X, et al. Comparative study of lignin characteristics from wheat straw obtained by soda-AQ and kraft pretreatment and effect on the following enzymatic hydrolysis process. Biores Technol. 2016;207:361–369. doi: 10.1016/j.biortech.2016.01.123
- O’Dea RM, Pranda PA, Luo Y, et al. Ambient-pressure lignin valorization to high-performance polymers by intensified reductive catalytic deconstruction. Sci Adv. 2022;8(3):eabj7523. doi: 10.1126/sciadv.abj7523
- Zhu Y, Zhang J, Zhu L, et al. Minimize the xylitol production in Saccharomyces cerevisiae by balancing the xylose redox metabolic pathway. Front Bioeng Biotechnol. 2021;9. doi: 10.3389/fbioe.2021.639595. https://www.frontiersin.org/article/10.3389/fbioe.2021.639595
- Gabhane J, Kumar S, Sarma AK. Effect of glycerol thermal and hydrothermal pretreatments on lignin degradation and enzymatic hydrolysis in paddy straw. Renewable Energy. 2020;154:1304–1313. doi: 10.1016/j.renene.2020.03.035
- Isikgor FH, Becer CR. LCB: a sustainable platform for the production of bio-based chemicals and polymers. Polym Chem. 2015;6(25):4497–4559. doi: 10.1039/C5PY00263J
- Cebreiros F, Risso F, Cagno M, et al. Enhanced production of butanol and xylosaccharides from eucalyptus grandis wood using steam explosion in a semi-continuous pre-pilot reactor. Fuel. 2021;290:119818. doi: 10.1016/j.fuel.2020.119818
- Zabihi S, Sharafi A, Motamedi H, et al. Environmentally friendly acetic acid/steam explosion/supercritical carbon dioxide system for the pre-treatment of wheat straw. Environ Sci Pollut Res. 2021;28(28):37867–37881. doi: 10.1007/s11356-021-13410-x
- Martínez-Patiño JC, Ruiz E, Romero I, et al. Combined acid/alkaline-peroxide pretreatment of olive tree biomass for bioethanol production. Biores Technol. 2017;239:326–335. doi: 10.1016/j.biortech.2017.04.102
- Noonari AA, Mahar RB, Sahito AR, et al. Effects of isolated fungal pretreatment on bio-methane production through the co-digestion of rice straw and buffalo dung. Energy. 2020;206:118107. doi: 10.1016/j.energy.2020.118107
- Houfani AA, Anders N, Spiess AC, et al. Insights from enzymatic degradation of cellulose and hemicellulose to fermentable sugars– a review. Biomass Bioenergy. 2020;134:105481. doi: 10.1016/j.biombioe.2020.105481
- Gao W, Li Z, Liu T, et al. Production of high-concentration fermentable sugars from lignocellulosic biomass by using high solids fed-batch enzymatic hydrolysis. Biochem Eng J. 2021;176:108186. doi: 10.1016/j.bej.2021.108186
- Mazzoli R, Olson DG. Chapter three - Clostridium thermocellum: a microbial platform for high-value chemical production from lignocellulose. In: editors Gadd GM Sariaslani S. Advances in applied microbiology. Academic Press; 2020Vol. 113pp. 111–161. doi:10.1016/bs.aambs.2020.07.004
- Thakur A, Sharma K, Mutreja R, et al. Thermostable enzymes from Clostridium thermocellum. In: Thatoi H, Mohapatra S Das SK, editors. Bioprospecting of enzymes in industry, Healthcare and sustainable environment. Springer; 2021. pp. 251–267. DOI:10.1007/978-981-33-4195-1_12.
