Abstract
Recent years have seen the development of a variety of mammalian library approaches for display and secretion mode. Advantages include library approaches for engineering, preservation of precious immune repertoires and their repeated interrogation, as well as screening in final therapeutic format and host. Mammalian display approaches for antibody optimization exploit these advantages, necessitating the generation of large libraries but in turn enabling early screening for both manufacturability and target specificity. For suitable libraries, high antibody integration rates and resulting monoclonality need to be balanced – we present a solution for sufficient transmutability and acceptable monoclonality by applying an optimized ratio of coding to non-coding lentivirus. The recent advent of microfluidic-assisted hit discovery represents a perfect match to mammalian libraries in secretion mode, as the lower throughput fits well with the facile generation of libraries comprising a few million functional clones. In the presented work, Chinese Hamster Ovary cells were engineered to both express the target of interest and secrete antibodies in relevant formats, and specific clones were strongly enriched by high throughput screening for autocrine cellular binding. The powerful combination of mammalian secretion libraries and microfluidics-assisted hit discovery could reduce attrition rates and increase the probability to identify the best possible therapeutic antibody hits faster.
Introduction
Display libraries based on phage [Citation1], yeast [Citation2], or ribosomal display have proven to be powerful and robust methodologies for therapeutic antibody hit discovery, indicated by a progressively matured development pipeline and approved antibody panels. All these approaches inherit the basic principle of physical linkage of the antibody product to its respective genetic information enabling identification of sequences with desired binding properties. While such libraries allow for conservation and iterative interrogation of large diversities from various sources, the hosts such as yeast or bacteria impede screening in full-length and final therapeutic formats. This on the one hand results in the need to re-format and re-produce primary hit candidates which in turn can lead to attrition within a hit discovery process [Citation3].
Construction of large mammalian libraries in turn is comparably sophisticated, labour-intensive and time-consuming. But such libraries offer the opportunity to conduct hit discovery or optimization in a host similar or same to production later-on and in an envisioned final format. Therapeutic antibody production is typically carried out in CHO cells, which themselves can be heavily engineered for high specific production rates [Citation4]. Beyond antibody discovery, CHO libraries have recently also been utilized for rapid characterization of virus surface protein variants and specific neutralizing antibodies [Citation5].
Mammalian libraries are often generated in human embryonic kidney (HEK293) or Chinese hamster ovary (CHO) cells, and to some extent in certain inherently suitable B cell subclones. Library generation is realized by, among others, lentiviral delivery [Citation6–11], vaccinia virus [Citation12], nucleases [Citation13–15], recombinases [Citation16–19], transposases [Citation20], or episomal approaches [Citation21] in conjunction with somatic hypermutation [Citation22] or unidirectional gene conversion [Citation23]. Similar to other random integration-based methodologies, lentiviral library generation warrants optimization towards acceptable levels of monoclonality to ensure reasonable downstream process efficiency. We report here that the combination of an adapted multiplicity of infection (MOI) with an optimized ratio of coding to non-coding lentivirus can result in balanced acceptable transducibility and high monoclonality, supporting effective library screens.
Libraries can be designed to display the candidates on the surface of the cell, secrete them into the supernatant, or enable both at the same time. Antibody display can be utilized for optimization such as affinity maturation [Citation9,Citation15,Citation20–22], to screen for enhanced manufacturability [Citation24] or in an autocrine binding for a desired function [Citation7]. While some designs include simultaneous display and secretion [Citation22,Citation25], recent work has leaned to secretion libraries in combination with microfluidics-assisted screens that become routine antibody discovery methodologies in pharmaceutical research and development [Citation26–28]. Antibodies secreted from single cells are interrogated after compartmentalization in droplets [Citation29], nanostructures such as nanopens [Citation30], or microcapillaries [Citation31–33]. Compatible with the high throughput of microfluidic setups, mostly fluorescence-based methods are applied for binding to recombinant targets or cells [Citation34], for internalization or functional screening [Citation6,Citation35–37].
Exemplary workflows and potential advantages are schematically presented in . Following library generation from various procurable immune repertoires, sorting for desired attributes can be carried out in display or secretion mode. In addition to single-cell PCR recovery or NGS and transient reproduction for confirmatory assays as mostly implemented when screening primary secreting cells, secretion libraries include an option for sub-cultivation of stable secretion hit candidate clones.
Figure 1. Applications and Opportunities (in blue) for mammalian libraries in antibody engineering and hit discovery. Libraries from precious diversities such as elite responders can be cloned in desired therapeutic formats and produced in display or secretion mode for repeated interrogation by flow cytometry or microfluidics, respectively. Hit candidate recovery by PCR gene amplification & transient re-production or single cell sub-cultivation enable secondary hit confirmation. Mammalian libraries offer several advantages for each step highlighted in blue and discussed in the main text.
