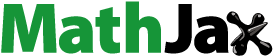
Abstract
In this study, non-toxic mercury nanoparticle was synthesized as per “Prakash theory of metal drugs” and nanoparticle’s characters has been demonstrated by employing several nanotechnological tools including XPS, XRD, EDAX. The size of the Prakasine nanoparticles (PRK-NP) ranged from 90–100 nm, confirmed using TEM, SEM, DLS and along with zeta potential of −29.5 mV before storage and −8.5 mV after storage. The FTIR provided information regarding the nanoparticle capping and functional groups. The study was further elaborated for determining PRK-NPs toxicity, genotoxicity, in-vivo toxicity, immunological anti-tumour activity, immunogenicity potential, gene expression profiling and confirmed by MTT and apoptosis assays, cancer zebrafish model studies and WBC proliferation assay. PRK-NPs revealed no cytotoxicity where cell viability was observed 99% in L6 mouse fibroblasts and 99% in MCF-7 cell lines. Also, the cell viability was to be 89.47% at a very high concentration of 320 µg/ml in HEK 293 cells. The PRK-NPs significantly reduced the tumour in zebrafish at dose of 90 μg/g by up regulating IL-1α, IL-1β, IL-2-ITK, IL-6, IL-8, IL-12, TNF-α and IFN-γ, and down regulating IL-4, IL-5, IL-10 and TGF-β compared to untreated controls without any adverse effects and toxicity. Thus, the current study beholds anticipation PRK-NPs may play a vital role in therapeutic.
Introduction
Total cancer burden is increasing in almost every region of the globe now [Citation1,Citation2], and current chemotherapies, radiotherapies, and surgeries often fail to eliminate the cancer permanently or are intolerable due to poor discrimination between healthy and cancerous tissues [Citation3]. There is currently a major focus on immunotherapies that harness endogenous inflammatory pathways against tumour tissue. Tumour growth may be arrested by locally elevating proinflammatory cytokines that activate natural killer (NK) cells and cytotoxic T-lymphocytes (CTLs). An ideal immunotherapy would activate proinflammatory cytokine signaling networks, increase immune cell populations, and selectively promote tumour infiltration.
Nanotechnology is a promising strategy for directly targeting such immunotherapies to tumour cells without exposing healthy cells. Nanoparticles (NPs) are promising vectors for anticancer therapeutics as they can be tailored with multiple advantageous physicochemical and pharmacological properties, such as biocompatibility for normal tissue, efficient tumour targeting, controlled activation, and biodegradability [Citation4].For instance, NPs can interact with cells and proteins to stimulate the innate immune response, cell-mediated immune response, humoral immune response, and the complement system [Citation5].
The engineering of nanostructured materials, including NPs, and successful applications in the field of immunology will facilitate the development of novel vaccines, adjuvants, and immunomodulatory drugs to improve clinical outcomes of infectious and non-infectious diseases [Citation6]. Nanostructured materials can also control stem cell behaviour in vitro and in vivo [Citation7]. Several NP-based strategies show particular promise for cancer treatment, including stimulation of innate immunity, induction of protective immunity against tumorigenesis from viral infection, and recruitment of CTLs to potentiate immunotherapy in the tumour microenvironment [Citation5,Citation8–16].
The zebrafish is gaining acceptance equal to mouse models and cell lines for cancer drug research and NP toxicity studies [Citation17,Citation18] owing to relatively inexpensive maintenance, rapid breeding, and amenability to certain genetic and microscopy techniques [Citation17]. Indeed, several studies have examined NP toxicity, efficacy of drug delivery, and effects on gene expression using zebrafish [Citation19–21]. Zebrafish are also used to assess stem cell function and for drug discovery [Citation22]. Furthermore, doctors often use zebrafish models to determine which cancer treatment works best for a particular patient [Citation23]. Despite extensive studies on the use of nanotubes, nanoclusters, nanocapsules, nanobubbles, nanocarriers, and nanorods for oncolytic virus therapies, cancer vaccines, cytokine therapies, adoptive cell transfer, and immune checkpoint inhibition, there is still no broadly efficacious NP-based cancer immunotherapy.
In a previous study [Citation24], this compound produced adaptive passive humoral immunity in birds against Newcastle disease virus without adverse effects. In the current study, the nanoparticle would be characterized, the cytotoxicity would be assessed in the cell lines, apoptosis would be analyzed by fluorescence activated cell sorting (FACS), the immunomodulatory and anticancer effects of PRK-NP would be examined in a zebrafish tumour model with a suitable design, the gene expression profile would be assessed using polymerase chain reaction (PCR), qPCR, and ELISA, and finally, the results would be compared with those of existing standard anticancerous drugs for the comparative immunotherapy efficacy of Prakasine for cancer treatment.
Material and methods
Preparation of prakasine nanoparticles
Prakasine (PRK-NP) nanoparticle was prepared with processed mercury, processed sulphur, and methionine (ligand) as mentioned in Indian Patent Application No. 5776/CHE/2014 and as reported previously [Citation24]. Briefly, the nanoparticle was synthesised by top down solid state method and employed for this present study. PRK-NP was subjected to characterisation, in vitro and in vivo experimentations for toxicity, chemicophysical properties, and bioactivities. All procedures involving zebrafish were reviewed and approved by the Pentagrit Institutional Animal Ethics Committee (Approval no: 209/Go02/IAEC) and all methods were performed according to relevant national guidelines and regulations.
Compositional analysis by X-ray photoelectron spectroscopy (XPS)
X-ray photoelectron spectra were obtained using an ESCA + Omicron Nanotechnology ESCA Probe spectrometer (Scienta Omicron, Germany) with monochromatized aluminium K-alpha X-rays (energy: 1486.6 eV). The procedure was essentially as described in several previous studies [Citation25–28]. Briefly, the X-ray power applied was 300 W, and the pass energy was 50 eV for survey scans and 20 eV for narrower specific regions. The sample solution was spotted on a molybdenum sample plate and dried in a vacuum. Spectra in the required binding energy ranges were collected and an average spectrum was constructed. Each spectrum was confirmed by replications (except the survey scan, which was performed only once). The scan rate (steps per second) was the same for all narrow scans. Beam-induced damage to the sample was reduced by adjusting the X-ray flux. The base pressure of the instrument was 5.0 × 10 − 10 mB.
X-Ray diffraction (XRD) analysis of crystalline structure
XRD analysis of dried PRK-NP powder was performed on a LabX XRD-6000 system (Shimadu, Kyoto, Japan) using a monochromatized X-ray beam with nickel-filtered CuKα radiation (λ = 1.5418 Å) at 40 kV. A continuous scan mode was used to collect 2θ data as described previously [Citation29]. XRD was performed to identify the arrangement of atoms in the nanoparticle and grain size by interpreting XRD peaks and intensities.
Functional group characterization by Fourier-transform infra-red (FTIR) spectroscopy
Fourier-transform IR spectra were acquired using a Perkin Elmer Spectrum One instrument by applying the protocols mentioned elsewhere [Citation30]. Briefly, the KBr crystals were used as the matrix for sample preparation with PRK-NP. The samples were prepared by depositing the nanoparticle suspension on KBr pellets which were then subjected to a drying process (using a UV lamp) before recording the spectra. The spectra ranged from 4000 to 400 cm−1 with a resolution of 2 cm−1. The spectrum and peaks were examined and interpreted for the presence of organic elements, functional groups, stretches, wag, and chemical composition.