- Nhim S, Waeonukul R, Uke A, et al. Biological cellulose saccharification using a coculture of Clostridium thermocellum and thermobrachium celere strain A9. Appl Microbiol Biotechnol. 2022;106(5):2133–2145. doi: 10.1007/s00253-022-11818-0
- Poudel S, Giannone RJ, Rodriguez M, et al. Integrated omics analyses reveal the details of metabolic adaptation of Clostridium thermocellum to lignocellulose-derived growth inhibitors released during the deconstruction of switchgrass. Biotechnol Biofuels. 2017;10(1):14. doi: https://doi.org/10.1186/s13068-016-0697-5
- Rajulapati V, Goyal A. Molecular Cloning, expression and characterization of Pectin Methylesterase (CtPME) from Clostridium thermocellum. Mol Biotechnol. 2017;59(4):128–140. doi: 10.1007/s12033-017-9997-7
- Berger E, Zhang D, Zverlov VV, et al. Two noncellulosomal cellulases of Clostridium thermocellum, Cel9I and Cel48Y, hydrolyse crystalline cellulose synergistically. FEMS Microbiol Lett. 2007;268(2):194–201. doi: 10.1111/j.1574-6968.2006.00583.x
- Furukawa K, Ichikawa S, Nigorikawa M, et al. Enhanced production of reducing sugars from transgenic rice expressing exo-glucanase under the control of a senescence-inducible promoter. Transgenic Res. 2014;23(3):531–537. doi: 10.1007/s11248-014-9786-z
- Kiyoshi K, Furukawa M, Seyama T, et al. Butanol production from alkali-pretreated rice straw by co-culture of Clostridium thermocellum and Clostridium saccharoperbutylacetonicum. Biores Technol. 2015;186:325–328. doi: 10.1016/j.biortech.2015.03.061
- Pluvinage B, Fillo A, Massel P, et al. Structural analysis of a family 81 glycoside hydrolase implicates its recognition of β-1,3-glucan quaternary structure. Structure. 2017;25(9):1348–1359.e3. doi: 10.1016/j.str.2017.06.019
- Basit A, Liu J, Rahim K, et al. Thermophilic xylanases: from bench to bottle. Crit Rev Biotechnol. 2018;38(7):989–1002. doi: 10.1080/07388551.2018.1425662
- Moraïs S, Heyman A, Barak Y, et al. Enhanced cellulose degradation by nano-complexed enzymes: synergism between a scaffold-linked exoglucanase and a free endoglucanase. J Biotechnol. 2010;147(3):205–211. doi: 10.1016/j.jbiotec.2010.04.012
- Stern J, Moraïs S, Lamed R, et al. Adaptor Scaffoldins: an original strategy for extended Designer cellulosomes, inspired from nature. MBio. 2016;7(2):e00083–16. doi: 10.1128/mBio.00083-16
- Ahmad S, Sajjad M, Altayb HN, et al. Engineering processive cellulase of Clostridium thermocellum to divulge the role of the carbohydrate‐binding module. Biotech And App Biochem. 2022;70(1):290–305. bab.2352. doi: 10.1002/bab.2352.
- Zheng Y, Tao L, Yang X, et al. Comparative study on pyrolysis and catalytic pyrolysis upgrading of biomass model compounds: thermochemical behaviors, kinetics, and aromatic hydrocarbon formation. J Energy Inst. 2019;92(5):1348–1363. doi: 10.1016/j.joei.2018.09.006
- Sansaniwal SK, Rosen MA, Tyagi SK. Global challenges in the sustainable development of biomass gasification: an overview. Renew Sust Energ Rev. 2017;80:23–43. doi: 10.1016/j.rser.2017.05.215
- Shetty D, Joshi A, Dagar SS, et al. Bioaugmentation of anaerobic fungus orpinomyces joyonii boosts sustainable biomethanation of rice straw without pretreatment. Biomass Bioenergy. 2020;138:105546. doi: 10.1016/j.biombioe.2020.105546
- Braga Nan L, Trably E, Santa-Catalina G, et al. Biomethanation processes: new insights on the effect of a high H2 partial pressure on microbial communities. Biotechnol Biofuels. 2020;13(1):141. doi: https://doi.org/10.1186/s13068-020-01776-y
- Dar RA, Parmar M, Dar EA, et al. Biomethanation of agricultural residues: potential, limitations and possible solutions. Renew Sust Energ Rev. 2021;135:110217. doi: 10.1016/j.rser.2020.110217
- Perkins G, Batalha N, Kumar A, et al. Recent advances in liquefaction technologies for production of liquid hydrocarbon fuels from biomass and carbonaceous wastes. Renew Sust Energ Rev. 2019;115:109400. doi: 10.1016/j.rser.2019.109400
- Li B, Yang T, Li R, et al. Co-generation of liquid biofuels from lignocellulose by integrated biochemical and hydrothermal liquefaction process. Energy. 2020;200:117524. doi: 10.1016/j.energy.2020.117524
