ABSTRACT
The tumor microenvironment is recognized as playing a significant role in the behavior of tumor cells and their progression to metastasis. However, tools to manipulate the tumor microenvironment directly, and image the consequences of this manipulation with single cell resolution in real time in vivo, are lacking. We describe here a method for the direct, local manipulation of microenvironmental parameters through the use of an implantable Induction Nano Intravital Device (iNANIVID) and simultaneous in vivo visualization of the results at single-cell resolution. As a proof of concept, we deliver both a sustained dose of EGF to tumor cells while intravital imaging their chemotactic response as well as locally induce hypoxia in defined microenvironments in solid tumors.
Introduction
With metastasis responsible for approximately 90% of cancer mortality,Citation1 research into the mechanisms driving metastasis and therapeutics that counter this process, is of paramount importance. Though advances in early detection have had some positive impact on metastasis and mortality rates, evidence is building that tumor cell dissemination is not a late-stage event, but rather an inherent property of the tumor, even at very early stages.Citation2-4 In addition to the inherent metastatic potential of tumor cells conferred by alterations in their gene expression, it is increasingly understood that other factors found in the microenvironment external to these cells play a role in determining clinical outcome.Citation5,6 Experiments designed to identify and test these mechanisms have been limited by the lack of tools that can be used in vivo and in combination with high-resolution imaging to interrogate how cells communicate with their surroundings.Citation7 Tools for use in vivo are required because conventional 2D in vitro assaysCitation8 do not adequately reflect the topography or diversity of environments encountered by cells in vivo.Citation4,8 Other techniques, including 3D in vitro assays,Citation9-11 systemic applications of drugs,Citation12 functional blocking antibodies,Citation13 or inducible genetic alterationsCitation14 may create many off-target effects that can confound experimental results.
To date, only one assay reported in the literature, the in vivo invasion assay,Citation15 successfully addresses these limitations. This technique induces local chemotaxis in solid tumors in vivo and attracts and collects only cells which constitute the tumor's motile fraction. Despite the success of the in vivo invasion assay, direct imaging of the needle opening is prevented by the orientation and lack of transparency of the needle and thus the intravital imaging results are of lower resolution than desired. This leaves the behavior and dynamics of the cells, and their chemotactic response, an incompletely described process. As has been shown in the literature, the ability to image tumor cell interactions with both extracellular matrix and other resident non-motile cellsCitation16,17 yields a wealth of information. Thus the combination of microenvironmental control with visualization of single cells during chemotaxis would add an important new dimension of understanding.
In our previous workCitation18 we described a microfabricated, self-contained device designed to slowly release a chemical factor in vitro. Here, we have extended this device to be the first technique able to accomplish the much more difficult task of directly altering the local tumor microenvironment in vivo while imaging intravitally. This novel device, which we call the Induction Nano Intravital Device (iNANIVID), is a redesign of the previously validated in vitro NANIVID.Citation18 It adds the functionality of the in vivo invasion assay and allows direct imaging of single cell behavior in response to its released signals using multiphoton microscopy, something not previously possible. We validate its use by in vivo imaging of tumor cell chemotaxis in solid tumors and demonstrate its general applicability for use with many factors by inducing hypoxia in chicken embryo chorioallantoic membrane (CAM) grown tumors.
Results
Device design
In this work, the previously described in vitro NANIVIDCitation18 was redesigned and optimized for in vivo implementation with a new geometry specifically intended for multiphoton-based intravital imaging. The new design described here is 3.0 mm × 1.3 mm × 150 µm (), with a pointed front to ease implantation into solid tumors and with the center of the outlet located ∼150 µm from the back edge (). When fully inserted into the tumor, the outlet is designed to be located at an ideal depth for multiphoton microscopy of most solid tissues (e.g. ∼100–200 µmCitation19). While the device outlet is located only a short distance below the tumor surface, the overall length of the device is much longer. This length was chosen for several reasons. First, the extra length ensures that there is sufficient surface area for the PDMS adhesive layer to provide proper adhesion to the two glass surfaces. Second, a longer device design makes for a shallow angle at the insertion tip which spreads the tissue gently upon insertion. Shortening the device would require increasing the angle of the pointed end which would lead to a more dramatic spread of the tissue as the device is inserted. Finally, tumor tissue is typically under high interstitial pressure and the extra length acts to retain the device in the tumor and prevent it from slipping back out. The edge of the device is made to be an obtuse angle to allow illumination of the region of the tumor near the device outlet (yellow triangle, Fig. S1, left). In other designs, the corner of the device would obstruct viewing of the outlet while implanted in vivo, resulting in shadowing and obscuration of cell migration (red triangle, Fig. S1, right).
Figure 1. Induction Nano Intravital Device (iNANIVID) optimized for intravital imaging. (A) iNANIVID next to a 17.9 mm U.S. dime for reference. (B) Use of the iNANIVID for intravital imaging within solid tumors imposes strict requirements of the design of the device. Insertion into stiff tumor tissue requires a small form factor (3 mm) and a sharp point. The chamber outlet is designed to be at the proper depth for multiphoton imaging when the device is fully inserted into the tumor (back edge flush with the tumor surface). Fluorescent beads placed in etched wells within the cover aid in locating the device outlet under the microscope. An obtuse shape prevents shadowing of the outlet during imaging. (C) The small size of the iNANIVID makes manual manipulation challenging. An insertion tool designed to facilitate implantation into the tumor tissue aids in manipulation and insertion. The front edge of the tool matches the obtuse shape of the iNANIVID and ensures insertion at the proper angle. The plunger ensures insertion to the proper depth. Scale bar = 10 mm. (D) 3D computer aided design rendering of the device with the direction of insertion indicated. Yellow indicates the back surface which is visible under the microscope. An asterisk indicates the location on the back surface, directly above the device outlet. (E) Schematic of a cross section of the tumor with the implanted device illustrating a diffusion gradient emanating from the device outlet. Red dots indicate the fluorescent bead chambers etched into the cover. (F) View of the tumor with the implanted device as would be viewed from the microscope perspective. Asterisk indicates the device outlet.
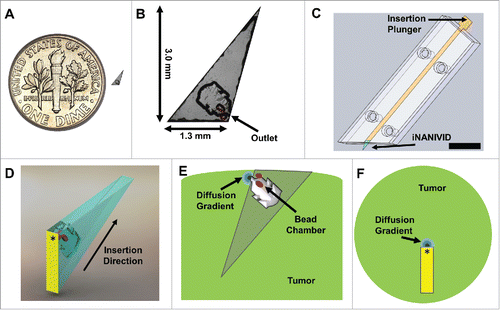
Insertion tool
Because of the iNANIVID's small size, manual manipulation is difficult. We have thus developed a transparent insertion tool to reproducibly implant the device into the tumor ensuring efficient experimental setup and proper orientation for multiphoton microscopy (, Fig. S2). With this setup, the iNANIVID is loaded into a small channel and a plunger is used to push it into the tumor, positioning the back edge flush with the tumor surface and ensuring that the outlet is at the proper depth for imaging. The front of the insertion tool is angled to match the shape of the iNANIVID and ensure that, after insertion, the back edge of the device is parallel to the tumor surface. shows a 3D computer aided design (CAD) rendering of the device with the direction of insertion indicated. For clarity, the back surface which is visible under the microscope has been pseudo-colored in yellow and the point above the device outlet marked with an asterisk. shows a schematic of a cross section of the tumor with the implanted device. A diffusion gradient is shown emanating from the device opening and red dots indicate the chambers etched into the cover which contain fluorescent polystyrene beads used to locate the outlet. shows a top view of the tumor with implanted device. The yellow pseudo-colored back surface and device outlet (asterisk) are shown as would be viewed from the microscope's perspective.