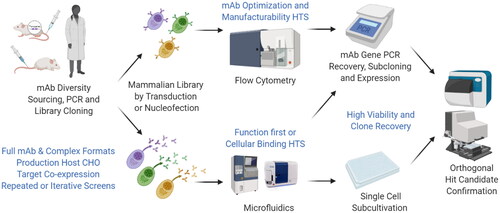
There work presented here comprises methodological contributions to five essential steps in antibody hit discovery. (1) Applying lentiviral delivery for library generation with optimization of MOI and ratio of coding to non-coding lentivirus, a high degree of monoclonal antibody display could be achieved on the surface of CHO cells. (2) Differences in surface display of published reference antibodies matched with reported poor to good manufacturability properties and this discrimination enabled enrichment for a clone with superior expression profile and desired target specificity. (3) Conditions optimized in display setting were then applied to antibody library generation in secretion mode for the analysis of cell behaviour in microfluidic devices. (4) Antibodies of relevant therapeutic and complex formats produced from such a library setup were functionally active, indicating applicability to high content screening approaches The robust nature of these cells allowed high hit recovery rates even after prolonged incubation within microfluidic devices. (5) Target specific antibodies could be enriched in high throughput cellular screens by co-encapsulation of tumour cells or an autocrine target expression setup. Collectively, data presented herein illustrates the potential of combining CHO-based secretion libraries with microfluidics for high throughput and high content screening approaches.
Results
Optimized transduction for high monoclonality
Generating mammalian libraries by lentiviral transduction, a fine balance between sufficient integration and yet acceptable monoclonality is desired. To optimize both, experiments were carried out using a compatible pair of fluorescent proteins (ZsGreen and mCherry). High transducibility was obtained with higher MOI, however, monoclonality was compromised (Figure S1(a) and Figure S2(a)). Addition of non-coding stuffer lentivirus significantly reduced the polyclonality without compromising transducibility (Figure S1(b) and Figure S1(c)). Similar observations were made when non-coding stuffer lentivirus was mixed with lentiviruses of surface displayed antibodies (chosen due to the relative ease of detection by flow cytometry when compared to microfluidic methods; Figure S2(a,c)). In subsequent mAb mixture double staining as proxy for monoclonality, MOI 2 yielded 98% transduced clones of which approximately 16% showed display of both antibodies (Figure S2(a,b)). When applying a 2:3 (Figure S2(c,d)) and finally a 1:4 ratio of coding to non-coding lentivirus (Figure S3), ∼5% transient integration rate (as assessed by 3 dpt FACS analyses, data not shown) with >95% monoclonality in displaying cells were obtained (, Figure S3). Of note, subsequent analyses were conducted approximately five weeks post transduction to balance for both sufficient puromycin selection and retained display, before reduction was observed after >6 weeks (potentially by CMV promotor silencing [Citation38], see up to 60% non-displaying cells in Figure S4). Similar library optimization for transfection and monoclonality was also conducted applying PiggyBac or RMCE [Citation8,Citation16] approaches (data not shown).
Figure 2. Differentiating display levels of exemplary Bococizumab versus Cetuximab and sorting for display and target specificity. (a)-(d) Spiking of cells displaying anti-IgG1 stained Cetuximab (gate C) and Bococizumab (gate B) in ascending ratios of 1:10,000 to 1:10. Labels represent percentage from pre-gated populations (Figure S5(a)). Increasing prevalence of events in gate C match the higher Cetuximab to Bococizumab ratio. 1:10 spiked cells were stained with either anti-IgG (d) or target antigen EGFR and PCSK9, respectively (h). Percentage of events in gates B and C equals with prevalence of specifically target-binding cells in (h) Q1 and Q3, respectively, indicating clone-dependent display levels observed in (a)-(d). A 1:10,000 mixture was sorted for highest display and subsequently for EGFR specificity (please refer to Figure S4 for full gating strategy and sorting plots). (e) An analytical FACS post display sorting indicates enrichment for EGFR-positive Cetuximab displaying cells. Subsequent sorting for EGFR specificity yields highly enriched Cetuximab displaying cells detected via EGFR binding (f) or anti-IgG1 staining (g). (h) A 1:10 clone mixture generated with optimized conditions when stained for EGFR or PCSK9 binding yields ∼38% monoclonal versus ∼1.4% oligoclonal cells, hence ∼96% monoclonality in displaying cells.