- Cheah WY, Sankaran R, Show PL, et al. Pretreatment methods for lignocellulosic biofuels production: Current advances, challenges and future prospects. Biofuel Res J. 2020;7(1):1115–1127. doi: 10.18331/BRJ2020.7.1.4
- Lo E, Brabo-Catala L, Dogaris I, et al. Biochemical conversion of sweet sorghum bagasse to succinic acid. J Biosci Bioeng. 2020;129(1):104–109. doi: 10.1016/j.jbiosc.2019.07.003
- Mateo S, Mateo P, Barbanera M, et al. Acid hydrolysis of olive tree leaves: preliminary study towards biochemical conversion. Processes. 2020;8(8):886. doi: 10.3390/pr8080886
- Meenakshisundaram S, Fayeulle A, Léonard E, et al. Combined biological and chemical/Physicochemical pretreatment methods of lignocellulosic biomass for bioethanol and Biomethane energy production—A review. Appl Microbiol. 2022;2(4):716–734. doi: 10.3390/applmicrobiol2040055
- Oliva JM, Negro MJ, Álvarez C, et al. Fermentation strategies for the efficient use of olive tree pruning biomass from a flexible biorefinery approach. Fuel. 2020;277:118171. doi: 10.1016/j.fuel.2020.118171
- Asemoloye MD, Ahmad R, Jonathan SG. Synergistic action of rhizospheric fungi with megathyrsus maximus root speeds up hydrocarbon degradation kinetics in oil polluted soil. Chemosphere. 2017;187:1–10. doi: 10.1016/j.chemosphere.2017.07.158
- Prasad RK, Chatterjee S, Mazumder PB, et al. Study on cellulase (Β-1,4-endoglucanase) activity of gut bacteria of sitophilus oryzae in cellulosic waste biodegradation. Bioresour Technol Rep. 2019;7:100274. doi: 10.1016/j.biteb.2019.100274
- Tsegaye B, Balomajumder C, Roy P. Icrobial delignification and hydrolysis of lignocellulosic biomass to enhance biofuel production: an overview and future prospect. Bull National Res Centre. 2019;43(1):51. doi: 10.1186/s42269-019-0094-x
- Pérez J, Muñoz-Dorado J, De La Rubia T, et al. Biodegradation and biological treatments of cellulose, hemicellulose and lignin: an overview. Int Microbiol. 2002;5(2):53–63. doi: 10.1007/s10123-002-0062-3
- Goodell B. Fungi involved in the biodeterioration and bioconversion of lignocellulose substrates. In: Benz JP Schipper K, editors. Genetics and biotechnology. Springer International Publishing; 2020. pp. 369–397. DOI:10.1007/978-3-030-49924-2_15.
- Saadeddin A. The complexities of hydrolytic enzymes from the termite digestive system. Crit Rev Biotechnol. 2012;34(2):115–122. doi: 10.3109/07388551.2012.727379
- Woo HL, Hazen TC, Simmons BA, et al. Enzyme activities of aerobic lignocellulolytic bacteria isolated from wet tropical forest soils. Syst Appl Microbiol 2012. 2014;37(1):60–67. doi: 10.1016/j.syapm.2013.10.001
- Saini JK, Saini R, Tewari L. Lignocellulosic agriculture wastes as biomass feedstocks for second-generation bioethanol production: concepts and recent developments. Biotech. 2015;5(4):337–353. doi: 10.1007/s13205-014-0246-5
- Hemmerle L, Maier BA, Bortfeld-Miller M, et al. Dynamic character displacement among a pair of bacterial phyllosphere commensals in situ. Nat Commun. 2022;13(1):2836. doi: 10.1038/s41467-022-30469-3
- Zang H, Du X, Wang J, et al. Insight into cold-active xylanase production and xylan degradation pathways in psychrotrophic Acinetobacter sp. HC4 from the cold region of China. Cellul. 2020;27(13):7575–7589. doi: 10.1007/s10570-020-03286-4
- Blake AD, Beri NR, Guttman HS, et al. The complex physiology of cellvibrio japonicus xylan degradation relies on a single cytoplasmic β‐xylosidase for xylo‐oligosaccharide utilization. Mol Microbiol. 2018;107(5):610–622. doi: 10.1111/mmi.13903
- Šuchová K, Fehér C, Ravn JL, et al. Cellulose- and xylan-degrading yeasts: enzymes, applications and biotechnological potential. Biotechnol Adv. 2022;59:107981. doi: 10.1016/j.biotechadv.2022.107981
- Helal GA, Khalil RR, Galal YG, et al. Studies on cellulases of some cellulose-degrading soil fungi. Arch Microbiol. 2022;204(1):1–16. doi: 10.1007/s00203-021-02705-9
- Lamilla C, Pavez M, Santos A, et al. Bioprospecting for extracellular enzymes from culturable actinobacteria from the South Shetland Islands, Antarctica. Polar Biology. 2017;40(3):719–726. doi: 10.1007/s00300-016-1977-z