Fixturing
Artifact-free imaging is essential for visualizing and measuring motility at single cell resolution in vivo and can only be attained by proper fixturing of the tissue (complete isolation from all sources of motion and vibration). To this end, we have developed the following fixturing process to immobilize the animal over the course of the experiment. After skin flap surgery, the tumor is supported and stabilized by attaching a rigid foam back (). A hydration chamber made of soft rubber was placed around the tumor and filled with PBS (). The iNANIVID is inserted into the tumor, which is then fit into a custom-built stage insert for imaging (). Breathing motion artifacts (Supplemental Movie 1) as well as specimen drift from dehydration (Supplemental Movie 2) create distortion in the images and make data interpretation difficult. These artifacts are dramatically reduced or even eliminated by this fixturing technique (Supplemental Movie 3).
Figure 2. Tumor fixturing. Visualization of single cell movement within a tissue requires motion and artifact free imaging that can be obtained by proper tissue fixturing. (A) Supine view of a mouse after skin flap surgery to expose the underlying mammary tumor. (B) A hole slightly larger than the tumor is cut into a sheet of rubber and placed around the tumor forming a hydration chamber that is filled with PBS to keep the tissue hydrated over the course of the experiment. To provide support, plastic-backed foam is attached to the skin behind the tumor with surgical staples. (C) Side view showing the tumor within the hydration chamber with the fixturing apparatus in place. Strips of hard rubber are affixed with tape to the foam backing to immobilize the tissue to the microscope stage adaptor, without causing tissue compression. iNANIVID devices are inserted into the tumor and a coverslip is held in place with an O-ring.
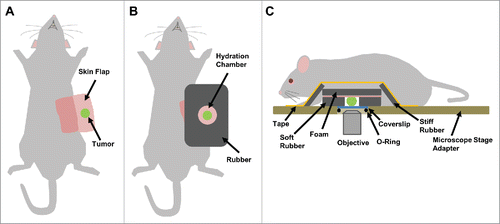
In vivo chemotaxis assays
Hydrogel containing a chemoattractant was loaded into the reservoir of the iNANIVID and the device was sealed with a cover containing fluorescent polystyrene beads to mark the outlet. Previously, the hydrogel matrix had been optimized to establish and maintain a physiologically relevant epidermal growth factor (EGF) gradient, which was sufficient to induce chemotaxis in metastatic mammary tumor cells in culture.Citation18
Here, migration experiments were performed in vivo using an iNANIVID loaded with 2 µM EGF and implanted into a tumor composed of MDA MB 231 cells expressing the Dendra2 protein. Dendra2 is a monomeric fluorescent protein which emits green light in its native state, but upon exposure to 405 nm light undergoes a switch to red emission.Citation20 A region of cells 150 µm away from the device was photoswitched and tracked over a 4 hour timelapse ().
Figure 3. MDA-MB-231 tumor cells expressing Dendra2 migrate in vivo up a chemoattractant gradient produced by an iNANIVID loaded with 2 µM EGF. After iNANIVID insertion, a region of the tumor approximately 150 µm away from the device was photocoverted (red). Non-photoconverted cells can be seen closer to the device (green) with collagen fibers (blue) visualized by second harmonic generation. Timelapse multiphoton microscopy was performed every 3 minutes for 4 hours to obtain a Z-stack of the tumor. (A) Image of the iNANIVID outlet region taken 120 minutes after device insertion (time noted on top right). An approximate outline of the top surface of the iNANIVID (see ) at the bottom of the image has been overlaid for clarity (white). The device outlet location has been marked with an asterisk. Cell outlines are shown from manual tracing to track migration over the time lapse. Scale bar = 100 µm. (B) At 145 minutes, cells have begun to migrate up the gradient of EGF produced by the iNANIVID. Cells have been outlined and their paths highlighted. (C) By 168 minutes, additional cells have responded to the gradient and migrated toward the device. Multiple cells have moved over 100 µm. (D) Vector plot showing directional migration of 33 tumor cells from 3 experiments. Each vector represents the net displacement of an individual cell plotted on a common origin.
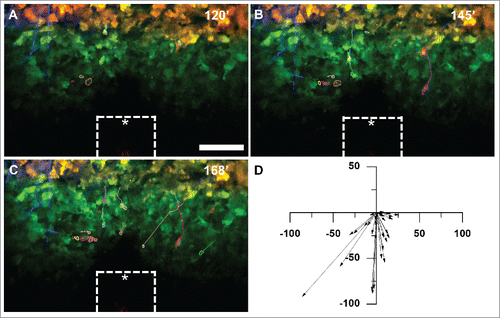
The migration of photoswitched cells toward the iNANIVID was observed approximately 2 hours after device insertion due to the time required for EGF diffusion to establish the gradient (Supplemental Movie 4). Cells that moved at least one cell length over the time course were manually traced to track cell motility. 145 minutes after device implantation, substantial migration of responsive cells was observed (). By 168 minutes, many of the cells had migrated more than 50 µm from their starting position (). Overlaying the displacement vectors for each cell tracked during three separate experiments demonstrated that the migration was directional toward the device (). Similar chemotaxis of tumor cells toward the iNANIVID has also been observed using a PyMT mouse model (data not shown).
Control migration assays
To examine the background level of tumor cell motility, we imaged tumors both prior to implanting any devices and, following the same protocol as above, with devices loaded with hydrogel containing solely PBS (). Time lapse multiphoton microscopy was again used to acquire images at intervals comparable to the EGF experiments. Little movement was observed either before implantation, at 145 minutes (), or 168 minutes after device implantation (). Few cells (<1%) moved more than one cell diameter in the control samples. Overall, the migration of all tracked cells showed no directional preference (). A low background level of motility was observed throughout the time-lapse both prior to implantation and using the control device (Supplemental Movie 5) indicating that the presence of the device has no adverse effects on cell motility.
Figure 4. Tumor cells do not migrate toward an iNANIVID loaded with hydrogel that does not contain EGF. Timelapse multiphoton microscopy was performed with an iNANIVID that did not contain a chemoattractant as a control. Experimental procedure was the same as that of . An approximate outline of the top surface of the iNANIVID (see ) at the bottom of the image has been overlaid for clarity (white). (A) Image taken 120 minutes post iNANIVID insertion. Since cell movement was random and to a lesser extent than in the EGF experiments, the 20 most motile cells in the timelapse were chosen for tracking. Scale bar = 100 µm. (B) At 145 minutes, most cells have moved less than 20 µm with no directional trend toward the iNANIVID. (C) At 168 minutes, only random migration is observed and most cells have moved less than 20 µm. (D) Vector plot showing displacement of 46 tumor cells after 51 minutes have elapsed. (D) Vector plot showing displacement of 46 tumor cells (from 3 experiments) after 51 minutes have elapsed.
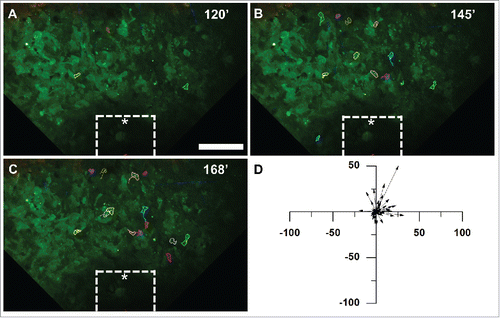
Cell motility parameters
To quantify the response to the EGF gradient, cell motility parameters were derived from the tracks of cells that migrated at least 10 µm in triplicate experiments. Cell tracking was performed prior to NANIVID insertion, and in experiments using devices loaded with EGF, or PBS as a control. Directionality (cell displacement divided by the total path lengthCitation21) was significantly higher for cells responding to the EGF gradient, with a mean value of 0.83 compared with 0.30 and 0.28 for the pre-NANIVID implantation and control experiments, respectively (). Cells in the control experiments changed orientation more often with a two-fold higher turning frequency compared with the EGF experiments (). When migrating toward the EGF iNANIVID, cells had an overall chemotactic index (the cosine of the angle of the cell displacement vector relative to the gradient sourceCitation22) of 0.88. The random cell migration observed in the control experiments yielded a chemotactic index of −0.16 (). While the cells did not exhibit a significantly higher mean velocity in the EGF experiments (), instantaneous cell velocities from 4.0 – 10.0 µm / min were frequently observed (). No cells were observed with velocities above 4.0 µm / min in the control experiments.