Elemental analyses by energy dispersive spectroscopy (EDS) and scanning electron microscopy (SEM)
Energy dispersive spectra and scanning electron micrographs were obtained by an X system (Oxford Instruments, Abingdon, UK) attached to a JMS-6390 scanning electron microscope (JEOL, Tokyo, Japan) as per previously described protocol [Citation29]. Briefly, samples were prepared by dispersing properly in ethanol as suspension by ultrosonication, the adhesive and substrate are placed over the SEM stub one after another respectively, finally, a droplet of suspension was placed on the substrate and air dried for SEM examination and data were acquired.
Nanoparticle morphology analyses by transmission electron microscopy (TEM)
The shape, size, and surface characteristics of synthesized PRK-NPs were examined by a High-Resolution Transmission Electron Microscope (Tecnai 12, Philips, Amsterdam, The Netherlands) at 200 kV acceleration voltage. Sample preparation and image acquisition were conducted as previously described [Citation31]. Briefly, samples were prepared by dispersing properly in ethanol. This nano particle mixed solution was sonicated for few minutes and drop-coating of 10 μL PRK-NP sonicated solution onto carbon-coated copper grids on a Whatman filter paper and air dried.
Particle size distribution and polydispersity index measurement by dynamic light scattering (DLS), and stability analysis by zeta potential measurement
The size of PRK-NPs and the polydispersity index (PDI), an indicator of the particle size distribution width, were evaluated by a DLS detector (Malvern Instruments, Malvern, UK). Size determination was conducted using a disposable folded capillary cuvette free of air bubbles. To decrease sample opalescence before particle measurement, 1 mg of the NP was dispersed in distilled water at a 1:1 ratio. Physical stability was appraised by measuring particle size, PDI, and zeta potential after 4 weeks of storage at 4 °C [Citation32–34].
In vitro cytotoxicity measurements by MTT assay
The dose-dependent toxicity of PRK-NP was measured in cultures of the L6 mouse fibroblast line CRL-2976 and human breast cancer line MCF-7 (American Type Culture Collection, Manassas, VA, USA) using the MTT assay as described [Citation35,Citation36]. Briefly, PRK-NPs were dissolved in 10% dimethyl sulfoxide (DMSO) and added to cultures at the indicated concentrations in 1 ml Roswell Park Memorial Institute (RPMI) 1640 medium. Briefly, cells were seeded at 1 × 105/well in microtiter plates. The L6 mouse fibroblasts were treated with 10, 20, 30, 40, 50 and 60 µg as final concentrations and MCF7 cells with 2, 6, 10, 20, 30, 40, 50, 60, 70, 80, 90 and 100 µg as final concentrations for 24, 48 and 72 h. Absorbance at 570 nm was measured on an ELISA reader (Span Diagnostics, California, USA) in triplicate and the mean value was recorded. Cell viability was calculated as
where As is sample absorbance, Ab is the absorbance of the blank (medium), and Ac is the absorbance of untreated control cells.
Apoptosis assay by Annexin V-FITC/propidium iodide dual staining and fluorescence activated cell sorting (FACS)
Treated HEK293 cells with PRK-NP at the concentration of 320 µg/ml were stained with FITC-labeled Annexin V and propidium iodide (PI) to detect the early and late stages of apoptosis. Briefly, treated cells were washed three times in PBS, detached by trypsinization, and washed three times with 500 ml 1× binding buffer. Then, 1–4 × 106 cells were resuspended in 100 ml 1× binding buffer containing 5 ml of FITC-labeled Annexin V and incubated in the dark for 12 min at 37 °C. Cells were washed again with 500 ml of 1× binding buffer and incubated in 100 ml of 1× binding buffer with 5 ml of PI. Cell staining was analyzed using a FACS Calibur System (Becton Dickinson, Franklin Lakes, New Jersey, USA) with a 515–545 nm emission filter for FITC (green) and 600 nm for PI (red) [Citation33–35].
Assessment of PRK-NP in vivo toxicity, antitumor efficacy, and immunomodulation in zebrafish
The in vivo toxicity, antitumor efficacy, and immunomodulatory activity of PRK-NP were examined in a zebrafish model. Four groups of 12 adults wild type zebrafish inbred from Pentagrit animal housing clutches were housed under a 14-h/10-h light/dark cycle at 26 °C water temperature in 10-L tanks with continuous aeration and biofiltration. Fish were fed 5 mg commercial feed (TetraBit, Spectrum Brands, USA) per gram of body weight once daily. Water quality and tanks were maintained as per housing and maintenance standards of the Pentagrit animal housing committee. All protocols followed Good Animal Practices as specified by the Institutional Animal Ethics Committee in accordance with the Committee for the Purpose of Control and Supervision of Experiments (CPCSEA), India. The average initial body weight was approximately 0.5 g. Fish were fed 0 (placebo control), 3, 30 and 90 µg PRK-NP per day per gram body weight with the food pellets (termed the control group, group 1, group 2, and group 3, respectively). After 14 and 30 days of treatment, fish were euthanized by rapid cooling to 15 °C for examination of the acute and chronic effects of PRK-NP as described [Citation36].
For the antitumor study, fishes were inoculated with MCF-7 breast cancer cells and the PRK-NP administrated in feed at 0, 3, 30, and 90 µg/g body weight per day as described. In addition to PRK-NP, the antitumor efficacies of the clinical chemotherapeutics docetaxel and paclitaxel and of a NP synthesized with unprocessed mercury and using elemental sulphur without ligands (termed unprocessed Prakasine) were also examined. After 14 days, fishes were sacrificed and examined for the presence of liver tumours. Tissues were smeared on glass slides and stained with Haematoxylin and Eosin (HE) [Citation37] for 2 min each, followed by water washes. Slides were then examined under light microscopy at 45× magnification. An iPad 3rd generation attached to a gooseneck was used to capture images from a fluorescent microscope. Total tumour size was measured using ImageJ (NIH, Bethesda, MD, USA).
White blood cell proliferation assay
White blood cell (WBC) proliferation assays [Citation38] were performed during the initial, middle, and last phases of PRK-NP treatment (days 0, 7 and 14, respectively). Briefly, blood was collected from the tail and gills by bleeding, smeared on slides, and air dried for HE staining. Slides were viewed at 45× magnification using a Labomed LX-400 microscope.
Gene expression measurements by PCR
Effects of PRK-NP on the expression levels of immune-related genes were examined at a dose of 90 µg/g based on the results of antitumor assays. Target genes chosen () were the proinflammatory factors IL-1α, IL-1β, IL-2-ITK, IL-6, IL-8, IL-12, TNF-α, and IFN-γ and the immune suppressors IL-4, IL-5, IL-10, and TGF-β. Unprocessed Prakasine, docetaxel, and paclitaxel were also tested at 90 µg/g body weight per day. These doses are based on a previously described protocol [Citation39] and treatments were maintained for 15 days.
Table 1. Primer sequences and target gene references.