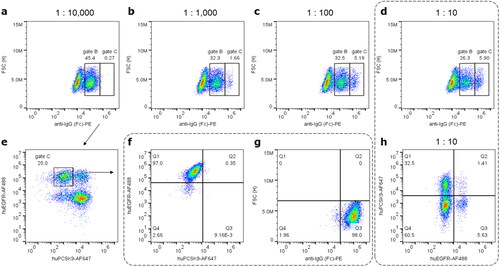
Sorting for manufacturability and target specificity
For confirmatory proof of concept studies, the mixture of surface displayed Cetuximab and Bococizumab, an antibody with reported manufacturability issues [Citation24,Citation39], was applied in spiking experiments. When stained for IgG display, two distinct populations were observed that matched the respective ratio applied () and correlated as well with specific binding when stained with the respective targets (). As a surrogate exemplifying the capacity to sort for superior manufacturability and target specificity, Cetuximab displaying cells could be enriched in a two-step FACS sorting for high display and subsequently for EGFR-specific binding from a 1:10,000 dilution to >97% ().
Stable secretion and gene recovery rates
Microfluidics data presented herein were recorded using a Sphere Fluidics Cyto-Mine [Citation40] or Berkeley Lights Beacon microfluidics device [Citation30]. Secretion rates were evaluated in bulk culture (), during single cells in-pen-analyses () and proven to be sufficient to support successful screens (). The high robustness of CHO cells is indicated by cell growth in pens () and high mAb PCR recovery rates even after prolonged time on Cyto-Mine device (). Viability and secretion over a period longer than 12 h represent a potential advantage over primary cells with fainting secretion ability for applications requiring longer assays times such as functional reporter assays.
Figure 3. Secretion, cell viability within and PCR recovery rates post microfluidic screening. (a) Bulk measured specific productivity of cells seeded at 0.2x106 vc/ml and 0.5x106 vc/ml, respectively. (b) Exemplary image of CHO cell secretion in pen on Beacon device and accumulated percentage of cells with confirmed secretion over time (c). (d) Cell growth in 8 representative pens over three days, indicating potential advantages over primary cells for longer-lasting functional screens. (e) Full mAb PCR recovery rates in relation to time on Cyto-Mine device. In comparison to primary cells after prolonged incubation (green and purple), CHO cells (blue circles) yield high recovery rates similar to hybridoma (red squares), indicating high retained viabilities and an overall robust screening system.
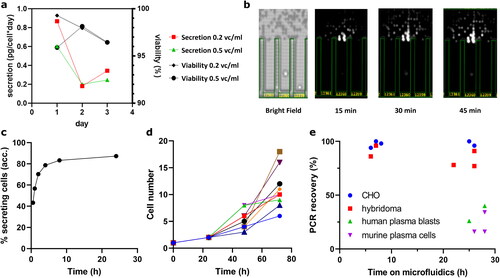
Figure 4. Therapeutic and complex formats can be produced in CHO library systems. (a) A stably integrated, secreted IgG1-VHH fusion targeting TMA and an TNFR showed simultaneous antigen binding in supernatant after bulk cultivation. 1, TMA loading; 2, quenching and baseline; 3, IgG-VHH binding from SN; 4, TNFR binding. (b) Similarly expressed, a tetra-specific VHH-SEED fusion bound all four targets, indicating the applicability of the system for complex formats including heterodimeric Fc fusions. Aligned to step 1. 1, loading of SEED SN; 2, baseline; 3, NKG2D; 4, IL-6R; 5, EGFR; 6, Her2.
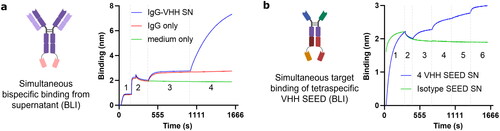
Secretion in therapeutic format
Microfluidic-assisted primary cell screening is restricted to formats that B cells produce, mainly to mouse or rat IgG, and sometimes limit screening options, for example when lacking a human Fc portion essential for the desired mode of action. In contrast to Phage or Yeast surface display limited to antibody fragment libraries, mammalian libraries may offer the advantage to clone and screen in the desired final therapeutic format. Two proof of concept complex antibody formats, secreted from bulk cultures, were evaluated in supernatant for simultaneous binding to all respective antigens using biolayer interferometry (BLI). A symmetric IgG-VHH bispecific antibody bound both a tumour mucin antigen (TMA) and a TNFR target protein (). Likewise, a heterodimeric tetraspecific VHH SEED fusion immobilized on AHC biosensors bound consecutively the four target proteins NKG2D, IL-6R, EGFR, and her2 (). Both examples provide evidence for functional expression of complex multispecific antibody formats [Citation53].
High throughput screening for cellular binding
Several recent publications describe primary cell microfluidic screens for cellular binding. To assess the applicability of herein presented CHO cells for cellular binding selection, Cetuximab secreting CHO cells were spiked 1:100 into non-secreting cells and screened for binding to EGFR positive A431 tumour cells co-encapsulated in droplets ( and Figure S5(a–c)). Two dispensed 96 well plates were evaluated for the presence of Cetuximab genes by PCR. Of 187 wells receiving droplets with at least one cell, 183 contained Cetuximab-secreting cells, equalling a hit rate of 98% (Figure S5(d)), hence a high single sorting round enrichment.