Figure 5. Analysis of tumor cell motility parameters in response to the iNANIVID. Cell motility parameters derived from the tracks of cells that migrated at least 10 µm in triplicate experiments. Cells migrated with (A) a significantly higher directionality (***, p<0.001) and (B) a significantly lower turning frequency toward the EGF iNANIVID than in the absence of the iNANIVID or with the control devices loaded with PBS indicating more directional higher efficiency migration (***, p<0.001). (C) Chemotactic index toward the EGF loaded device was also greatly increased compared with the control consistent with panels A&B (**, p<0.01). (D) While mean velocities were not significantly different between the three conditions, the variability of the instantaneous cell velocities was higher with the EGF device and velocities of 4.0 – 10.0 µm / min were frequently observed. (E) Histogram showing distribution of instantaneous velocities of all tracked cells. Most of the cells in the control have a velocity less than 2.0 µm / min and none were observed above 4.0 µm / min.
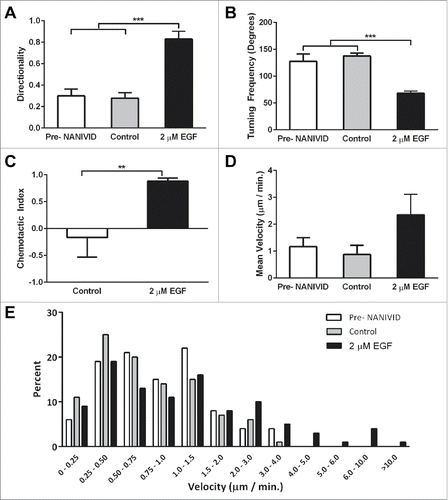
Induction of hypoxic microenvironments with the iNANIVID
Since many biomolecules and chemicals are compatible with the hydrogel, they can alternatively be loaded into the device for delivery to the tumor. Thus, the iNANIVID platform can be used to investigate the role of other microenvironments in promoting metastasis. As a proof of concept, we utilized the iNANIVID to release deferoxamine (DFOM) and cobalt chloride (CoCl2), hypoxia mimeticsCitation23 in patient-derived head and neck squamous carcinoma tumor xenografts (HEp3) implanted in the chicken embryo chorioallantoic membrane (CAM).Citation24
Increased microvessel formation was observed in the DFOM-iNANIVID-influenced tumor compared to the control. Expression of Glucose Transporter 1 (GLUT1), a marker frequently elevated in hypoxia,Citation25 was increased in the HEp3 tumors with CoCl2 and DFOM-iNANIVIDs implants compared PBS loaded devices (). To determine whether the iNANIVIDs were creating defined microenvironments, we collected HEp3 tumor tissue proximal or distal to the device and measured gene expression. In agreement with the immunofluorescence results, GLUT1 mRNA expression was significantly increased in regions of the tumor proximal to the CoCl2-iNANIVIDs when compared with regions that were distal to, and thus not influenced by, the device (). Similarly, a fourfold increase in vascular endothelial growth factor A (VEGF-A) was observed proximal to the CoCl2 iNANIVID as is expected under conditions of hypoxia.Citation26,27
Figure 6. Generation of defined microenvironments via the iNANIVID. (A) Protein expression of membrane GLUT1 (red) and DAPI nuclear stain (blue) is shown in HEp3 tumors exposed to PBS, CoCl2 or DFOM loaded devices. Insets added to show detail. (B) GLUT1 and VEGF-A mRNA expression in the proximal and distal regions to the outlet of the CoCl2-iNANIVIDs (*, p<0.05; ****, p<0.0001). p values calculated with Student's t-test.
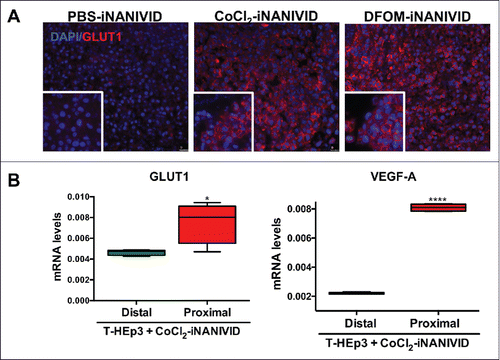
Effect on tumor physiology
Given that the device is designed to alter the microenvironment in a controlled manner, it is important to verify that the simple presence of the device itself does not cause significant alteration to the tumor physiology. To test this, we performed several control experiments.
First, we implanted PBS or DFOM- loaded iNANIVIDs in CAM grown tumors and examined the structure and viability of the tissue by histology. As can be seen in the tissue did not show signs of apoptosis or necrosis even after 3 days of incubation.
Figure 7. Physiological Effects of iNANIVID. (A) Tissue remains viable days after implantation of device. Histological (H&E) staining of HEp3 tumors after 3 days of being exposed to PBS or DFOM-loaded iNANIVIDs. Insets added to show detail. Neither implant shows signs of necrosis or apoptosis, while the DFOM exposed tissue shows a higher density of blood vessels (arrows). (B) Vascular perfusion near outlet is preserved after insertion of device. Stitched merge of two best-focus 5x stereoscope images showing intravenously injected, fluorescently-labeled, high molecular weight dextran (green signal) in flowing vasculature after insertion of a NANIVID (yellow box) into a mammary tumor. The vessels in the region adjacent to the outlet of the device (asterisk) and within the 512µm field of view that would be captured with multiphoton imaging (red square), are still intact and perfused. (C) Quantification of collagen damage. Collagen fibers near device entrances were imaged in 16 different mice. The distance from the edge of the device (yellow bar – opening indicated by asterisk) to the end of the damaged collagen region is measured (red arrow and line). Distances are plotted in a dot plot (right).
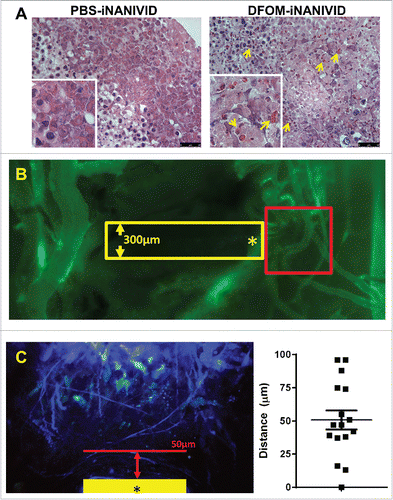
Next, to verify that the vasculature of the tissue is not significantly altered within the imaging region due to insertion of the device, we implanted an iNANIVID into a non-fluorescent PyMT transplant tumor approximately 0.7 cm in diameter. We then intravenously injected a fluorescently-labeled high molecular weight dextran (500 kD FITC) into the mouse. This has the effect of only labeling fully perfused, undamaged, flowing vessels. shows a stitched merge of two best-focus 5x stereoscope images of the tumor after insertion of the device (yellow box) and subsequent injection of the fluorescent dextran (green signal). As can be seen, within the 512 µm field of view at outlet of the device (asterisk) that would be captured with multiphoton intravital imaging (red square) vessels are still intact and perfused.
Finally to ensure that the extracellular matrix of the tissue was not significantly altered, second harmonic generation images from collagen fibers were analyzed for evidence of damage (altered structure and bunching/alignment to device edge). No alteration was observed beyond 100 µm from the device edge with 76% of the measurements (n = 16) showing no damage beyond 75 µm (, mean = 51.9 µm, SD = 28.8 µm).
Extent of gradient
To determine the spatial and temporal extent of the gradient, we loaded a device with fluorescently labeled EGF and inserted it into a non-fluorescent PyMT transplant tumor. We then performed intravital imaging of the device outlet for over 4.5 hours. shows an image of the peak gradient formed by the device 123 min after implantation of the device in the tumor. For clarity, a yellow box representing the size and location of the device has been superimposed. Line averaging of the signal recorded within the red box was used to generate line plots () that show the spatial extent and distribution of the gradient. Taking the peak value from each time point reveals the temporal dynamics of the gradient, shown in Figure 8G.