Gene expression assays were performed by PCR using Qiagen kits according to the manufacturer’s instructions and a previously described protocol [Citation40]. The zebrafish genome was screened using ZFIN.com and reference RNA sequences identified () for primer design using Primer.3 The expression level of each gene was measured three times per treatment condition from gel band peaks using ImageJ [Citation41]. In addition to gel PCR, the Rotor Gene Q real time PCR thermal cycler to identify the absolute quantification of the gene expression.
Blood cytokine assays
Cytokines, the proinflammatory IL-1α, IL-1β, IL-2-ITK, IL-6, IL-8, IL-12, TNF-α, and IFN-γ and the immune suppressive IL-4, IL-5, IL-10, and TGF-β were also measured in blood samples collected from the posterior ventral tail and gills using an ELISA reader (Span Diagnostics, California, USA) and Qiagen kits according to the manufacturer’s instructions. All measurements were conducted in triplicate.
Statistical analysis
Group means were compared by Student’s t-test or analysis of variance as indicated.
Results
Physicochemical properties of PRK-NPs
The Physicochemical properties of PRK-NPs was demonstrated with X-ray Photoelectron Spectroscopy Analysis, Analysis of powder XRD and FTIR, Zeta potential, SEM,TEM, EDAX and dynamic light scattering (DLS) characterization. Subsequently, in-vitro and in-vivo studies performed to establish the non-toxic immunological anticancer effect of PRK-NP.
X-ray photoelectron spectroscopy analysis
The surface composition and elemental analysis of the prepared PRK-NPs were characterised using an XPS technique. The shows the narrow XPS spectra of PRK-NP. The Hg 4f7/2 and 4f5/2 peaks appeared at 100.3 and 104.3 eV, respectively reveal the presence of Hg (). The peaks in the S energy region at 162.6 and 162.8 eV are attributable to S transition (2p3/2 and 2p1/2, ) while the S (2S1/2) peak appeared at 285.3 eV (). Ratio of Hg 4f to S 2p peak areas was 1.1:1, close to 1:1 for HgS. Broad XPS spectra () confirm the purity of HgS with C, N, O, S and Hg elements. Nitrogen gas purging during the synthesis of HgS-based NPs may have prevented oxidation [Citation42].
Figure 1. Chemical composition of Prakasine nanoparticles (PRK-NPs). (A–C) Narrow X-ray photoelectron spectra. (D) Survey scan. The spectra reveal the presence of (A) Hg, (B) HgS, (C) S, and (D) Hg compounds.
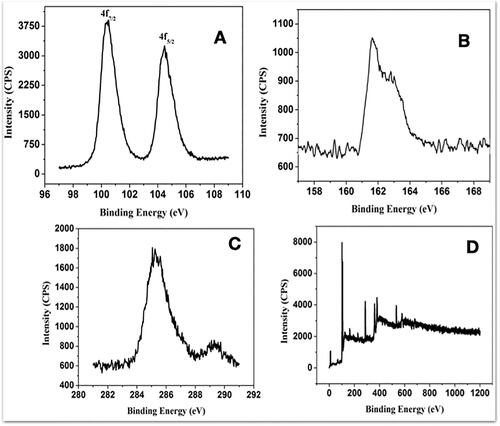
Analysis of powder XRD and FTIR
The XRD was generated using LabX XRD-6000 system using a monochromatized X-ray beam with nickel-filtered CuKα radiation (λ = 1.5418 Å) at 40 kV. A continuous scan mode was used to collect 2θ data. The diffraction shifts match diffraction peaks () corresponded to (102), (111), (200), (202), (300) and (220) planes, in good agreement with the JCPDS-pattern (JCPDS file No.19-0798) [Citation43] for HgS. Broadened signals in the XRD pattern indicated diminished dimensions of HgS nanoparticles, with estimated mean crystalline diameter of 60 nm using the Scherrer formula [Citation44]. To examine whether the surface of as synthesized PRK-NP was capped with an organic surface, FTIR detection was performed (). A Fourier transform infra-red spectroscopy (FT-IR) from the non-fractionated extract was obtained by a Perkin-Elmer FT-IR System Spectrum GX using a CaF2 cell. The spectrum was obtained on transmittance mode at a resolution of 0.3 cm−1, from 4000 to 500 cm−1. The band observed at 2600 cm−1 corresponds to S–H stretch vibration and the bands at 1614 and 752 cm−1 are related to C=N and C–S stretching, respectively [Citation45], further confirmed by the absence of S–H, C–N, and C–S peaks () and (), confirming that pure HgS while the insignificant amount of sulphur powder-base molecule was likely absorbed on the surface of HgS [Citation46].
Figure 2. PRK-NP purity and stability. (A) PRK-NP X-ray diffraction (XRD) spectrum pattern. The diffraction peaks are indicative of pure hexagonal phase HgS. (B) Fourier-transform infra-red (FTIR) spectrum indicates that HgS is capped with an organic surface. (B-a) FTIR shows that Hg is bonded to sulphide groups. (B-b) FTIR shows that the HgS product is free of impurities. (C–D) Zeta potential measurement before (C) and after storage (D).
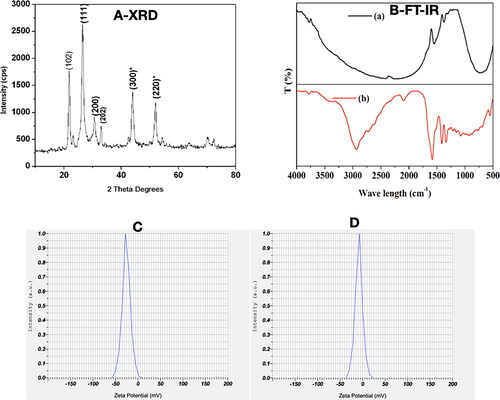
Zeta potential
The zeta potential of PRK-NP was −29.5 mV before storage and −8.5 mV after storage for 4 weeks at 4 °C (). The increase in zeta potential may be due to the presence of amino acid methionine and the mild agglomerate size of nanoparticles over time. The PRK-NPs’ zeta potential values −29.5 mV before storage and −8.5 mV after storage for 4 weeks, signifying that the Nano particles synthesized were negatively charged and moderately distributed in the medium. Generally, the negative sign of zeta potential is considered a good indication of colloidal stability with enhanced repulsion force ensuring optimal nanoparticles dispersibility. The value of zeta potential is highly dependent on individual ingredients composing the core/shell nanoparticles. Low PDI value indicates homogenous nanodispersion with optimum size distribution with no risk for aggregation.
SEM, TEM, EDAX and dynamic light scattering (DLS) characterization of PRK-NP
Commonly, SEM is to examine nanoparticle’s surface morphology and TEM is considered as a great tool to gather data about the size, dispersion, and morphology of the synthesized nanoparticles. ) presents SEM images of PRK-NPs produced by the solid state mercury nanoparticle synthesis method with different reaction times. Very fine particles were obtained at 54 h, while reducing the reaction time led to the formation of bulk irregular masses 100–150 nm in diameter. Typical energy dispersive spectroscopy (EDAX) spectra of samples demonstrating the presence of Hg and S are shown in . Analyses by TEM () at different resolutions revealed an average particle size of 90–100 nm. The surface composition of the synthesised PRK-NP was also visible by EDS-TEM [Citation47–49].