- Rajkumar J, Dilipan E, Ramachandran M, et al. Bioethanol production from seagrass waste, through fermentation process using cellulase enzyme isolated from marine actinobacteria. Vegetos. 2021;34(3):581–591. doi: 10.1007/s42535-021-00239-5
- Arelli V, Mamindlapelli NK, Juntupally S, et al. Solid-state anaerobic digestion of sugarcane bagasse at different solid concentrations: impact of bio augmented cellulolytic bacteria on methane yield and insights on microbial diversity. Biores Technol. 2021;340:125675. doi: 10.1016/j.biortech.2021.125675
- Manni A, Filali-Maltouf A, Manni A, et al. Diversity and bioprospecting for industrial hydrolytic enzymes of microbial communities isolated from deserted areas of south-east Morocco. AIMS microbiol. 2022;8(1):5–25. doi: 10.3934/microbiol.2022002
- Shi YL, Sun Y, Ruan ZY, et al. Cellulomonas telluris sp. Nov., an endoglucanase-producing actinobacterium isolated from Badain Jaran desert sand. Int J Syst Evol Microbiol. n.d;70(1):631–635. doi: 10.1099/ijsem.0.003806
- Yang M, Zhao J, Yuan Y, et al. Comparative metagenomic discovery of the dynamic cellulose-degrading process from a synergistic cellulolytic microbiota. Cellul. 2021;28(4):2105–2123. doi: 10.1007/s10570-020-03671-z
- Paul M, Nayak DP, Thatoi H. Optimization of xylanase from Pseudomonas mohnii isolated from simlipal biosphere reserve, Odisha, using response surface methodology. J Genet Eng Biotechnol. 2020;18(1):1–19. doi: 10.1186/s43141-020-00099-7
- Ingle AP, Chandel AK, Silva SS. Biorefining of lignocellulose into valuable products. In: Ingle A, Chandel A Silva S editors. Lignocellulosic biorefining technologies 2020. 1st ed. Wiley. pp. 1–5. 10.1002/9781119568858.ch1.
- Tushar MSHK, Dutta A. Efficiency analysis of crude versus pure cellulase in industry. In: Srivastava N, Srivastava M, Mishra P Gupta V, editors. Biofuel production technologies: critical analysis for sustainability. Springer; 2020. pp. 283–298. DOI:10.1007/978-981-13-8637-4_10.
- Gavande PV, Nath P, Kumar K, et al. Highly efficient, processive and multifunctional recombinant endoglucanase RfGH5_4 from ruminococcus flavefaciens FD-1 v3 for recycling lignocellulosic plant biomasses. Int j biol macromol. 2022;209:801–813. doi: 10.1016/j.ijbiomac.2022.04.059
- Chang H, Wohlschlager L, Csarman F, et al. Real-time measurement of cellobiose and glucose formation during enzymatic biomass hydrolysis. Anal Chem. 2021;93(21):7732–7738. doi: 10.1021/acs.analchem.1c01182
- Huang C, Feng Y, Patel G, et al. Production, immobilization and characterization of beta-glucosidase for application in cellulose degradation from a novel Aspergillus versicolor. Int j biol macromol. 2021;177:437–446. doi: 10.1016/j.ijbiomac.2021.02.154
- Nidetzky B, Zhong C. Phosphorylase-catalyzed bottom-up synthesis of short-chain soluble cello-oligosaccharides and property-tunable cellulosic materials. Biotechnol Adv. 2021;51:107633. doi: 10.1016/j.biotechadv.2020.107633
- Adebami GE, Kuila A, Ajunwa OM, et al. Genetics and metabolic engineering of yeast strains for efficient ethanol production. J Food Process Eng. 2022;45(7). doi: https://doi.org/10.1111/jfpe.13798
- Lan Q, Duan Y, Wu P, et al. Coordinately express hemicellulolytic enzymes in Kluyveromyces marxianus to improve the saccharification and ethanol production from corncobs. Biotechnol Biofuels. 2021;14(1):220. doi: https://doi.org/10.1186/s13068-021-02070-1
- Sun Y, Li X, Wu L, et al. The advanced performance of microbial consortium for simultaneous utilization of glucose and xylose to produce lactic acid directly from dilute sulfuric acid pretreated corn stover. Biotechnol Biofuels. 2021;14(1):233. doi: https://doi.org/10.1186/s13068-021-02085-8
- Gao M, Ploessl D, Shao Z. Enhancing the co-utilization of biomass-derived mixed sugars by yeasts. Front Microbiol. 2019;9. doi: 10.3389/fmicb.2018.03264
- Adebami GE. Adebayo-tayo BC.Chapter 8—development of cellulolytic strain by genetic engineering approach for enhanced cellulase production. In: Kuila A Sharma V, editors. Genetic and metabolic engineering for improved biofuel production from lignocellulosic biomass. Elsevier; 2020. pp. 103–136. DOI:10.1016/B978-0-12-817953-6.00008-7.