Figure 8. Spatial and temporal extent of the gradient in vivo. (A) Intravital multiphoton image of fluorescently labeled EGF (green signal) diffusing from the outlet (asterisk) of an implanted device (yellow box, 1.3mm wide). Line averaging the EGF signal within the red box produces plots (B-F) showing the onset (B) and (C), the peak at 123 min (D) and the initial decline (E) and (F) of the gradient. (G) Plotting the peak value for each time point shows the temporal extent of the gradient which lasts well in excess of 4 hours.
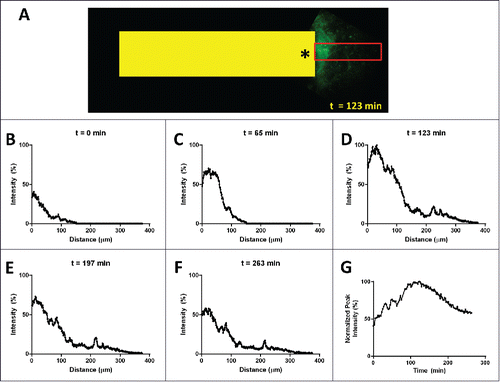
Discussion
It is now widely recognized that numerous interactions between tumor cells and their microenvironment help to drive tumor malignancy.Citation28,29 Chemotaxis toward gradients of released growth factors (e.g., EGF, HGF, IL-6, IL-8),Citation30,31 responses to regions of local hypoxiaCitation32 and interactions with extracellular matrix degradation productsCitation33 have all been shown to be mechanisms affecting tumor growth and progression. However notable differences in cell behavior have been observed between those grown in culture versus in live animals.Citation34-36 This underpins the importance of understanding just how the microenvironment dominates tumor cell phenotype in vivo. Elucidating the mechanisms by which individual tumor cells interact with their microenvironment is thus believed to be crucial to improved diagnosis and treatment.
Conventional cell migration studies utilize platforms such as wound healingCitation37 and micropipette assaysCitation38,39) that are performed in vitro on 2D substrates that severely limit the topography and diversity of the tumor cell environs.
Attempts to overcome the geometric constraints of 2D cultures represent an advancement (e.g. Boyden chambersCitation9 and 3D matricesCitation10,11,40), but these in vitro assays are still highly artificial environments which do not adequately mimic the variety and complexity of the in vivo milieu.Citation41
Attempts to reconstruct microenvironment-related cell-cell communication in vitro have been of limited value since the number of variables contributing to tumor phenotype in vivo is not understood well enough to create meaningful assays.
Finally, attempts to control and test microenvironmental parameters in vivo have been limited to systemic wide treatments with drugs,Citation12 functional blocking antibodiesCitation13 or inducible genetic alterationsCitation14 which may create many off-target effects that confound observed results. For example, a conditional ablation mouse model has been developed in which macrophages can be systemically eliminated through delivery of a drug.Citation14 Since macrophages serve diverse functions and can be found in large numbers throughout mammary tumors, complete ablation of macrophages, even if transient in nature, can cause adverse secondary effects making for a less than ideal method for studying their role in the tumor microenvironment. Injectable drugs such as chemotherapeuticsCitation12,42 or functional inhibitorsCitation13 are also systemic applications that potentially suffer from poor tumor targeting, toxicity, and organism-wide side effects.
The only assay reported in the literature to date capable of inducing microenvironmental changes on a localized scale that limits the range of effect of test factors is the in vivo invasion assay. In this assay, EGF-loaded needles are inserted directly into tumors to attract and collect only cells which constitute the tumor's motile fraction.Citation15 Isolation of this subpopulation from the rest of the tumor allows gene expression analysis to identify hundreds of genes which are differentially expressed in invasive/migratory cells compared to the bulk tumor population.Citation43
Together, these genes form an invasion signature that correlates with clinical metastatic riskCitation44 and has led to the discovery of an important microenvironmental signaling interaction between tumor associated macrophages and mammary carcinoma cellsCitation45 that directs tumor cell chemotaxis and dissemination in vivo. This interaction was shown to be the result of a paracrine signaling loop in which macrophages releasing EGF and tumor cells releasing colony-stimulating factor 1 (CSF1).Citation46 This loop induces co-migration of the two cell types along collagen fibers toward stable structures lining blood vessels, termed TMEM (Tumor Microenvironment of Metastasis), which act as the doorway for tumor cells to enter into the vascular system.Citation47
The iNANIVID offers several important advantages over the aforementioned techniques. Unlike the Boyden chamber and 3D matrices, the design of the iNANIVID has been optimized according to the experimental requirements for in vivo applications. Further; its miniaturized size and unique shape give it a significant advantage over the in vivo invasion assay. The EGF-loaded needle of the in vivo invasion assay protrudes out from the tumor tissue by tens of millimeters which precludes its use during intravital imaging. The iNANIVID meanwhile, is completely implanted within the tissue (making it suitable for use over extended durations (days) and its outlet is readily accessible for intravital imaging. The area of interest where the device interrogates the local microenvironment can be directly visualized in vivo.
The device is also advantageous compared to other implantable devices such as controlled release beads or hydrogel pellet injections in that the iNANIVID provides a sustained directional release at a depth that can be controlled by design. This more precisely defines the area under direct influence of the released factor than that which can be obtained with a bead or pellet which has omnidirectional release and an uncontrolled depth.
It is crucial to be certain the observed physiological responses are due to the released factor and not the presence of the device itself. We observe no impact on vascular perfusion within the multiphoton imaging field of view of the device outlet, a limit of collagen alteration to within 100 µm of the device edge and no signs of apoptosis or necrosis even after 3 days of implantation.
The device's controlled release of signaling factors allows direct manipulation of the microenvironment using a variety of pathway targets. Its combination with optical imaging allows the altered environment to be directly observed at single cell resolution. In particular, this work has enabled the use of the iNANIVID with time-lapse intravital multiphoton imaging to study changes in the tumor in response to released factors over time. And finally, its ability to act locally allows direct comparison of altered microenvironments with regions of the tumor farther away and not influenced by the iNANIVID, creating an internal control.
To aid with the multiphoton imaging, we developed a fixturing process designed to stabilize the specimen over the course of the experiment and provide artifact free imaging at single cell resolution. In this process, a microscope stage adapter fitted with a removable coverglass window provides a means of accessing the mounted tumor and implanting the device with minimal tissue manipulation and disruption. The insertion tool improves the repeatability of the in vivo experiments when compared to manual insertion of the device. Together, these techniques provide a method for directly altering the tumor microenvironment while imaging the affected area at single cell resolution.
Induction of in vivo tumor cell chemotaxis via the EGF gradient produced by the iNANIVID has validated the device as a tool to manipulate the tumor microenvironment to produce cell behaviors that otherwise occur spontaneously during tumor progression and dissemination. The iNANIVID can autonomously generate gradients of chemoattractants or other factors to induce microenvironmental changes and investigate the role of these factors in promoting metastasis.
The ability to load various factors into the iNANIVID permits generation and testing of a myriad of tumor microenvironments. One such target, hypoxia, was chosen to demonstrate this concept due to its association with tumor progression, dissemination and resistance to therapy.Citation48 To locally mimic hypoxia, CoCl2 and DFOM loaded iNANIVIDs were implanted in solid tumors. Delivery of these chemicals resulted in an upregulation of the hypoxia markers, GLUT1 and VEGF-A, in cells located near the device. Use of the iNANIVID will thus enable investigation of the effects of local hypoxic microenvironments in vivo on tumor cell metastasis from different regions of the primary tumor.