Figure 5. High throughput screening for binding to co-encapsulated target cells or in autocrine setup with increased sorting throughput. (a) Scheme representing a droplet and setup for sorting of cellular binding antibodies secreted from CHO cells (blue) to co-encapsulated target cells (orange) and detected via anti-IgG-488, and overlaid plot indicating a peak in cell-targeted fluorescence allowing sorting for green peak versus average fluorescence signals. (b) Dispensing plot of positive run with Cetuximab IgG1 secreting cells spiked 1:100 in non-secreting cells, co-encapsulated with EGFR-positive A431 cells and successfully sorted for cellular binding. (c) Sorting plot and statistics of interrogation of a cLC diversity against a tumour-specific mucin antigen via cellular binding on HeLa adenocarcinoma cells. Dispensed hits were subjected to Next Generation Sequencing. (d) Scheme of sorting in an autocrine setup. The CHO cell (blue) is both recombinantly expressing the antigen of interest and secreting one antibody candidate. Sorting is feasible for green peak fluorescence as in (a). (e) Dispensing plot and statistics for proof-of-concept autocrine cellular binding exemplarily to NKG2D by targeted anti-NKG2D or irrelevant anti-EGFR VHH-Fc binders applied in a 1:100 ratio. Note both a comparatively high throughput and high recovery rates indicating a robust screening system. For full sorting and dispensing plus gating information refer to Figure S5.
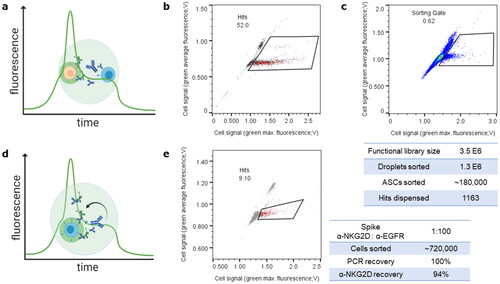
Applying this methodology, an antibody library was generated and screened for clones specific to a tumour-specific mucin antigen (TMA) present on a primary tumour cell. Libraries generated for TMA-specific common light chain (cLC) antibodies were at least 65% functional as assessed by 96 clones’ Sanger sequencing (data not shown). Following the sorting of 1.3 million droplets, 1163 droplets containing cells secreting full-length IgG specifically binding to target cells were dispensed and subjected to NGS analyses (). Assessing the resulting diversity yielded 8 strongly enriched clonal families that are subject to confirmatory binding studies. In addition to PoC spiking experiments, this indicates the applicability of library generation and successful rapid screening for cellular full-length binders.
As recombinant antibody-secreting cells allow integration of the respective antigen of interest as well, previously described autocrine selection systems were adapted to evaluate the potential of high throughput droplet-based microfluidic screens for autocrine cellular binding (). This approach supersedes the need for co-encapsulation and thus enlarges the screening throughput in otherwise Poisson-constricted systems. Cells secreting an anti-NKG2D VHH-Fc or irrelevant anti-EGFR VHH-Fc spiked in 1:100 ratio but all concomitantly surface-expressing NKG2D antigens were sorted for autocrine cellular binding (). Single dispensed droplets were evaluated for presence of anti-NKG2D VHH-Fc by PCR as before. Of 95 cell-carrying droplets, 89 contained desired binders, equalling a 94% hit rate. Both high PCR recovery () and enrichment rates presented here are indicative of a high CHO cell viability and robustness of the microfluidic screening system.
Enhanced downstream workflow by single-cell sub-cultivation
Primary plasma cells identified to secrete antibodies of desired profiles need to be subjected to extensive post-microfluidic processes such as NGS or single cell mAb gene PCR recovery plus transient reproduction to enable hit confirmation. As antibody-hit secreting CHO cells are robust, continuously growing and secreting antibodies already in the desired format, single cell cultivation was shown to yield antibodies in the supernatant that can be screened for specific binding to recombinant protein (Figure S6a) or target cell binding (Figure S6(b)). This requires comparatively low efforts, consumables or labware and can therefore enhance the workflow and output.
Discussion
Recent years have seen both the rise of microfluidics for antibody hit discovery as well as various reports on mammalian libraries for the discovery and optimization of antibody therapeutics. Within the past decade, CHO libraries have been generated by, among others, lentiviral delivery [Citation11], nucleases [Citation14], recombinases [Citation17,Citation18] and episomal approaches [Citation21].