- Kim SR, Park YC, Jin YS, et al. Strain engineering of Saccharomyces cerevisiae for enhanced xylose metabolism. Biotechnol Adv. 2013;31(6):851–861. doi: 10.1016/j.biotechadv.2013.03.004
- Chu BCH, Lee H. Genetic improvement of Saccharomyces cerevisiae for xylose fermentation. Biotechnol Adv. 2007;25(5):425–441. doi: 10.1016/j.biotechadv.2007.04.001
- Peris D, Alexander WG, Fisher KJ, et al. Synthetic hybrids of six yeast species. Nat Commun. 2020;11(1):2085. doi: https://doi.org/10.1038/s41467-020-15559-4
- Zhao Z, Xian M, Liu M, et al. Biochemical routes for uptake and conversion of xylose by microorganisms. Biotechnol Biofuels. 2020;13(1):21. doi: https://doi.org/10.1186/s13068-020-1662-x
- Knychala MM, dos Santos AA, Kretzer LG, et al. Strategies for efficient expression of heterologous monosaccharide transporters in Saccharomyces cerevisiae. Journal Of Fungi. 2022;8(1):84. doi: 10.3390/jof8010084
- Cai Z, Zhang B, Li Y. Engineering Saccharomyces cerevisiae for efficient anaerobic xylose fermentation: reflections and perspectives. Biotechnol J. 2012;7(1):34–46. doi: 10.1002/biot.201100053
- Yang B, Sun Y, Fu S, et al. Improving the production of riboflavin by introducing a mutant ribulose 5-phosphate 3-epimerase gene in Bacillus subtilis. Front Bioeng Biotechnol. 2021;9. https://www.frontiersin.org/article/10.3389/fbioe.2021.704650
- Jeffries TW. Engineering yeasts for xylose metabolism. Curr Opin Biotechnol. 2006;17(3):320–326. doi: 10.1016/j.copbio.2006.05.008
- Ma M, Liu ZL, Moon J. Genetic engineering of inhibitor-tolerant Saccharomyces cerevisiae for improved xylose utilization in ethanol production. BioEnergy Res. 2012;5(2):459–469. doi: 10.1007/s12155-011-9176-9
- Selim KA, El-Ghwas DE, Easa SM, et al. Bioethanol a microbial biofuel metabolite; new insights of yeasts metabolic engineering. Fermentation. 2018;4(1):16. doi: 10.3390/fermentation4010016
- Li YJ, Wang MM, Chen YW, et al. Engineered yeast with a CO2-fixation pathway to improve the bioethanol production from xylose-mixed sugars. Sci Rep. 2017;7(1):43875. doi: 10.1038/srep43875
- Favaro L, Jansen T, van Zyl WH. Exploring industrial and natural Saccharomyces cerevisiae strains for the bio-based economy from biomass: the case of bioethanol. Crit Rev Biotechnol. 2019;39(6):800–816. doi: 10.1080/07388551.2019.1619157
- Zhang Y, Xie Z, Wang Z, et al. Unveiling the adsorption mechanism of zeolitic imidazolate framework-8 with high efficiency for removal of copper ions from aqueous solutions. Dalton Trans. 2016;45(32):12653–12660. doi: 10.1039/c6dt01827k
- Scordia D, Cosentino SL, Lee JW, et al. Bioconversion of giant reed (arundo donax L.) hemicellulose hydrolysate to ethanol by Scheffersomyces stipitis CBS6054. Biomass Bioenergy. 2012;39:296–305. doi: 10.1016/j.biombioe.2012.01.023
- Zuroff TR, Xiques SB, Curtis WR. Consortia-mediated bioprocessing of cellulose to ethanol with a symbiotic Clostridium phytofermentans/yeast co-culture. Biotechnol Biofuels. 2013;6(1):59. doi: https://doi.org/10.1186/1754-6834-6-59
- Damayanti D, Supriyadi D, Amelia D, et al. Conversion of lignocellulose for bioethanol production, applied in bio-polyethylene terephthalate. Polymers. 2021;13(17):2886. doi: 10.3390/polym13172886
- Mussatto SI, Yamakawa CK, van der Maas L, et al. New trends in bioprocesses for lignocellulosic biomass and CO2 utilization. Renew Sust Energ Rev. 2021;152:111620. doi: 10.1016/j.rser.2021.111620