The potential use of the iNANIVID is not limited to EGF and hypoxia mimetics, however. All of the agents introduced into solid tissue using the in vivo invasion assay can also be introduced using the iNANIVID. These include chemoattractants (e.g., colony stimulating factor-1 to attract the co-migratory macrophage population from the tumor), various cytokines (e.g. VEGF to induce angiogenesis), therapeutics (e.g., doxorubicin to induce local cell death and observe immune responses), function blocking antibodies (e.g. anti- CSF-1 to block macrophage function) or chemical agents (e.g., L-ascorbic acid to alter the extracellular matrix composition). We have demonstrated the utility of the iNANIVID by recapitulating naturally occurring EGF gradientsCitation49 in metastatic tumors. The ability to experimentally recapitulate gradients, and image their responses in vivo, extends to many other physiological processes where such gradients are naturally found including all other solid tumors accessible to intravital imaging,Citation50 wound healing,Citation51 infection,Citation52 embryogenesis,Citation53 and many others.Citation45,54
For spatial and temporal control of gradient formation, one could modify the hydrogel chemistry to alter the kinetics of factor release. Multi-factor gradients can be produced by including additional factors in the hydrogel mixture. Longer time durations can be achieved by combining the iNANIVID with implantable imaging windows for serial studies either by insertion of the device under the window or by integrating the microfluidics into the window to provide surface application of the factors. Work toward this latter application is currently underway. While the current iteration forms a gradient near the surface of the tumor, other iNANIVID designs provide access to different regions of the tumor. The flexibility of the iNANIVID design will provide additional avenues of investigation and further insight into the many signals involved in the interactions of tumor cells with the various microenvironments that define tumor phenotype at single cell resolution.
Materials and methods
Device fabrication
The iNANIVID was fabricated using methods similar to those described previously.Citation18 Briefly, 10 nm of Cr followed by 100 nm of Au were deposited onto two glass substrates through e-beam evaporation. Photolithography was used to pattern the hydrogel/cell collection chamber of the device reservoir and fluorescent bead wells of the device cover. These features were isotropically etched into the glass using 10:1 hydrofluoric acid:hydrochloric acid. Photoresist and metals were removed using acetone, Au etchant, and Cr etchant. Substrates were cleaned using 3:1 sulfuric acid:hydrogen peroxide and diced into the desired shape. Fluorescent polystyrene microspheres (Life Technologies, Carlsbad, CA, USA) were placed in the bead wells and sealed inside with a thin layer of polydimethylsiloxane (Dow Corning, Midland, MI, USA). The NANIVID was next loaded with a hydrogel solution consisting of polyethylene glycol diacrylate (Glycosan, Salt Lake City, UT, USA), methoxypolyethylene glycol monoacrylate (Sartomer, Exton, PA, USA), Irgacure2959 (BASF, Florham Park, NJ, USA) and a factor of interest, i.e. EGF (Sigma-Aldrich, St. Louis, MO, USA). Hydrogel components were mixed with a UV photoinitiator in order to crosslink the hydrogel upon exposure to UV light. A small volume of the mixture was drawn up between the tips of fine tweezers and dispensed in the hydrogel chamber by opening the tweezers. The solution was dispensed until the back half of the chamber (demarcated by the “anchor points” etched into the sidewall of the chamber, see Fig. S1) was filled: approximately 3.15 nL. The hydrogel was then crosslinked by brief UV exposure and the cover placed on top to seal the device. Devices were stored at −20 C until the time of the experiment.
For the experiment the device was placed in a dish of 1 mg/ml Matrigel in PBS, on ice. The dish was transferred to a desiccator chamber and vacuum applied to both draw air bubbles out of the porous hydrogel and fill the device with matrigel upon return to atmospheric pressure. The device was placed in PBS at 37 C until implantation in the tumor. The cover and reservoir were activated in oxygen plasma and sealed together to create the final device. Devices were stored at −20 C until the time of experiment.
Mouse models
All procedures were conducted in accordance with the National Institutes of Health regulations and approved by the Albert Einstein College of Medicine and Mount Sinai School of Medicine animal use committees. For the MDA-MB-231 xenografts, a total of 2 × 106 MDA-MB-231-dendra2 cells per animal were resuspended in sterile PBS with 20% collagen I (BD Biosciences, Franklin Lakes, NJ, USA) and injected into the inguinal mammary fat pad of SCID mice (NCI, Frederick, MD, USA). All experiments were performed on tumors that were 1 to 1.2 cm in diameter.
Fixturing
After a standard skin flap surgery is performed to expose the mammary tumor, a piece of plastic-backed foam is attached to the skin behind the tumor using surgical staples to provide stiffness and anchor points for fixturing. The tumor is placed through a hole cut in a piece of soft rubber (40 Duro hardness) of similar thickness to prevent compression of the tissue. Two additional stiff rubber substrates (Shore 70A) are placed on top of the foam backing and taped to a custom-built microscope stage adapter to stabilize the specimen. The top of the stage adapter features a hole through which the tumor is placed and the bottom has a recess for a removable microscope coverglass which is then fixed in place with an o-ring to prevent water loss. Suitable regions with high fluorescence intensity are identified by imaging the tumor on the microscope. To provide access to the tumor, the stage adapter and cover glass are removed, and the iNANIVID is implanted using the insertion tool. The cover glass is replaced and the stage adapter is returned to the microscope for timelapse imaging.
in vivo cell migration assays
Mice are anesthetized with 1.5–3% isoflurane in O2. The tumor area is wiped with 70% ethanol and an incision is made to expose the tumor. Fixturing is performed by the aforementioned protocol. Matrigel is diluted in ice cold PBS to a final concentration of 1.0 mg/ mL and degassed for 10 minutes on ice. For the migration experiments, 2 µM EGF loaded iNANIVIDs is degassed in matrigel solution on ice for 1 minute. Devices are removed from the matrigel solution and placed in 37 C PBS. Regions of high fluorescence intensity are located using epi-fluorescence mode on the microscope. The coverslip is removed from the stage insert and each iNANIVID is implanted into the tumor using the insertion device. The outlet of the implanted iNANIVID is located by the fluorescent polystyrene beads inside the device. Dendra2 photoconversion is performed on cells located approximately 150 µm away from the device using a 405 nm diode. Time lapse multiphoton microscopy was performed with a frame rate of approximately one stack every 3 minutes for 4 hours, to obtain a 75 µm Z-stack composed of 5 µm steps. Continuous time lapse imaging of the region in front of the outlet is performed for 4 hours. Control assays are performed under the same protocol using an iNANIVID containing hydrogel without EGF.
Microscope setup
Multiphoton microscopy is performed using a custom-built multi-channel two-laser microscope previously reported.Citation55 The microscope, based upon an inverted Olympus IX71 stand, features two femtosecond pulsed lasers for simultaneous excitation of both the green and red forms of Dendra2. All fluorescence signals (SHG, Dendra2 green and Dendra2 red) were cleanly separable in multiple simultaneously acquiring detectors. For this work, we have additionally modified the system by the addition of a high power 405 nm diode laser (Omicron, PhoxX 405–60) coupled into the system through the same port as the femtosecond pulsed beam. The field of view illuminated by the photoconversion laser is limited by placing a mask in the conjugate plane located between the scan and tube lenses. Photoconversion is accomplished using ∼4.5 mW of 405 nm light at a scan rate of 0.4 fps.
Cell migration analysis
Time lapse image data was converted into a 4D hyperstack using ImageJ software. Minor residual drift in the XY directions was eliminated through use of the StackReg pluginCitation56 for ImageJ.Citation57 Channel subtraction was performed to improve image color separation. Time lapses were rotated for clarity or cropped to highlight areas of interest. Cell tracking was performed using the ROI_Tracker plugin.Citation55 Cells that moved at least one cell length over the time course were manually traced to track cell movement. Cell centroid data was exported for analysis of cell migration parameters.