In comparison to established screening technologies such as Phage and Yeast surface display, shared advantages are the preservation of full immune repertoires including both memory B and plasma cells of often precious diversities (obtained for example from human elite responders or autoimmune patients [Citation41], rodent disease models or extraordinary individual animal immunizations) as well as the option for repeated interrogation. Challenges include time- and labour-intensive library generation of comparatively smaller resulting diversities. Therefore, mammalian libraries were applied to focussed display libraries for antibody optimization [Citation14] and the combination of secretion libraries with microfluidics with similar library size or throughput, respectively. Library diversities of 50,000 to 1 million likely capture most of an immune diversity [Citation42,Citation43]. Generation of single-chain domain (dAbs) or common light chain (cLC) antibodies require lower efforts, while natively paired libraries can be generated via preceding microfluidic workflows [Citation44]. Another relevant parameter is the resulting monoclonality, i.e. guaranteeing one candidate molecule per cell to avoid labour-intensive downstream identification of hit candidates’ appropriate heavy and light chain pairing. Solutions commonly applied are reduced amount of coding DNA [Citation20] or lower coding DNA titrated into non-coding DNA, both resulting in lower transfection efficacy. While published for episomal approaches [Citation45], few reports on lentiviral libraries include information about optimization towards a high degree of monoclonality. We adopted the coding to non-coding titration approach to lentiviral delivery by choosing an MOI sufficient for effective transduction (MOI 2) and titrating in non-coding lentivirus for equal distribution of coding lentivirus leading to high monoclonality (Figures S1–S3). Resulting transient transduction of 5% was sufficient as in similar previous reports [Citation14,Citation20] and monoclonality of >90% in displaying cells suitable for selections. To our knowledge, this represents the first report on optimizing the essential attribute of monoclonality for lentivirus-based antibody libraries. Proceeding with envisioned experiments 3–5 weeks after selection proved optimal for sufficient selection of stably integrated and displaying cells but avoiding losing display over time (potentially due to promotor silencing upon prolonged cultivation) [Citation38].
Display of antibodies on mammalian cells can be utilized for optimization such as affinity maturation [Citation9,Citation15,Citation20–22] or enhancing manufacturability [Citation24]. Advantages over established display technologies include the option to screen in final therapeutic format and final host CHO with natural post-translational modifications and proper folding by vertebrate chaperones, leading to potentially less attrition [Citation3]. Display levels on the surface of mammalian cells can - due to constrained volume and resulting high local concentration - be indicative of manufacturability, in particular stability () and hydrophobicity (submitted data). Data presented herein on differential expression levels of Cetuximab when compared to Bococizumab confirm previous reports [Citation24] underline the opportunity to screen for manufacturability and target specificity early on. Flow cytometry allowed for rapid method optimization in display mode, and antibody secretion library generation benefitted from the application of similar conditions. Successful enrichment of target-specific antibodies indicated the utility of optimized library generation methods for all CHO libraries.
Secretion libraries represent a perfect match for combination with rapid microfluidic hit discovery methodologies. While as discussed above, library sizes fit well to throughput, also the compatibility of secreting with mostly mammalian target or reporter cell lines represents a distinct advantage. Data in represents, to our knowledge, the first report on the functional expression of therapeutically relevant complex IgG-VHH bispecific or heterodimeric tetraspecific VHH-SEED formats from a mammalian library system. Secretion rates were measured and sufficiently high for picoliter droplet or in-pen applications as proven by several examples presented herein. Stable secretion within microfluidic devices () could enable longer incubation times, for example, longlasting functional reporter assays in droplets or several consecutive assays without loss of function on Beacon workflows. High PCR recovery rates even after prolonged incubations () indicate robustness and high viability and represent a considerable advantage over primary cells screened on microfluidics.
While several groups report sorting mammalian libraries for specific binding followed by functional screening of hits in the supernatant [Citation20], microfluidics enable direct high throughput screening for specific cellular binding or function first selections [Citation6,Citation46]. Cellular binding of full IgG to tumour cells co-encapsulated in droplets was herein shown in spiking and library screens (). Inherent drawback of most high throughput droplet-based microfluidics systems is a dependency on Poisson-distributed co-encapsulation of secreting and target cells. We adapted the concept of previously reported autocrine systems [Citation47–50] combining VHH-Fc secretion with target membrane expression fully exploiting the high throughput capacities of droplet-based microfluidics. Sorting for autocrine cellular binding of approximately 720,000 cells in 7 h total assay time () to our knowledge represents the first example of high throughput autocrine cellular binding screening of CHO-based antibodies libraries in a droplet-based microfluidic workflow. Such an approach could facilitate identification of hits against co-expressed complex targets, e.g. membrane spanning receptors, omitting the need for recombinant target protein or membrane-like particles such as baculovirus particles but ensuring high throughput.