Figure 3. Characterization of PRK-NP morphology by scanning electron microscopy (SEM), energy dispersive spectroscopy (EDAX), and transmission electron microscopy (TEM). (A1–A3) SEM images of products fabricated by solid state synthesis with different reaction times. (A4) The EDAX scan indicates the presence of Hg and S in the product. (B) TEM images at different resolutions reveal an average particle size of 90–100 nm. (C) Size (diameter) distribution of PRK-NPs before storage. (D) Size distribution after storage for 4 weeks. (E) Zeta potential and particle dispersity index (DPI) as metrics of particle stability in storage.
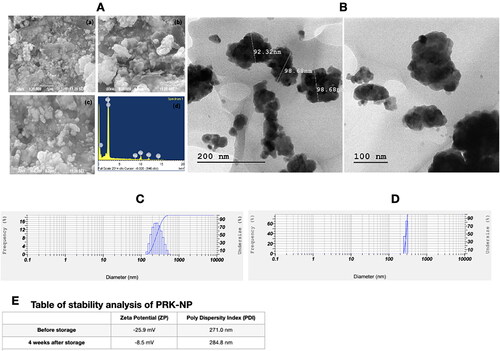
Figure 4. Nontoxicity of PRK-NPs against L6 mouse fibroblasts. (A) Summary of MTT assay results (% viable cells) for the indicated concentrations and exposure times. All treatments were conducted in triplicates. (B–D) Inverted Light microscopy images of the indicated treatment groups.
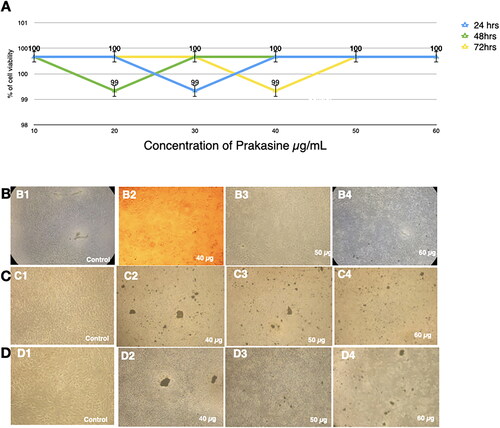
A size-distribution image as dynamic light scattering (DLS) of PRK-NPs is represented in . The detected mean distribution of PRK-NPs sizes are 271.0 and 284.8 nm with a PDI value of 0.542 before and after storage respectively. Here, the polydispersed nature of the PRK-NPs is mainly because of the negative zeta potential which averts the aggregation of the NPs among each other, resulting in well dispersed and stable materials. The mean particle size 271.0 nm before storage and 284.8 nm after storage indicating only a modest degree of aggregation (), and neither zeta potential nor PDI were substantially changed after storage ().
Absence of in vitro cytotoxicity of PRK-NP
Cultured L6 cells demonstrated 99% viability after treatment with 30 µg/mL PRK-NP for 24 h, 99% viability after 20 µg/mL PRK-NP exposure for 48 h, and 99% viability following 40 µg/mL PRK-NP treatment 72 h (). Similarly, viabilities of the MCF-7 cell line were 99% at 10 µg/mL for 24 h, 99% at 50 µg/mL for 48 h, and 99% at 40 µg/mL for 72 h (). Therefore, PRK-NP proved essential non-toxic in vitro.
Apoptosis measured by Annexin V-FITC/PI
In HEK293 cultures, 320 µg/mL PRK-NP induced early apoptosis in 1.92% of cells, late apoptosis in 6.35%, and necrosis in 2.26%. Further, total viability was 89.47% after treatment (), consistent with MTT results showing low cytotoxicity. The significant viability of HEK293 cells demonstrate that the PRK-NP does not produce genotoxicity.
Figure 6. Low apoptosis rate of the HEK293 cells in response to the PRK-NP treatment. The apoptosis rate was assessed by fluorescence activated cell sorting (FACS) analysis of cells double-stained with Annexin V and propidium iodide. (A) Control and (B) PRK-NP-treated cells.
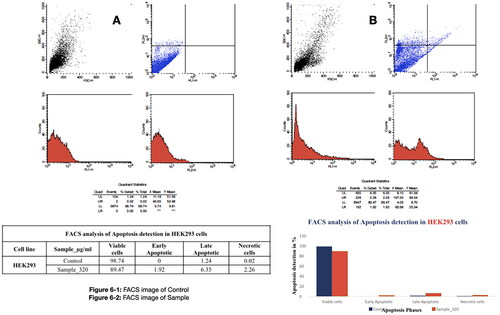
Absence of in vivo toxicity and immunological antitumor activity of PRK-NP
No tumour cells were detected in liver samples from zebrafish treated with 90 µg/g PRK-NP () with suppressed neovascularization on day 15, while neovascularization in the 3 µg/g treatment group was comparable to that of untreated MCF-7 inoculated zebrafish on day 15 [] and 30 µg/g only partially reduced neovascularization []. On day 15, tumour samples from the 90 µg/g dose group exhibited numerous necrotic cells [], while no such cells were observed on day 45, indicating that necrosis is the primary death pathway to eradicate the tumour. In contrast to tumour tissue, no toxicity was observed in visceral organs, indicating that PRK-NPs can exert a potent antitumor effect without toxicity to nontarget tissues ( and ). Also, there are no significant changes in the survival rate, growth, and development of zebrafish compared with the control group as a measure of biotoxicity in vivo indicate there is no biotoxicity.
Figure 7. Low acute PRK-NP toxicity in zebrafish. (A) Tissues from untreated control animals: (1) heart (gross morphology), (2) myocardial fibres, (3) brain section, (4) liver (gross morphology), (5) liver histology. (B–D) Tissues from zebrafish receiving the compound at a concentration of (B) 3 µg/g body weight, (C) 30 µg/g body weight, or (D) 90 µg/g body weight.
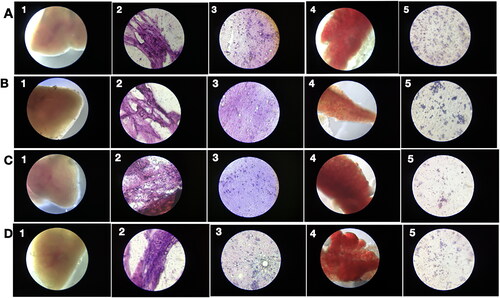
Figure 8. PRK-NP antitumor activity of and its effects on WBC proliferation in zebrafish. (A) Liver tumour elimination by high-dose PRK-NP treatment. The tissue samples were obtained 14 days after the treatment. (1) Control zebrafish not inoculated with liver cancer cells with normal tissues and normal cell spacing (black arrows). (2) Liver tissue at day 14 after inoculation with liver cancer cells without treatment shows a more disorganised tissue structure, abnormal growth, and irregular membrane patterns (red arrows). (3–5) Reduced number of tumour cells and absence of cancer cells at 3 and 90 µg/g PRK-NP, respectively. (B) PRK-NP-mediated suppression of neovascularization in liver tissues. (1) The control tissue from uninoculated zebrafish shows no neovascularization, established blood vessels, or new capillary formation. (2) Well-established neovascularization and capillary networks indicative of fully functional tumour tissue 14 days after inoculation without intervening treatment. (3–5) Thinner neovascularization to the absence of neovascularization at 3, 30 and 90 µg/g of PRK-NP, respectively, 14 days after the inoculation. (C) Visualization of WBC proliferation in response to liver cancer cell inoculation and PRK-NP treatment. The WBC number gradually increased from day 0 to 7 and returned to the baseline by day 14, concomitant with tumour elimination in tumour models treated with 90 µg/g of PRK-NP. (D) The treatment with 90 mg/g of PRK-NP markedly reduced the tumour size (to 6% of that of the inoculated control animals). (E) Difference in the WBC proliferation rate following inoculation and PRK-NP treatment. The graph indicates an increased WBC number of approximately 16 × 106 cells/mL blood on day 7 post-inoculation in the 90 µg/g group, which reduced to approximately 7 × 106 cells by day 14. No significant changes could be observed in the WBC number in the other groups.