Hypoxia induction
Since in vivo imaging was not performed for the hypoxia experiments, an alternative iNANIVID design featuring a longer tail was utilized so as to more readily facilitate handling of the device. This device was fabricated using the same aforementioned methods but did not require the plunger for implantation and their openings were located deeper within the solid tumors. Devices were loaded with either 2 mM Cobalt Chloride (CoCl2), 1 mM Deferoxamine (DFOM), or PBS as a control.
iNANIVID implantation in HEp3 tumors grown on CAMs
The chicken chorioallantoic membrane model has been previously described.Citation58 We used premium specific pathogen-free (SPF), fertile, E8 day incubated chicken embryos supplied by Charles River Laboratories, Inc. For the iNANIVID implantation, a layer of minced T-HEp3 tumor fragments were seeded inside of a Teflon® ring on the chorioallantoic membrane of an E10 embryo as described previously.Citation59 In this ring, on the first layer of tumor cells, the iNANIVID was implanted manually in the middle of the ring with the opening of the iNANIVID to the left. This allows diffusion to occur leftwards primarily. Then a second layer of minced T-HEp3 tumor fragmets were added to cover the iNANIVID completely. The Teflon® ring kept the minced tumor pieces in place. Embryos were implanted with DFOM (n = 5), CoCl2 (n = 5), or PBS (n = 5) - containing iNANIVIDs. Following tumor implantation, the embryos were incubated for 3 days at 37 C which enables tumor growth and encapsulation of the iNANIVID. Subsequently, the upper left quadrant of the tumor, where the opening of the iNANIVID was placed or a region in the lower left quadrant distal to the opening, were harvested for processing.
Histology and immunofluorescence (IF) assays
Paraffin embedded sections of the T-HEp3 CAM tumors were stained with hematoxylin and eosin to determine the overall integrity of the tissue after implantation of the iNANIVID devices. Paraffin embedded sections of T-HEp3 CAM tumors were stained with an antibody for Glut1 (used at 1:100; #07-1401, Millipore, USA). Sections were blocked with 3% normal goat serum (NGS; #PCN5000, Invitrogen, USA) in PBS prior to the first antibody. Antibody binding was carried out at 4C overnight. For detection, a secondary Alexa-568 antibody reacting to the primary was applied for 1 hour in the dark at room temperature (used at 1:1000; #A-11011, Invitrogen, USA). The sections were mounted in ProLong® Gold Antifade with DAPI (#P-36931, LifeTechnologies, USA) to detect the DNA in the cells nuclei. Sections were evaluated and pictures taken using a Leica DM 5500 B fluorescence microscope with Leica DCF 400 camera or a Nikon Eclipse Ti-S fluorescence microscope with SPOT RT-KE/SE camera with the corresponding manufacturers' software.
Quantitative PCR (qPCR)
Whole RNA was isolated from T-HEp3 CAM tumors influenced by the PBS, CoCl2 or DFOM iNANIVIDs using TRIzol® reagent (#15596-026, Invitrogen, USA) according to the manufacturer's instructions. Quantitative qPCR for GLUT1 and VEGF-A were performed as described previously.Citation60 GAPDH was used for normalization.
Vascular damage assessment
FVB mice bearing syngeneic tumor Polyoma Middle-T transplants were grown until the tumors were approximately 0.5 cm in diameter. Devices were prepared as above and loaded with hydrogel only. Blood flow was evaluated by inserting the device into the tumor followed by an intravenous injection of high-molecular weight dextran (500 SkD Fluorescein isothiocyanate–dextran, Sigma-Aldrich, St. Louis, MO, USA) which ensures that no significant leakage out of the vessels will occur. Epi-fluorescent stereoscope (SZX16, Olympus) imaging of the vasculature around the device was then performed.
Collagen damage assessment
Second Harmonic Generation Images of the device entrances in 16 different mice were analyzed and distances between the edge of the device and the end of the damaged collagen (assessed as either broken or bunched fibers) were measured in ImageJ.
Gradient imaging
FVB mice bearing syngeneic tumor Polyoma Middle-T transplants were grown until the tumors were approximately 0.5 cm in diameter. Devices were prepared as above and loaded with 15 µM EGF labeled with Fluorescein (Thermo Fisher, Waltham, MA). Tumors of anesthetized mice were exposed using a skin flap surgery and devices were implanted into tumors of anesthetized mice. Z-stack (125 µm in 12.5 µm steps) intravital images of the FITC-EGF gradient at the outlet was acquired every 2–2.5 min for 4.5 hours. Stacks were average intensity projected and a 100 × 375 µm area in front of the outlet line averaged to produce line plots. The peak value of each time point was then normalized to the value of the highest peak time point of the series and plotted.
Statistical analysis
All statistical analysis was carried out using the 770 GraphPad Prism software version 5.0d. For the cell migration analysis, One-Way ANOVA tests were performed; a p-value of ≤0.05 was considered significant. For the hypoxia gene analysis, a Non-parametric Mann-Whitney test was used to calculate the significance in differences between groups; a one-tailed p-value of ≤0.05 was considered significant.
Disclosure of potential conflicts of interest
No potential conflicts of interest were disclosed.
Supplemental_Captions.docx
Download MS Word (12.7 KB)Figure_S1.tif
Download TIFF Image (510.2 KB)Figure_S2.pdf
Download PDF (32.4 KB)Supplemental_Movie_1.wmv
Download (2.7 MB)Supplemental_Movie_2.wmv
Download (2.5 MB)Supplemental_Movie_3.wmv
Download (6.3 MB)Supplemental_Movie_4.wmv
Download (809.6 KB)Supplemental_Movie_5.wmv
Download (3.2 MB)Acknowledgments
The authors thank Mike Rottenkolber, Ricardo Ibagon and Anthony Leggiadro of the Einstein machine shop for their skilled and timely craftsmanship and Patricia Keely and Kevin Eliceiri of the University of Wisconsin-Madison for informative discussions and guidance.
Funding
This research was supported by the Tumor Microenvironment Network center grant CA163131 for J.C, J.A.A-G. and J.S.C., the Einstein Integrated Imaging Program for D.E. and the Samuel Waxman Cancer Research Foundation Tumor Dormancy Program and NIH/National Cancer Institute CA109182 for J.A.A-G.