Finally, single cell cultivation and supernatant screening post-selection has been regularly reported for mammalian libraries [Citation20,Citation22] but is not applicable to primary plasma cells applied in microfluidics hit discovery that necessitate single cell mAb gene PCR recovery or NGS for hit identification. In contrast, a CHO cell secreting an antibody sorted for desired properties comprises a stable integration of a monoclonal antibody in the relevant therapeutic format. Therefore, CHO cell sub-cultivation could considerably facilitate downstream hit discovery workflows. The supernatant can be applied to confirmatory secondary screens and usage of monoclonal cell cultures rather than single cells as templates can simplify hit candidate sequence determination. To this end, a strong correlation of antibody concentration and cellular binding signals confirmed applicability of tool ASC culture supernatants for confirmatory BLI and FACS assays (Figure S6), similar to previous reports with other library systems [Citation20,Citation22].
In summary, mammalian libraries can cover full diversities of interesting immune repertoires and are not restricted to plasma or cultured memory B primary cell microfluidic screens. Such libraries allow repetitive interrogation and identification of diverse hit panels with differing desired MoA profiles. While data presented here represents displaying or secreting CHO cells, simultaneous display and secretion [Citation22,Citation25] or switchable systems seem desirable. Painting the broader picture, an integrated system of components presented and discussed here could utilize display for pre-enrichment of clones secreting antibodies with good manufacturability properties (via FACS)[Citation24] or functional antibodies (via anti-LC MACS) generating focussed libraries of smaller sizes suitable for subsequent microfluidic screening. Functional secretion of complex antibody formats, target co-expression and robust cells enabling long readout reporter assays could allow high throughput screens directly for the desired function such as effector cell activation or internalization, hence adding value to rapid microfluidic antibody hit discovery.
Materials and methods
Target and reporter cells
EGFR-expressing A431 epidermoid carcinoma (ATCC) cells and HeLa adenocarcinoma cells (ATCC) were cultivated in Dulbecco’s Modified Eagle’s Medium (DMEM high glucose, Sigma-Aldrich) with 10% FBS. Jurkat-GFP reporter cell line (NF-κB/Jurkat/GFP™ Transcriptional Reporter Cell Line) was sourced from SBI System Bioscience, Palo Alto, California, USA and cultured in RPMI 1640 medium (Sigma-Aldrich) with 10% FBS heat inactivated and 1x Glutamax.
Tool mAb ASC and library generation
Lentivirus production to support CHO-S transduction was carried out with the Lentivector Packaging Kit (System Biosciences, cat. LV500A-1) and PureFection Transfection Reagent (System Biosciences, cat. LV750A-1) on HEK 293TN cells, concentrated using the Lenti-X Concentrator (Takara Bio, cat. 631231) and finalized by titre estimation applying the Lenti-X p24 Rapid Titre Kit (Takara Bio, cat. 632200), all according to the manufacturer’s protocols. For transduction, 0.5 x 106 cells of >95% viability were added in complete culture media containing 1X TransDux, 1X Max enhancer and 1 M HEPES to 24 well plates, respective amount of virus described in results and supportive information sections was added, and the plates swirled to mix. After sealing and centrifugation at 2400 rpm, 32 °C for 90 min, the plates were carefully removed, and cells were mixed by aspirating at least 10 times with a pipette. After another centrifugation at similar conditions for 5 min, media was removed and replaced by 500 µl fresh media. Cells were cultivated without antibiotic selection for 3 days, with 5 µg/ml puromycin selection for 7 days and 10 µg/ml for another 7 days with media changes in regular intervals. Transient expression was evaluated by flow cytometry on day 3, with stable pools on days 24–35. Bococizumab or Cetuximab display was determined by staining with 5 µg/ml human PCSK9-ECD-His (Syngene, Bangalore, India) + 1:1000 anti-penta-His-AF647 (Qiagen, cat. 1019252) or directly labelled human EGFR-AF488 (Syngene, Bangalore, India), respectively, and washing with DPBS in between and after staining steps. Surface display was enabled by genetic C-terminal fusion of the PDGFR transmembrane domain as previously reported [Citation21]. As references in addition to transient expression based on pTT5 system, stable integration was also evaluated essentially as described by nucleofection of insert cassettes for PiggyBac transposase [Citation51], and recombinases such as Cre [Citation52] and Flp8.
Microfluidics-assisted ASC evaluation and sorting
Cyto-Mine-based sorting was conducted as previously described [Citation46] including ASC staining with 5 µM CellTracker™ Orange CMRA Dye (cat. C34551, ThermoFisher Scientific). During sorting of ASCs for cellular binding via co-encapsulation of target cells, a ratio of 1:6 million ASC to target cells was used to generate 2 million droplets. In autocrine cellular binding runs, 1 million target co-expressing ASCs were encapsulated in 2 million droplets to achieve a high degree of monoclonality. As all binders were recombinantly cloned to include a human IgG1 Fc portion, analysis of human IgG secretion or cellular binding was conducted with Goat Anti-Human IgG Fc-DyLight® 488 (cat. ab97003, Abcam, Cambridge, United Kingdom) detection antibody and Goat F(ab’)2 Anti-Human IgG - (Fab’)2 (DyLight® 594), pre-adsorbed (cat. ab98602, Abcam) or Alexa Fluor® 488 AffiniPure Fab Fragment Goat Anti-human IgG (H + L) (cat. 109-547-003, Jackson ImmunoResearch Europe LTD, Ely, United Kingdom). Maximum fluorescence signals were recorded applying ‘peak mode’ setting.