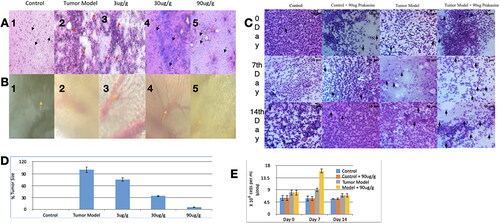
Stimulation of WBC proliferation by PRK-NP
In the WBC proliferation assay, tumour-bearing treatment group a 3-fold increase in WBC proliferation rate compared to untreated controls []. This indicates the immunogenicity potential of PRK-NP.
Stimulation of cytokine gene expression by PRK-NP
Gene expression profiling was then conducted using 90 µg/g PRK-NP due to the efficacy of this dose for tumour suppression. Untreated control and PRK-NP-treated control groups demonstrated a similar cytokine profile at both day 7 and 14 post-treatment, indicating that PRK-NP had no acute immune induction activity in the absence of tumours [, and ]. Untreated tumour-bearing zebrafish exhibited modest rises in the expression of proinflammatory factors IL-1α, IL-1β, IL-2-ITK, IL-6, IL-8, IL-12, TNF-α, and IFN-γ, and slight decreases in expression of the anti-inflammatory factors IL-4, IL-5, IL-10, and TGF-β compared to untreated controls. Thus, the presence of tumours appears to induce a proinflammatory state. In PRK-NP-treated tumour-bearing zebrafish; however, the proinflammatory factors have elevated an average of 15-fold compared to untreated controls and 5-fold compared to untreated tumour-bearing zebrafish. Conversely, the average expression of anti-inflammatory factors was reduced 10-fold compared to controls and 3-fold compared to untreated tumour-bearing zebrafish. Thus, PRK-NP induces a stronger proinflammatory state in the presence of tumours, suggesting that the observed reduction in tumour load results from local inflammation and ensuing cell death at the tumour site.
Figure 10. Gene expression profiling of zebrafish liver following tumour cell inoculation and (or) PRK-NP treatment. (A) Gene expression profile on day 7. (B) Gene expression profile on day 14. (The assay values are average values of triplicates.).
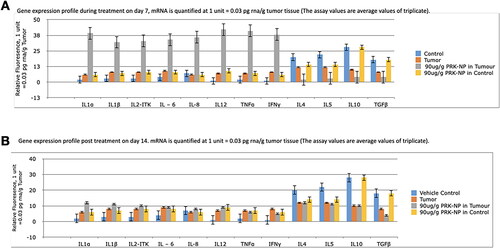
Figure 11. Changes in the immune-related gene expression levels within liver tumour samples following the treatment with 90 µg/g of PRK-NP or unprocessed mercury compound. (A) After 7 days of treatment. (B) After 14 days of treatment. The results are expressed as the average values of triplicate RT-qPCR measurements. In general, PRK-NP increased the expression of proinflammatory factors and suppressed that of anti-inflammatory factors. (The assay values are average values of triplicates.).
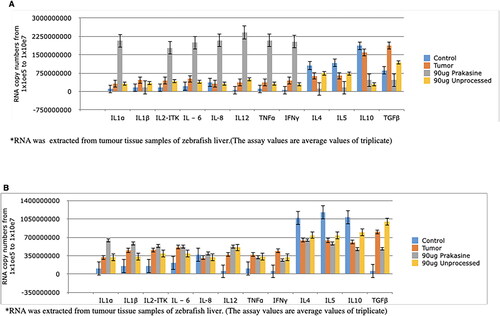
Figure 12. Changes in serum levels of immune-related factors following the treatment with 90 µg/g of PRK-NP or unprocessed mercury compound. (A) After 7 days of treatment. (B) After 14 days of treatment. The results are expressed as the average values of triplicate PCR measurements. The blood samples were obtained from the posterioventral tail and gills. The concentrations were measured by ELISA. The pattern of concentration changes generally reflected the changes in the tumour expression levels. (The assay values are average values of triplicates).
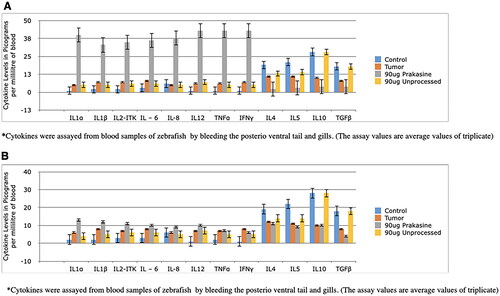
The antitumor efficacy, nontarget toxicity, and proinflammatory induction capacity of PRK-NP were also compared to unprocessed Prakasine (synthesised from unprocessed mercury without ligand) as well as to the clinical chemotherapeutic drugs docetaxel and paclitaxel. The PRK-NP performed better than others ().
Discussion
Recent advances in nanotechnology for cancer treatment include magnetic nanocapsules that promote tumour destruction when combined with ultrasound stimulation [Citation50], folate-conjugated gold NPs [Citation51], that sensitize tumours to ultrasound irradiation [Citation52] AgCl NPs biosynthesized by E. coli lysate with cytotoxic effects on human breast cancer cells [Citation53], and non-toxic transformable peptide NPs shown to arrest HER2 signaling and induce cancer cell apoptosis in a mouse xenograft model of HER2+ breast cancer when used as monotherapy [Citation13]. In addition, TiO2 microparticles decorated with nano spikes (spiky particles) were shown to activate and amplify the immune response by exerting mechanical stress on macrophages and dendritic cells, resulting in potassium efflux and inflammasome activation during phagocytosis and subsequent protective immunity against tumour growth and influenza viral infection [Citation14]. Nanoparticles with specific ratios of positively and negatively charged ligands can selectively target lysosomes in cancerous cells and induce cytotoxicity. However, only a few small molecules—mostly repurposed drugs—have been tested with NPs so far, and these typically exhibit low cancer selectivity [Citation15]. The NP Nano-sapper was shown to simultaneously promote CTL recruitment and CTL infiltration by reducing physical obstacles in the tumour microenvironment, thereby potentiating immunotherapy against immune-excluded tumours [Citation16]. An iron oxide NP was also shown to enhance the delivery of doxorubicin across the blood–brain barrier (BBB) for glioblastoma therapy [Citation54]. Other cancer immunotherapies with potential nanotechnology applications include oncolytic virus therapies, cancer vaccines, cytokine therapies, adoptive cell transfer, and immune checkpoint inhibitors [Citation55]. In most cases, however, there are still substantial challenges to overcome before clinical application. In contrast, PRK-NP is stable, non-toxic in the absence of inflammatory activity, and a potent activator of tumour inflammation. Therefore, PRK-NP may be among the more promising candidate NPs for cancer immunotherapy.