- Lee CR, Sung BH, Lim KM, et al. Co-fermentation using recombinant Saccharomyces cerevisiae yeast strains hyper-secreting different cellulases for the production of cellulosic bioethanol. Sci Rep. 2017;7(1):4428. doi: 10.1038/s41598-017-04815-1
- Nakatani Y, Yamada R, Ogino C, et al. Synergetic effect of yeast cell-surface expression of cellulase and expansin-like protein on direct ethanol production from cellulose. Microb Cell Fact. 2013;12(1):66. doi: https://doi.org/10.1186/1475-2859-12-66
- Kricka W, Fitzpatrick J, Bond U. Metabolic engineering of yeasts by heterologous enzyme production for degradation of cellulose and hemicellulose from biomass: a perspective. Front Microbiol. 2014;5. doi: 10.3389/fmicb.2014.00174
- Seok JY, Yang J, Choi SJ, et al. Directed evolution of the 3-hydroxypropionic acid production pathway by engineering aldehyde dehydrogenase using a synthetic selection device. Metab Eng. 2018;47:113–120. doi: 10.1016/j.ymben.2018.03.009
- Roell GW, Zha J, Carr RR, et al. Engineering microbial consortia by division of labor. Microb Cell Fact. 2019;18(1):35. doi: https://doi.org/10.1186/s12934-019-1083-3
- Yanase S, Yamada R, Kaneko S, et al. Ethanol production from cellulosic materials using cellulase-expressing yeast. Biotechnol J. 2010;5(5):449–455. doi: 10.1002/biot.200900291
- Baek S-H, Kim S, Lee K, et al. Cellulosic ethanol production by combination of cellulase-displaying yeast cells. Enzyme Microb Technol. 2012;51(6):366–372. doi: 10.1016/j.enzmictec.2012.08.005
- Chen L, Du J-L, Zhan Y-J, et al. Consolidated bioprocessing for cellulosic ethanol conversion by cellulase–xylanase cell-surfaced yeast consortium. Prep Biochem Biotechnol. 2018;48(7):653–661. doi: 10.1080/10826068.2018.1487846
- Xiao W, Li H, Xia W, et al. Co-expression of cellulase and xylanase genes in Saccharomyces cerevisiae toward enhanced bioethanol production from corn stover. Bioengineered. 2019;10(1):513–521. doi: 10.1080/21655979.2019.1682213
- Kwak S, Jin Y-S. Production of fuels and chemicals from xylose by engineered Saccharomyces cerevisiae: a review and perspective. Microb Cell Fact. 2017;16(1):82. doi: https://doi.org/10.1186/s12934-017-0694-9
- Robak K, Balcerek M. Review of second generation bioethanol production from residual biomass. Food Technol Biotechnol. 2018;56(2):174–187. doi: 10.17113/ftb.56.02.18.5428
- Mussatto SI, Machado EMS, Carneiro LM, et al. Sugars metabolism and ethanol production by different yeast strains from coffee industry wastes hydrolysates. Appl Energy. 2012;92:763–768. doi: 10.1016/j.apenergy.2011.08.020
- Kumari R, Pramanik K. Bioethanol production from ipomoea carnea biomass using a potential hybrid yeast strain. Appl Biochem Biotechnol. 2013;171(3):771–785. doi: 10.1007/s12010-013-0398-5
- Henningsen BM, Hon S, Covalla SF, et al. Increasing anaerobic acetate consumption and ethanol yields in Saccharomyces cerevisiae with NADPH-specific alcohol dehydrogenase. Appl Environ Microbiol. 2015;81(23):8108–8117. doi: 10.1128/AEM.01689-15
- Papapetridis I, van Dijk M, Dobbe AP, et al. Improving ethanol yield in acetate-reducing Saccharomyces cerevisiae by cofactor engineering of 6-phosphogluconate dehydrogenase and deletion of ALD6. Microb Cell Fact. 2016;15(1):67. doi: 10.1186/s12934-016-0465-z
- Papapetridis I, Dijk M, Maris AJ, et al. Metabolic engineering strategies for optimizing acetate reduction, ethanol yield and osmotolerance in Saccharomyces cerevisiae. Biotechnol Biofuels. 2017;10(1):107. doi: 10.1186/s13068-017-0791-3