For functional screenings, EGFR surface-expression as well as EGFR-specific and irrelevant anti-NKG2D BiTE secreting cells were stained with 5 µM CellTracker™ Orange CMRA Dye (cat. C34551, ThermoFisher Scientific) and co-encapsulated with Jurkat-GFP reporter cells in the absence of costimulatory signals (anti-human CD28 antibody (clone 28.2, cat. 302902, BioLegend).
Single cell mAb gene recovery, subcloning and small-scale production
Positive droplets from Cyto-Mine were dispensed, treated for single cell antibody gene recovery PCR including subcloning and small-scale reproduction in HEK293 as previously described [Citation46].
Biolayer interferometry
Recombinant target proteins for BLI binding confirmation were internally produced at Merck Healthcare KGaA, Darmstadt, Germany (MTA, TMA, TNFR and EGFR) or sourced at Sino Biological as previously described [Citation53] (NKG2D, her2, IL6R). Kinetic measurements were performed on an Octet RED96 system (ForteBio, Pall Life Science, New York, USA). For analysis of IgG1-VHH fusion antibody, biotinylated TMA was loaded onto streptavidin biosensors for 180 s, followed by 60 s quenching (PBS pH 7.4; 1% skimmed milk powder; 1% BSA; 0.1% Tween-20; all Merck KGaA, Darmstadt, Germany, and 10 µg/ml Biocytin, Thermo Scientific cat. 28022), 120 s baseline in medium; association in IgG1-VHH containing supernatant for 600 s and finally 600 s association of TNFR at 100 nM concentration. Tetraspecific VHH-SEED antibody or non-binding control in supernatant were loaded onto anti-human Fc (AHC) biosensors for 300 s, a baseline in kinetics buffer (KB; PBS + 0.1% Tween-20 + 1% BSA) for 120 s was followed by 300 s association of 100 nM NKG2D antigen with similar association steps consecutively adding IL-6R, EGFR, and her2 (hence the last well contained all four target proteins). All steps were performed at 25 °C and 1000 rpm. Data were analyzed with ForteBio data analysis software 8.2 and 12.0 without fitting. Raw data normalized to end of loading are reported.
Sequence confirmation and analyses
Sanger sequencing was used for sequence analyses, and the resulting forward + reverse consensus sequences were analyzed with Geneious Prime software version 2022.1.1.
Authors contribution
AD, RG, SJ were involved in design and interpretation of the data; RGa, KK, HMM, DY, SPT conducted and interpreted experiments; AD drafted the paper; all authors revised it critically for intellectual content and approved the version to be published.
Supplemental Material
Download MS Word (1.6 MB)Acknowledgements
We thank Matthias Peipp for hints on NKG2D surface expression constructs, as well as Roland Schucht and Tom Wahlicht for methodology input and support. and were created using BioRender.com.
Disclosure statement
The authors are affiliated to Merck Healthcare KGaA, EMD Serono Inc. or Syngene International Limited, and confirm that there are no potential conflicts of interests.
Data availability statement
The data that support the findings of this study are available from the corresponding author, AD, upon reasonable request
Additional information
Funding
References
- Frenzel A, Schirrmann T, Hust M. Phage display-derived human antibodies in clinical development and therapy. MAbs. 2016;8(7):1177–1194.
- Krah S, Grzeschik J, Rosowski S, et al. A streamlined approach for the construction of large yeast surface display fab antibody libraries. Methods in Molecular Biology. 2018;1827:145–161.
- Wang B, DeKosky BJ, Timm MR, et al. Functional interrogation and mining of natively paired human V(H): V(L) antibody repertoires. Nat Biotechnol. 2018;36(2):152–155.
- Shin SW, Lee JS. CHO cell line development and engineering via site-specific integration: challenges and opportunities. Biotechnol Bioproc E. 2020;25(5):633–645.
- Javanmardi K, Chou CW, Terrace CI, Annapareddy A, Kaoud TS, Guo Q, Lutgens J, Zorkic H, Horton AP, Gardner EC, et al. Rapid characterization of spike variants via mammalian cell surface display. Mol Cell 2021; 81:5099–5111.e8.
- Wang Y, Jin R, Shen B, et al. High-throughput functional screening for next-generation cancer immunotherapy using droplet-based microfluidics. Sci Adv. 2021;7:3839–3850.