Prakasine is a non-toxic mercury-based nanoparticle (diameter <100 nm) shown to increase humoral immunity against Newcastle disease virus in my previous study [Citation24]. A mercury-based nanoparticle termed Prakasine nanoparticle (PRK-NP) was developed according to the “Prakash theory of metal drugs” (patent registration Ref. No. 5776/CHE/2014). My theory is as follows:
When metal atoms are attached to sulphur atoms with a coordinated covalent bond gives rise to a metallic sulphur complex, if this metallic sulphur complex is attached further to the organic compound (ligand), the organometallic complex will be formed, which will not produce any toxicity or toxicity in less quantity.
This metallic sulphur complex will form the disulphide bond in vivo and evince therapeutic action with less adverse effects.
Some metals particularly mercury attaches with sulphur and form a covalent bond even though other elements present in a biochemical reaction. The bond between Hg and S cannot be broken by biochemical reactions both in vivo and in vitro.
The theory posits that the attachment of metal atoms to sulphur atoms and subsequent attachment of ligands yields complexes without metal toxicity due to the stable disulphide bonds. The stable disulphide bonds (coordinated covalent bond) between metal-sulphur-ligands (Hg-S-X) atoms could not be breakable by any of the biochemical reactions inside the human body and avoid the free metal atoms (Hg++) getting attached with sulphur of the sulphur-containing amino acids. Thus, the adverse effects, accumulations, and toxicity are being avoided as well.
In the current study, PRK-NP suppressed tumour development in zebrafish by enhancing antitumor immunity without inherent cytotoxicity in vitro or induction of non-target tissue damage in vivo. Metals such as mercury and platinum have antitumor efficacy but are also cytotoxic to healthy tissue. The binding energy of mercury in Prakasine as measured by XPS is markedly higher compared to other mercury compounds [Citation25–28], which may explain the relatively low cytotoxicity.
The toxicity of PRK-NP against MCF-7 cells in vitro was reduced after 24 h of treatment in addition to insignificant changes in the survival rate, growth, and development of zebrafish compared with the control group suggesting that this NP rapidly loses cytolytic activity due to biodegradation. This property may enhance the safety for clinical applications by preventing nontarget toxicity during long-term treatment. Mercury compounds damage cells by covalently binding to sulphur-containing amino acids, thereby inhibiting protein function and interfering with various biochemical reactions [Citation56]. In PRK-NPs; however, the mercury atoms are first covalently bound to sulphur atoms, and the Hg–S complex is bound covalently to sulphur-containing ligands on the surface. This tight binding to sulphur prevents the dissociation of mercury and subsequent binding to proteins. In addition to stabilizing ligands, it may also be possible to produce PRK-NPs with additional bioactive ligands for enhanced clinical efficacy. Alternatively, the unprocessed Prakasine demonstrated no anticancer efficacy or immune induction capacity and resulted in high lethality, suggesting substantial Hg-mediated toxicity.
From the results of my present study, the physicochemical properties, analysis of powder XRD and FTIR, zeta potential, SEM, and TEM characterization of PRK-NP confirm that the PRK-NP is having nanoparticle characters. The results of in-vitro toxicity, apoptosis, and zebrafish toxicity studies confirm that the PRK-NP is non-toxic once again next to my previous study. The results of Immunological antitumor activity, stimulation of WBC proliferation, and stimulation of cytokine gene expression studies confirm that PRK-NP is having therapeutic efficacy.
Collectively, these toxicity ( and ), mortality, antitumor, and gene expression studies suggest that PRK-NP (at 90 mg/g) selectively damages tumour cells by upregulating the expression of proinflammatory mediators and suppressing anti-inflammatory mediators within the tumour tissue. The effects of PRK-NP on WBC proliferation paralleled the effects on tumour burden and immune-related gene expression, again suggesting that antitumor activity is mediated by the immune system. Moreover, Prakasine was more efficacious at reducing tumour volume, preventing mortality, and inducing an intratumoral immune response than the clinical chemotherapy drugs docetaxel and paclitaxel.
These findings once again suggest the understanding of our previous studies in HIV that Prakasine is also effective against both HIV and cancer without inducing toxicity, as the same inflammatory factors upregulated by PRK-NP (IL-1α, IL-1β, IL-2, IL-6, IL-8, IL-12, TNF-α and IFN-γ) are required to eliminate the causative pathogen HIV, depending on the secretion pattern of cytokines, CD4 T cells could be differentiated into Th1 and Th2 cells. The Th1 CD4 cells primarily produce interleukin-2 (IL-2) and IFN-γ, representing the cytokines that support the effector functions of the immune system (CTL, NK cells, and macrophages) able to recognize and eliminate both virus-infected and cancer cells. These cytokines can directly stimulate immune effector cells and stromal cells at the tumour site and enhance tumour cell recognition by cytotoxic effector cells. Numerous animal model studies have demonstrated broad antitumor activity of cytokines and indeed led to the development of several cytokine-based approaches for cancer therapy [Citation57]. Moreover, IFN-γ has been shown to enhance CTL and NK cell activities against HIV-1-infected cells and to regulate the immune response [Citation58,Citation59]. In addition, the upregulated IFN-γ due to PRK-NP stimulation suppresses HIV-1 LTR promoter activity, affects viral transcription [Citation60–62], and it could suppress the oncogene LTR promoter activity as well. The compound produced, PRK-NP, could also eliminate HIV based on the proposed effector mechanism (; see also S.K. Prakash, April 18-20, EECAAC 2018, Moscow, Russia, page: 256 “Prakasine as the potential drug toward the HIV elimination”), although further studies are required to generate more data for confirmation despite the correlation and relevancy demonstrated between my doctoral and previous studies and this present study that increases IL-2 and IFN-γ levels lead to HIV elimination and tumour reduction. Again it is proposed by corroborating my studies, while there is a lack of CTL killing mechanism in HIV and Cancer individuals when the Prakasine nanomedicine being administered, the nanoparticle gets attached with the unique receptor in unique domain and triggering the unique singling pathway to induce the cytokine gene expression. The increased cytokines IL-1α, IL-1β, IL-2 IL-6, IL-8, IL-12, TNF-α, and IFN-γ increase the natural immunity, adoptive immunity and cell mediated immunity. The increased CD8 cells due to increased cell mediated immunity gets sensitized with HIV or cancer antigen and becomes HIV or cancer specific Cytotoxic T Lymphocytes.
The relevancy of PRK-NP to eliminate HIV and cancer is the increasing level of cytokines that can influence the activity of B cells, TC cells, NK cells, macrophages, granulocytes, and haematopoietic stem cells, thereby activating the entire network of interacting cells and the immune system. IL-2, IFN-γ, and other cytokines interplay in the network, and this networking increases CTLs in both HIV and cancer towards elimination. The increased CTL destroys the HIV and tumour-harboring cells by chemotaxis mechanism as the antigen sensitized CTL is having the tendency to find the foreign protein-harboring cells by binding and discharging perforin and granzymes into HIV and cancer cells through the pores of the cytoplasmic membrane thus formed. Cumulatively, through PRK-NP-stimulated cytokine upregulation, the subsequent cascading mechanism is induced related to the upregulated cytokines affecting the activated antigen-specific CTLs and NK cells and increasing their clonal population to destroy cancer and HIV cells as well. Therefore, based on several studies, Prakasine has potential therapeutic effects and can be used to treat cancer and HIV.