References
- Mehlen P, Puisieux A. Metastasis: a question of life or death. Nat Rev Cancer 2006; 6:449-58; PMID:16723991; http://dx.doi.org/10.1038/nrc1886
- Schardt JA, Meyer M, Hartmann CH, Schubert F, Schmidt-Kittler O, Fuhrmann C, Polzer B, Petronio M, Eils R, Klein CA. Genomic analysis of single cytokeratin-positive cells from bone marrow reveals early mutational events in breast cancer. Cancer Cell 2005; 8:227-39; PMID:16169467; http://dx.doi.org/10.1016/j.ccr.2005.08.003
- Weigelt B, Peterse JL, van 't Veer LJ. Breast cancer metastasis: markers and models. Nat Rev Cancer 2005; 5:591-602; PMID:16056258; http://dx.doi.org/10.1038/nrc1670
- Wang W, Goswami S, Sahai E, Wyckoff JB, Segall JE, Condeelis JS. Tumor cells caught in the act of invading: their strategy for enhanced cell motility. Trends Cell Biol 2005; 15:138-45; PMID:15752977; http://dx.doi.org/10.1016/j.tcb.2005.01.003
- Hanahan D, Weinberg RA. Hallmarks of cancer: the next generation. Cell 2011; 144:646-74; PMID:21376230; http://dx.doi.org/10.1016/j.cell.2011.02.013
- Quail DF, Joyce JA. Microenvironmental regulation of tumor progression and metastasis. Nat Med 2013; 19:1423-37; PMID:24202395; http://dx.doi.org/10.1038/nm.3394
- Zaman MH. The role of engineering approaches in analysing cancer invasion and metastasis. Nat Rev Cancer 2013; 13:596-603; PMID:23864050; http://dx.doi.org/10.1038/nrc3564
- Bravo-Cordero JJ, Hodgson L, Condeelis J. Directed cell invasion and migration during metastasis. Curr Opin Cell Biol 2012; 24:277-83; PMID:22209238; http://dx.doi.org/10.1016/j.ceb.2011.12.004
- Toetsch S, Olwell P, Prina-Mello A, Volkov Y. The evolution of chemotaxis assays from static models to physiologically relevant platforms. Integrative Biol 2009; 1:170-81; http://dx.doi.org/10.1039/B814567A
- Kim JB, Stein R, O'Hare MJ. Three-dimensional in vitro tissue culture models of breast cancer– a review. Breast Cancer Res Treat 2004; 85:281-91; PMID:15111767; http://dx.doi.org/10.1023/B:BREA.0000025418.88785.2b
- Goswami S, Sahai E, Wyckoff JB, Cammer M, Cox D, Pixley FJ, Stanley ER, Segall JE, Condeelis JS. Macrophages promote the invasion of breast carcinoma cells via a colony-stimulating factor-1/epidermal growth factor paracrine loop. Cancer Res 2005; 65:5278-83; PMID:15958574; http://dx.doi.org/10.1158/0008-5472.CAN-04-1853
- Nakasone ES, Askautrud HA, Kees T, Park JH, Plaks V, Ewald AJ, Fein M, Rasch MG, Tan YX, Qiu J, et al. Imaging tumor-stroma interactions during chemotherapy reveals contributions of the microenvironment to resistance. Cancer Cell 2012; 21:488-503; PMID:22516258; http://dx.doi.org/10.1016/j.ccr.2012.02.017
- Ahn GO, Tseng D, Liao CH, Dorie MJ, Czechowicz A, Brown JM. Inhibition of Mac-1 (CD11b/CD18) enhances tumor response to radiation by reducing myeloid cell recruitment. Proc Natl Acad Sci U S A 2010; 107:8363-8; PMID:20404138; http://dx.doi.org/10.1073/pnas.0911378107
- Burnett SH, Kershen EJ, Zhang J, Zeng L, Straley SC, Kaplan AM, Cohen DA. Conditional macrophage ablation in transgenic mice expressing a Fas-based suicide gene. J Leukoc Biol 2004; 75:612-23; PMID:14726498; http://dx.doi.org/10.1189/jlb.0903442
- Wyckoff JB, Segall JE, Condeelis JS. The collection of the motile population of cells from a living tumor. Cancer Res 2000; 60:5401-4; PMID:11034079
- Gligorijevic B, Bergman A, Condeelis J. Multiparametric classification links tumor microenvironments with tumor cell phenotype. PLoS Biol 2014; 12:e1001995; PMID:25386698; http://dx.doi.org/10.1371/journal.pbio.1001995
- Patsialou A, Bravo-Cordero JJ, Wang Y, Entenberg D, Liu H, Clarke M, Condeelis JS. Intravital multiphoton imaging reveals multicellular streaming as a crucial component of in vivo cell migration in human breast tumors. Intravital 2013; 2:e25294; PMID:25013744; http://dx.doi.org/10.4161/intv.25294
- Raja WK, Gligorijevic B, Wyckoff J, Condeelis JS, Castracane J. A new chemotaxis device for cell migration studies. Integr Biol (Camb.) 2010; 2:696-706; PMID:20938544; http://dx.doi.org/10.1039/c0ib00044b
- Zipfel WR, Williams RM, Webb WW. Nonlinear magic: multiphoton microscopy in the biosciences. Nat Biotechnol 2003; 21:1369-77; PMID:14595365; http://dx.doi.org/10.1038/nbt899
- Gurskaya NG, Verkhusha VV, Shcheglov AS, Staroverov DB, Chepurnykh TV, Fradkov AF, Lukyanov S, Lukyanov KA. Engineering of a monomeric green-to-red photoactivatable fluorescent protein induced by blue light. Nat Biotechnol 2006; 24:461-5; PMID:16550175; http://dx.doi.org/10.1038/nbt1191
- Petrie RJ, Doyle AD, Yamada KM. Random vs. directionally persistent cell migration. Nat Rev Mol Cell Biol 2009; 10:538-49; PMID:19603038; http://dx.doi.org/10.1038/nrm2729
- Kay RR, Langridge P, Traynor D, Hoeller O. Changing directions in the study of chemotaxis. Nat Rev Mol Cell Biol 2008; 9:455-63; PMID:18500256; http://dx.doi.org/10.1038/nrm2419-c2
- Guo M, Song LP, Jiang Y, Liu W, Yu Y, Chen GQ. Hypoxia-mimetic agents desferrioxamine and cobalt chloride induce leukemic cell apoptosis through different hypoxia-inducible factor-1alpha independent mechanisms. Apoptosis 2006; 11:67-77; PMID:16374551; http://dx.doi.org/10.1007/s10495-005-3085-3
- Aguirre-Ghiso JA, Ossowski L, Rosenbaum SK. Green fluorescent protein tagging of extracellular signal-regulated kinase and p38 pathways reveals novel dynamics of pathway activation during primary and metastatic growth. Cancer Res 2004; 64:7336-45; PMID:15492254; http://dx.doi.org/10.1158/0008-5472.CAN-04-0113
- Hoskin PJ, Sibtain A, Daley FM, Wilson GD. GLUT1 and CAIX as intrinsic markers of hypoxia in bladder cancer: relationship with vascularity and proliferation as predictors of outcome of ARCON. Br J Cancer 2003; 89:1290-7; PMID:14520462; http://dx.doi.org/10.1038/sj.bjc.6601260
- Liu XH, Kirschenbaum A, Yao S, Stearns ME, Holland JF, Claffey K, Levine AC. Upregulation of vascular endothelial growth factor by cobalt chloride-simulated hypoxia is mediated by persistent induction of cyclooxygenase-2 in a metastatic human prostate cancer cell line. Clin Exp Metastasis 1999; 17:687-94; PMID:10919714; http://dx.doi.org/10.1023/A:1006728119549
- Goldberg MA, Schneider TJ. Similarities between the oxygen-sensing mechanisms regulating the expression of vascular endothelial growth factor and erythropoietin. J Biol Chem 1994; 269:4355-9; PMID:8308005
- Egeblad M, Nakasone ES, Werb Z. Tumors as organs: complex tissues that interface with the entire organism. Dev Cell 2010; 18:884-901; PMID:20627072; http://dx.doi.org/10.1016/j.devcel.2010.05.012
- Hanahan D, Coussens LM. Accessories to the crime: functions of cells recruited to the tumor microenvironment. Cancer Cell 2012; 21:309-22; PMID:22439926; http://dx.doi.org/10.1016/j.ccr.2012.02.022
- Yamaguchi H, Wyckoff J, Condeelis J. Cell migration in tumors. Curr Opin Cell Biol 2005; 17:559-64; PMID:16098726; http://dx.doi.org/10.1016/j.ceb.2005.08.002
- Arihiro K, Oda H, Kaneko M, Inai K. Cytokines facilitate chemotactic motility of breast carcinoma cells. Breast Cancer 2000; 7:221-30; PMID:11029802; http://dx.doi.org/10.1007/BF02967464