- Wei N, Quarterman J, Kim SR, et al. Enhanced biofuel production through coupled acetic acid and xylose consumption by engineered yeast. Nat Commun. 2013;4(1):2580. doi: 10.1038/ncomms3580
- Kang NK, Lee JW, Ort DR, et al. L-malic acid production from xylose by engineered Saccharomyces cerevisiae. Biotechnology Journal. 2021;2021(3):e2000431. doi: 10.1002/biot.202000431
- Adeboye P, Bettiga M, Olsson L. ALD5, PAD1, ATF1 and ATF2 facilitate the catabolism of coniferyl aldehyde, ferulic acid and p-coumaric acid in Saccharomyces cerevisiae. Sci Rep. 2017;7(1):42635. doi: https://doi.org/10.1038/srep42635
- Wang C, Mas A, Esteve-Zarzoso B. The interaction between Saccharomyces cerevisiae and non-Saccharomyces yeast during Alcoholic fermentation is species and strain specific. Front Microbiol. 7 2016 Apr 13: 502. PMID: 27148191; PMCID: PMC4829597. 10.3389/fmicb.2016.00502
- Wang C, Bao X, Li Y, et al. Cloning and characterization of heterologous transporters in Saccharomyces cerevisiae and identification of important amino acids for xylose utilization. Metab Eng. 2015;30:79–88. doi: 10.1016/j.ymben.2015.04.007
- Shin HY, Nijland JG, de Waal PP, et al. An engineered cryptic Hxt11 sugar transporter facilitates glucose–xylose co-consumption in Saccharomyces cerevisiae. Biotechnol Biofuels. 2015;8(1):176. doi: 10.1186/s13068-015-0360-6
- Shin HY, Nijland JG, de Waal PP, et al. The amino-terminal tail of Hxt11 confers membrane stability to the Hxt2 sugar transporter and improves xylose fermentation in the presence of acetic acid. Biotechnol Bioeng. 2017;114(9):1937–1945. doi: 10.1002/bit.26322
- Nijland JG, Shin HY, Boender LGM, et al. Improved xylose metabolism by a CYC8 mutant of Saccharomyces cerevisiae. Appl Environ Microbiol. 2017;83(11):e00095–17. doi: 10.1128/AEM.00095-17
- Wu R, Chen D, Cao S, et al. Enhanced ethanol production from sugarcane molasses by industrially engineered Saccharomyces cerevisiae via replacement of the PHO4 gene. RSC Adv. 2020;10(4):2267–2276. doi: 10.1039/C9RA08673K
- Jagtap RS, Mahajan DM, Mistry SR, et al. Improving ethanol yields in sugarcane molasses fermentation by engineering the high osmolarity glycerol pathway while maintaining osmotolerance in Saccharomyces cerevisiae. Appl Microbiol Biotechnol. 2019;103(2):1031–1042. doi: https://doi.org/10.1007/s00253-018-9532-1
- Swinnen S, Henriques SF, Shrestha R, et al. Improvement of yeast tolerance to acetic acid through Haa1 transcription factor engineering: towards the underlying mechanisms. Microb Cell Fact. 2017;16(1):7. doi: https://doi.org/10.1186/s12934-016-0621-5
- Qiu Z, Jiang R. Improving Saccharomyces cerevisiae ethanol production and tolerance via RNA polymerase II subunit Rpb7. Biotechnol Biofuels. 2017;10(1):125. doi: https://doi.org/10.1186/s13068-017-0806-0
- Hasunuma T, Sakamoto T, Kondo A. Inverse metabolic engineering based on transient acclimation of yeast improves acid-containing xylose fermentation and tolerance to formic and acetic acids. Appl Microbiol Biotechnol. 2016;100(2):1027–1038. doi: 10.1007/s00253-015-7094-z
- Hou J, Jiao C, Peng B, et al. Mutation of a regulator Ask10p improves xylose isomerase activity through up-regulation of molecular chaperones in Saccharomyces cerevisiae. Metab Eng. 2016;38:241–250. doi: 10.1016/j.ymben.2016.08.001
- Xu H, Kim S, Sorek H, et al. PHO13 deletion-induced transcriptional activation prevents sedoheptulose accumulation during xylose metabolism in engineered Saccharomyces cerevisiae. Metab Eng 2016a. 2016;34:88–96. doi: 10.1016/j.ymben.2015.12.007