Conclusion
This study demonstrates that a mercury-based nanoparticle can be rendered non-toxic by strong complexation with sulphur and surface capping with sulphur-containing ligands and that such compounds can still demonstrate highly specific antitumor activity through immune induction. Thus this non-toxic mercury nanoparticle and its immunotherapy potential are the novelty when compare to the regular existing state of art mercury and mercury compounds. Prakasine has potential therapeutic effects and can be applied to treat cancer and HIV if these two conditions are concurrently present in an individual or independently present in different individuals.
The study concludes the significant effectiveness of PRK-NP in cancer reduction in zebrafish better than existing standard anti-cancerous drugs. Future clinical investigations should be performed to assure the therapeutic value of PRK-NP in humans.
Author contributions
Dr. SK Prakash was involved in the conception and design, or analysis and interpretation of the data; the drafting of the paper, revising it critically for intellectual content; and the final approval of the version to be published
Acknowledgments
I wish to express my gratitude to K. Vincent, St. Joseph College, Trichy, for advice on FTIR, Madras University for help on XPS, and Karunya University for assistance with XRD, EDAX, SEM, and TEM studies. I would also like to thank Dr. M. Parthiban, Department of Biotechnology, Madras Veterinary College, for assistance with in vitro cell culture studies. I also acknowledge Ben Joseph and P. Kalaichitra for assistance with zebrafish studies at Pentagrit Ltd, Chennai. I thank the Nishka lab (Hyderabad) and Skanda lab (Bangalore) for assistance on DLS and FACS studies, respectively.
Disclosure statement
No potential conflict of interest was reported by the author(s).
Data availability statement
The data that support the findings of this study are available from the corresponding author, [Prakash SK], upon reasonable request.
Additional information
Funding
References
- Uhlen M, Zhang C, Lee S, et al. A pathology atlas of the human cancer transcriptome. Science. 2017;357(6352):6352.
- Siegel RL, Miller KD, Jemal A. Cancer statistics, 2020. CA A Cancer J Clin. 2020;70(1):7–30.
- Samadian H, Hosseini-Nami S, Kamrava SK, et al. Folate-conjugated gold nanoparticle as a new nanoplatform for targeted cancer therapy. J Cancer Res Clin Oncol. 2016;142(11):2217–2229.
- Liu L, Xu K, Wang H, et al. Self-assembled cationic peptide nanoparticles as an efficient antimicrobial agent. Nat Nanotechnol. 2009;4(7):457–463.
- Liu Y, Hardie J, Zhang X, et al. Effects of engineered nanoparticles on the innate immune system. Semin Immunol. 2017;34:25–32.
- Smith DM, Simon JK, Baker JR. Applications of nanotechnology for immunology. Nat Rev Immunol. 2013;13(8):592–605.
- Conway A, Vazin T, Spelke DP, et al. Multivalent ligands control stem cell behaviour in vitro and in vivo. Nat Nanotechnol. 2013;8(11):831–838.
- Shi J, Kantoff PW, Wooster R, et al. Cancer nanomedicine: progress, challenges and opportunities. Nat Rev Cancer. 2017;17(1):20–37.
- Davis ME, Chen ZG, Shin DM. Nanoparticle therapeutics: an emerging treatment modality for cancer. Nat Rev Drug Discov. 2008;7(9):771–782.
- Smith TT, Stephan SB, Moffett HF, et al. In situ programming of leukaemia-specific T cells using synthetic DNA nanocarriers. Nat Nanotechnol. 2017;12(8):813–820.
- Blanco E, Shen H, Ferrari M. Principles of nanoparticle design for overcoming biological barriers to drug delivery. Nat Biotechnol. 2015;33(9):941–951.
- Weissleder R, Nahrendorf M, Pittet MJ. Imaging macrophages with nanoparticles. Nat Mater. 2014;13(2):125–138.
- Zhang L, Jing D, Jiang N, et al. Transformable peptide nanoparticles arrest HER2 signaling and cause cancer cell death in vivo. Nat Nanotechnol. 2020;15(2):145–153.
- Wang J, Chen HJ, Hang T, et al. Physical activation of innate immunity by spiky particles. Nat Nanotechnol. 2018;13(11):1078–1086.
- Borkowska M, Siek M, Kolygina DV, et al. Targeted crystallization of mixed-charge nanoparticles in lysosomes induces selective death of cancer cells. Nat Nanotechnol. 2020;15(4):331–341.
- Huang Y, Chen Y, Zhou S, et al. Dual-mechanism based CTLs infiltration enhancement initiated by nano-sapper potentiates immunotherapy against immune-excluded tumors. Nat Commun. 2020;11(1):622.
- Chakraborty C, Sharma AR, Sharma G, et al. Zebrafish: a complete animal model to enumerate the nanoparticle toxicity. J Nanobiotechnology. 2016;14(1):65.
- Kaufman CK, Mosimann C, Fan ZP, et al. A zebrafish melanoma model reveals emergence of neural crest identity during melanoma initiation. Science. 2016;351(6272):aad2197–453.
- Javed I, Peng G, Xing Y, et al. Inhibition of amyloid beta toxicity in zebrafish with a chaperone-gold nanoparticle dual strategy. Nat Commun. 2019;10(1):3780.
- Jia HR, Zhu YX, Duan QY, et al. Nanomaterials meet zebrafish: toxicity evaluation and drug delivery applications. J Control Release. 2019;311–312:301–318.
- Zhou W, Tian D, He J, et al. Prolonged exposure to carbon nanoparticles induced methylome remodeling and gene expression in zebrafish heart. J Appl Toxicol. 2019;39(2):322–332.
- Langenau DM, Zon LI. The zebrafish: a new model of T-cell and thymic development. Nat Rev Immunol. 2005;5(4):307–317.
- Leslie M. Zebrafish larvae could help to personalize cancer treatments. Science. 2017;357(6353):745.
- Prakash SK. Effect of feed supplementation of mercury nanoparticles on immunostimulation of live lentogenic Newcastle disease vaccine in layer birds. Indian Vet J. 2017;94:11–13.
- Goswami N, Giri A, Kar S, et al. Protein-directed synthesis of NIR emitting, tunable HgS quantum dots and their applications in metal-ion sensing. Small. 2012;8(20):3175–3184.
- Hutson ND, Attwood BC, Scheckel KG. XAS and XPS characterization of mercury binding on brominated activated carbon. Environ Sci Technol. 2007;41(5):1747–1752.
- Ley L, Pollak RA, McFeely FR, et al. Total Valance—band densities of states of III − V and II– VI compounds from X-ray photoemission spectroscopy. Phys Rev B. 1974;9(2):600–621.
- Marimuthu G, Ramalingam K, Rizzoli C, et al. Solvothermal preparation of nano-β-HgS from a precursor, bis(dibenzyldithiocarbamato)mercury(II). J Nanopart Res. 2012;14(2):710.