- Helmlinger G, Yuan F, Dellian M, Jain RK. Interstitial pH and pO2 gradients in solid tumors in vivo: high-resolution measurements reveal a lack of correlation. Nat Med 1997; 3:177-82; PMID:9018236; http://dx.doi.org/10.1038/nm0297-177
- Mott JD, Werb Z. Regulation of matrix biology by matrix metalloproteinases. Curr Opin Cell Biol 2004; 16:558-64; PMID:15363807; http://dx.doi.org/10.1016/j.ceb.2004.07.010
- Condeelis J, Segall JE. Intravital imaging of cell movement in tumours. Nat Rev Cancer 2003; 3:921-30; PMID:14737122; http://dx.doi.org/10.1038/nrc1231
- Zhou ZN, Boimel PJ, Segall JE. Tumor-stroma: In vivo assays and intravital imaging to study cell migration and metastasis. Drug Discov Today Dis Models 2011; 8:95-112; PMID:22081771; http://dx.doi.org/10.1016/j.ddmod.2011.07.003
- Friedl P, Brocker EB. The biology of cell locomotion within three-dimensional extracellular matrix. Cell Mol Life Sci 2000; 57:41-64; PMID:10949580; http://dx.doi.org/10.1007/s000180050498
- Simpson KJ, Selfors LM, Bui J, Reynolds A, Leake D, Khvorova A, Brugge JS. Identification of genes that regulate epithelial cell migration using an siRNA screening approach. Nat Cell Biol 2008; 10:1027-38; PMID:19160483; http://dx.doi.org/10.1038/ncb1762
- Soon L, Mouneimne G, Segall J, Wyckoff J, Condeelis J. Description and characterization of a chamber for viewing and quantifying cancer cell chemotaxis. Cell Motil Cytoskeleton 2005; 62:27-34; PMID:16025469; http://dx.doi.org/10.1002/cm.20082
- Mouneimne G, DesMarais V, Sidani M, Scemes E, Wang W, Song X, Eddy R, Condeelis J. Spatial and temporal control of cofilin activity is required for directional sensing during chemotaxis. Curr Biol 2006; 16:2193-205; PMID:17113383; http://dx.doi.org/10.1016/j.cub.2006.09.016
- Magalhaes MA, Larson DR, Mader CC, Bravo-Cordero JJ, Gil-Henn H, Oser M, Chen X, Koleske AJ, Condeelis J. Cortactin phosphorylation regulates cell invasion through a pH-dependent pathway. J Cell Biol 2011; 195:903-20; PMID:22105349; http://dx.doi.org/10.1083/jcb.201103045
- Sharma VP, Beaty BT, Patsialou A, Liu H, Clarke M, Cox D, Condeelis JS, Eddy RJ. Reconstitution of in vivo macrophage-tumor cell pairing and streaming motility on one-dimensional micro-patterned substrates. Intravital 2012; 1:77-85; PMID:24634804; http://dx.doi.org/10.4161/intv.22054
- Krysko DV, Kaczmarek A, Krysko O, Heyndrickx L, Woznicki J, Bogaert P, Cauwels A, Takahashi N, Magez S, Bachert C, et al. TLR-2 and TLR-9 are sensors of apoptosis in a mouse model of doxorubicin-induced acute inflammation. Cell Death Differentiation 2011; 18:1316-25; PMID:21311566; http://dx.doi.org/10.1038/cdd.2011.4
- Wang W, Goswami S, Lapidus K, Wells AL, Wyckoff JB, Sahai E, Singer RH, Segall JE, Condeelis JS. Identification and testing of a gene expression signature of invasive carcinoma cells within primary mammary tumors. Cancer Res 2004; 64:8585-94; PMID:15574765; http://dx.doi.org/10.1158/0008-5472.CAN-04-1136
- Patsialou A, Wang Y, Lin J, Whitney K, Goswami S, Kenny PA, Condeelis JS. Selective gene-expression profiling of migratory tumor cells in vivo predicts clinical outcome in breast cancer patients. Breast Cancer Res 2012; 14:R139; PMID:23113900; http://dx.doi.org/10.1186/bcr3344
- Roussos ET, Condeelis JS, Patsialou A. Chemotaxis in cancer. Nat Rev Cancer 2011; 11:573-87; PMID:21779009; http://dx.doi.org/10.1038/nrc3078
- Wyckoff J, Wang W, Lin EY, Wang Y, Pixley F, Stanley ER, Graf T, Pollard JW, Segall J, Condeelis J. A paracrine loop between tumor cells and macrophages is required for tumor cell migration in mammary tumors. Cancer Res 2004; 64:7022-9; PMID:15466195; http://dx.doi.org/10.1158/0008-5472.CAN-04-1449
- Harney AS, Arwert EN, Entenberg D, Wang Y, Guo P, Qian BZ, Oktay MH, Pollard JW, Jones JG, Condeelis JS. Real-Time imaging reveals local, transient vascular permeability, and tumor cell intravasation stimulated by TIE2hi Macrophage-Derived VEGFA. Cancer Discov 2015; 5(9):932-43; PMID:26269515; http://dx.doi.org/10.1158/2159-8290.CD-15-0012
- Wilson WR, Hay MP. Targeting hypoxia in cancer therapy. Nat Rev Cancer 2011; 11:393-410; PMID:21606941; http://dx.doi.org/10.1038/nrc3064
- Desmarais V, Yamaguchi H, Oser M, Soon L, Mouneimne G, Sarmiento C, Eddy R, Condeelis J. N-WASP and cortactin are involved in invadopodium-dependent chemotaxis to EGF in breast tumor cells. Cell Motil Cytoskeleton 2009; 66:303-16; PMID:19373774; http://dx.doi.org/10.1002/cm.20361
- Shields JD, Fleury ME, Yong C, Tomei AA, Randolph GJ, Swartz MA. Autologous chemotaxis as a mechanism of tumor cell homing to lymphatics via interstitial flow and autocrine CCR7 signaling. Cancer Cell 2007; 11:526-38; PMID:17560334; http://dx.doi.org/10.1016/j.ccr.2007.04.020
- Farooqui R, Fenteany G. Multiple rows of cells behind an epithelial wound edge extend cryptic lamellipodia to collectively drive cell-sheet movement. J. Cell Sci 2005; 118:51-63; PMID:15585576; http://dx.doi.org/10.1242/jcs.01577
- Osma-Garcia IC, Punzon C, Fresno M, Diaz-Munoz M. D. Dose-dependent effects of prostaglandin E2 in macrophage adhesion and migration. Eur J Immunol 2016; 46:677-88; PMID:26631603; http://dx.doi.org/10.1002/eji.201545629
- Tabata T, Takei Y. Morphogens, their identification and regulation. Development 2004; 131:703-12; PMID:14757636; http://dx.doi.org/10.1242/dev.01043
- Jin T, Xu X, Hereld D. Chemotaxis, chemokine receptors and human disease. Cytokine 2008; 44:1-8; PMID:18722135; http://dx.doi.org/10.1016/j.cyto.2008.06.017
- Entenberg D, Wyckoff J, Gligorijevic B, Roussos ET, Verkhusha VV, Pollard JW, Condeelis J. Setup and use of a two-laser multiphoton microscope for multichannel intravital fluorescence imaging. Nat Protoc 2011; 6:1500-20; PMID:21959234; http://dx.doi.org/10.1038/nprot.2011.376
- Thevenaz P, Ruttimann UE, Unser M. A pyramid approach to subpixel registration based on intensity. IEEE Trans Image Process 1998; 7:27-41; PMID:18267377; http://dx.doi.org/10.1109/83.650848
- Schneider CA, Rasband WS, Eliceiri KW. NIH Image to ImageJ: 25 years of image analysis. Nat Methods 2012; 9:671-5; PMID:22930834; http://dx.doi.org/10.1038/nmeth.2089
- Ghiso JAA, Kovalski K, Ossowski L. Tumor dormancy induced by downregulation of urokinase receptor in human carcinoma involves integrin and MAPK signaling. J Cell Biol 1999; 147:89-103; PMID:10508858; http://dx.doi.org/10.1083/jcb.147.1.89
- Aguirre-Ghiso JA, Liu D, Mignatti A, Kovalski K, Ossowski L. Urokinase receptor and fibronectin regulate the ERKMAPK to p38(MAPK) activity ratios that determine carcinoma cell proliferation or dormancy in vivo. Mol Biol Cell 2001; 12:863-79; PMID:11294892; http://dx.doi.org/10.1091/mbc.12.4.863
- Adam AP, George A, Schewe D, Bragado P, Iglesias BV, Ranganathan AC, Kourtidis A, Conklin DS, Aguirre-Ghiso JA. Computational identification of a p38SAPK-regulated transcription factor network required for tumor cell quiescence. Cancer Res 2009; 69:5664-72; PMID:19584293; http://dx.doi.org/10.1158/0008-5472.CAN-08-3820