- Hoang P, Ko JK, Gong G, et al. Genomic and phenotypic characterization of a refactored xylose-utilizing Saccharomyces cerevisiae strain for lignocellulosic biofuel production. Biotechnol Biofuels. 2018;11(1):268. doi: https://doi.org/10.1186/s13068-018-1269-7
- Xia PF, et al. Recycling carbon dioxide during xylose fermentation by engineered Saccharomyces cerevisiae. ACS Synth Biol. 2016. doi: 10.1021/acssynbio.6b00167
- Li H, Schmitz O, Alper HS. Enabling glucose/xylose co-transport in yeast through the directed evolution of a sugar transporter. Appl Microbiol Biotechnol. 2016;100(23):10215–10223. doi: 10.1007/s00253-016-7879-8
- Ledesma-Amaro R, Nicaud JM. Metabolic engineering for expanding the substrate range of yarrowia lipolytica. Trends Biotechnol. 2016;34(10):798–809. doi: 10.1016/j.tibtech.2016.04.010
- Yook SD, Kim J, Gong G, et al. High-yield lipid production from lignocellulosic biomass using engineered xylose-utilizing yarrowia lipolytica. GCB Bioenergy. 2020;12(9):670–679. doi: https://doi.org/10.1111/gcbb.12699
- Qiao K, Wasylenko T, Zhou K, et al. Lipid production in Yarrowia lipolytica is maximized by engineering cytosolic redox metabolism. Nat Biotechnol. 2017;35(2):173–177. doi: https://doi.org/10.1038/nbt.3763
- Xu P, Qiao K, Ahn WS, et al. Engineering yarrowia lipolytica as a platform for synthesis of drop-in transportation fuels and oleochemicals. Proc Natl Acad Sci, USA. 2016b;113(39):10848–10853. doi: 10.1073/pnas.1607295113
- Yang K, Qiao Y, Li F, et al. Subcellular engineering of lipase dependent pathways directed towards lipid related organelles for highly effectively compartmentalized biosynthesis of triacylglycerol derived products in Yarrowia lipolytica. Metab Eng. 2019;55:231–238. doi: 10.1016/j.ymben.2019.08.001
- Kim JH, Ryu J, Huh IY, et al. Ethanol production from galactose by a newly isolated Saccharomyces cerevisiae KL17. Bioproc biosyst eng. 2014;37(9):1871–1878. doi: 10.1007/s00449-014-1161-1
- Tanimura A, Nakamura T, Watanabe I, et al. Isolation of a novel strain of Candida shehatae for ethanol production at elevated temperature. Springerplus. 2012;1(1):27. doi: 10.1186/2193-1801-1-27
- da Silva Filho EA, de Melo HF, Antunes DF, et al. Isolation by genetic and physiological characteristics of a fuel-ethanol fermentative Saccharomyces cerevisiae strain with potential for genetic manipulation. J Ind Microbiol Biotechnol. 2005;32(10):481–486. doi: 10.1007/s10295-005-0027-6
- Wang C, Bao XLY, Jiao C, et al. Cloning and characterization of heterologous transporters in Saccharomyces cerevisiae and identification of important amino acids for xylose utilization. Metab Eng. 2015;30:79–88. doi: 10.1016/j.ymben.2015.04.007
- Ko JK, Jung JH, Altpeter F, et al. Largely enhanced bioethanol production through the combined use of lignin-modified sugarcane and xylose fermenting yeast strain. Biores Technol. 2018;256:312–320. doi: 10.1016/j.biortech.2018.01.123
- Ko JK, Lee SM. Advances in cellulosic conversion to fuels: engineering yeasts for cellulosic bioethanol and biodiesel production. Curr Opin Biotechnol. 2018;50:72–80. doi: 10.1016/j.copbio.2017.11.007
- Tran HN, Ko P, Gong JK, et al. Improved simultaneous co-fermentation of glucose and xylose by Saccharomyces cerevisiae for efficient lignocellulosic biorefinery. Biotechnol Biofuels. 2020;13(1):12. doi: 10.1186/s13068-019-1641-2