- Paulkumar K, Gnanajobitha G, Vanaja M, et al. Piper nigrum leaf and stem assisted green synthesis of silver nanoparticles and evaluation of its antibacterial activity against agricultural plant pathogens. ScientificWorld Journal. 2014;2014:829894.
- Lupusoru RV, Pricop DA, Uritu CM, et al. Effect of TAT-DOX-PEG irradiated gold nanoparticles conjugates on human osteosarcoma cells. Sci Rep. 2020;10(1):6591.
- Androussi Y. Transmission electron microscopy analysis of the shape and size of semiconductor quantum dots. Philos Mag Lett. 1999;79(4):201–208.
- Nemati E, Mokhtarzadeh A, Panahi-Azar V, et al. Ethambutol-loaded solid lipid nanoparticles as dry powder inhalable formulation for tuberculosis therapy. AAPS PharmSciTech. 2019;20(3):120.
- Bakhtiary Z, Barar J, Aghanejad A, et al. Microparticles containing erlotinib-loaded solid lipid nanoparticles for treatment of non-small cell lung cancer. Drug Dev Ind Pharm. 2017;43(8):1244–1253.
- Vandghanooni S, Forouharmehr A, Eskandani M, et al. Cytotoxicity and DNA fragmentation properties of butylated hydroxyanisole. DNA Cell Biol. 2013;32(3):98–103.
- Baharifar H, Amani A. Cytotoxicity of chitosan/streptokinase nanoparticles as a function of size: an artificial neural networks study. Nanomedicine. 2016;12(1):171–180.
- Wallace CK, Bright LA, Marx JO, et al. Effectiveness of rapid cooling as a method of euthanasia for young zebrafish (Danio rerio). J Am Assoc Lab Anim Sci. 2018;57(1):58–63.
- Saadat N, Akhtar S, Goja A, et al. Dietary garcinol arrests pancreatic cancer in p53 and K-ras conditional mutant mouse model. Nutr Cancer. 2018;70(7):1075–1087.
- Halwani R, Vazquez-Tello A, Sumi Y, et al. Eosinophils induce airway smooth muscle cell proliferation. J Clin Immunol. 2013;33(3):595–604.
- Chen M, Li J, Liang J, et al. Systemic toxicity reported for CDK8/19 inhibitors CCT251921 and MSC2530818 is not due to target inhibition. Cells. 2019;8(11):1413.
- Olsson K, Gerard CJ, Zehnder J, et al. Real-time t(11;14) and t(14;18) PCR assays provide sensitive and quantitative assessments of minimal residual disease (MRD). Leukemia. 1999;13(11):1833–1842.
- Antiabong JF, Ngoepe MG, Abechi AS. Semi-quantitative digital analysis of polymerase chain reaction-electrophoresis gel: potential applications in low-income veterinary laboratories. Vet World. 2016;9(9):935–939.
- Hsu‐Kim H, Sedlak DL. Similarities between inorganic sulfide and the strong Hg(II)‐complexing ligands in municipal wastewater effluent. Environ Sci Technol. 2005;39(11):4035–4041.
- Jenkins R, Snider R. L. Chemical analysis. In: Introduction to X-ray powder diffractometry. New York: John Wiley & Sons Inc, 1996. DOI:10.1002/9781118520994
- Marimuthu G, Ramalingam K, Rizzoli C. Predominant ionic interactions in CdS4N2 and HgS4 coordination environments. J Coord Chem. 2013;66(4):699–711.
- Zhang J, Chen Z, Wang Z, et al. The synthesis of HgS microcrystallites with controllable structure and morphology. Mater Res Bull. 2004;39(14–15):2241–2247.
- Kandasamy N, Saravanan S, Nayak DR. Synthesis and characterization of mercury doped and un-doped manganese sulfide nanostructure. International Conference on Nanoscience, Engineering and Technology (ICONSET); 2011. p. 104–106. DOI:10.1109/ICONSET.2011.6167922
- Carotenuto G, Nicolais L, Nicolais F. Synthesis of polymer-embedded metal, semimetal, or sulfide clusters by thermolysis of mercaptide molecules dissolved in polymers. MATS. 2008;1(1):1–11.
- Wang DS, Zheng W, Hao CH, et al. A synthetic method for transition-metal chalcogenide nanocrystals. Chemistry. 2009;15(8):1870–1875.
- Kim YY, Walsh D. Metal sulfide nanoparticles synthesized via enzyme treatment of biopolymer stabilized nanosuspensions. Nanoscale. 2010;2(2):240–247.
- Shakeri-Zadeh A, Khoee S, Shiran MB, et al. Synergistic effects of magnetic drug targeting using a newly developed nanocapsule and tumor irradiation by ultrasound on CT26 tumors in BALB/c mice. J Mater Chem B. 2015;3(9):1879–1887.
- Beik J, Khademi S, Attaran N, et al. A nanotechnology-based strategy to increase the efficiency of cancer diagnosis and therapy: folate-conjugated gold nanoparticles. Curr Med Chem. 2017;24(39):4399–4416.
- Beik J, Shiran MB, Abed Z, et al. Gold nanoparticle‐induced sonosensitization enhances the antitumor activity of ultrasound in colon tumor‐bearing mice. Med Phys. 2018;45(9):4306–4314.
- Bigdeli R, Shahnazari M, Panahnejad E, et al. Cytotoxic and apoptotic properties of silver chloride nanoparticles synthesized using Escherichia coli cell-free supernatant on human breast cancer MCF 7 cell line. Artif Cells Nanomed Biotechnol. 2019;47(1):1603–1609.
- Norouzi M, Yathindranath V, Thliveris JA, et al. Doxorubicin-loaded iron oxide nanoparticles for glioblastoma therapy: a combinational approach for enhanced delivery of nanoparticles. Sci Rep. 2020;10(1):11292.
- Zhang Y, Zhang Z. The history and advances in cancer immunotherapy: understanding the characteristics of tumor-infiltrating immune cells and their therapeutic implications. Cell Mol Immunol. 2020;17(8):807–821.
- Brunton LL, Hilal-Dandan R, Knollmann BC. Goodman and Gilman’s the pharmacological basis of therapeutics.13th ed. New York: McGraw-Hill; 2006.
- Lee S, Margolin K. Cytokines in cancer immunotherapy. Cancers. 2011;3(4):3856–3893.
- Roff SR, Noon-Song EN, Yamamoto JK. The significance of interferon-γ in HIV-1 pathogenesis, therapy, and prophylaxis. Front Immunol. 2014;4:498.
- Januškevica I, Rozentâle B, Hagina E, et al. Role of interferon gamma (IFN-γ) in immune response regulation in HIV-1 and HIV-1 + Mycobacterium tuberculosis (TB) infected patients. Proc Latv Acad Sci B. 2016;70.211–214.
- Prakash SK. Immunological and virological effects of novel prakasine nanomedicine in HIV-infected patients in South India: a preliminary study. Virol Antivir Res. 2020;9(2).195.
- Prakash SK. Phytochemical therapy as a possible cure for asymptomatic AIDS patients [dissertation]. India: Bharathidasan University; 2010.
- Prakash SK. Immunological and virological effects of Prakasine nanomedicine in HIV eradication: a preliminary study. Fifth Eastern Europe and Central Asia AIDS conference; 2016 Mar 23–25, Moscow.