- Zhang H, Yea K, Xie J, et al. Selecting agonists from single cells infected with combinatorial antibody libraries. Chem Biol. 2013;20(5):734–741.
- Nehlsen K, Schucht R, da Gama-Norton L, et al. Recombinant protein expression by targeting pre-selected chromosomal loci. BMC Biotechnol. 2009;9(1):1–12.
- Eguchi A, Nakakido M, Nagatoishi S, et al. An epitope-directed antibody affinity maturation system utilizing mammalian cell survival as readout. Biotechnol Bioeng. 2019;116(7):1742–1751.
- Matreyek KA, Stephany JJ, Chiasson MA, et al. An improved platform for functional assessment of large protein libraries in mammalian cells. Nucleic Acids Res. 2020;48:1–12.
- Robertson N, Lopez-Anton N, Gurjar SA, et al. Development of a novel mammalian display system for selection of antibodies against membrane proteins. J Biol Chem. 2020;295(52):18436–18448.
- Smith ES, Zauderer M. Antibody library display on a mammalian virus vector: combining the advantages of both phage and yeast display into one technology. Curr Drug Discov Technol. 2014;11(1):48–55.
- Kim H, Kim JS. A guide to genome engineering with programmable nucleases. Nat Rev Genet. 2014;15(5):321–334.
- Parthiban K, Perera RL, Sattar M, et al. A comprehensive search of functional sequence space using large mammalian display libraries created by gene editing. MAbs. 2019;11(5):884–898.
- Parola C, Neumeier D, Friedensohn S, et al. Antibody discovery and engineering by enhanced CRISPR-Cas9 integration of variable gene cassette libraries in mammalian cells. MAbs. 2019;11(8):1367–1380.
- Zhou C, Jacobsen FW, Cai L, et al. Development of a novel mammalian cell surface antibody display platform. MAbs. 2010;2(5):508–518.
- Luo R, Zhao Y, Fan Y, et al. High efficiency CHO cell display-based antibody maturation. Sci Rep. 2020;10:1–10.
- Jia JY, Yu D, Long TX, et al. A novel and effective approach to generate germline-like monoclonal antibodies by integration of phage and mammalian cell display platforms. Acta Pharmacol Sin. 2022;43(4):954–962.
- Durrant MG, Fanton A, Tycko J, et al. Large-scale discovery of recombinases for integrating DNA into the human genome. bioRxiv. 2021; 2021.11.05.467528.
- Waldmeier L, Hellmann I, Gutknecht CK, et al. Transpo-mAb display: transposition-mediated B cell display and functional screening of full-length IgG antibody libraries. MAbs. 2016;8(4):726–740.
- Nguyen AW, Le KC, Maynard JA. Identification of high affinity HER2 binding antibodies using CHO fab surface display. Protein Eng Des Sel. 2018;31(3):91–101.
- Bowers PM, Horlick RA, Kehry MR, et al. Mammalian cell display for the discovery and optimization of antibody therapeutics. Methods. 2014;65(1):44–56.
- Seo H, Masuda H, Asagoshi K, et al. Streamlined human antibody generation and optimization by exploiting designed immunoglobulin loci in a B cell line. Cell Mol Immunol. 2021;18(6):1545–1561.
- Dyson MR, Masters E, Pazeraitis D, et al. Beyond affinity: selection of antibody variants with optimal biophysical properties and reduced immunogenicity from mammalian display libraries. MAbs. 2020;12(1):1829335.
- Zhou Y, Wang J, Zhou I, et al. Simultaneous expression of displayed and secreted antibodies for antibody screen. PLOS One. 2013;8:2–7.
- Shembekar N, Hu H, Eustace D, et al. Single-cell droplet microfluidic screening for antibodies specifically binding to target cells. Cell Rep. 2018;22(8):2206–2215.
- Fitzgerald V, Leonard P. Single-cell screening approaches for antibody discovery. Methods. 2017;116:34–42.
- Seah YFS, Hu H, Merten CA. Microfluidic single-cell technology in immunology and antibody screening. Mol Aspects Med. 2018;59:47–61.
- Mazutis L, Gilbert J, Ung WL, et al. Single-cell analysis and sorting using droplet-based microfluidics. Nat Protoc. 2013;8(5):870–891.
- Winters A, McFadden K, Bergen J, et al. Rapid single B cell antibody discovery using nanopens and structured light. MAbs. 2019;11(6):1025–1035.
- Fitzgerald V, Manning B, O'Donnell B, et al. Exploiting highly ordered subnanoliter volume microcapillaries as microtools for the analysis of antibody producing cells. Anal Chem. 2015;87(2):997–1003.
- Jones BE, Brown-Augsburger PL, Corbett KS, et al. LY-CoV555, a rapidly isolated potent neutralizing antibody, provides protection in a non-human primate model of SARS-CoV-2 infection. bioRxiv [Preprint]. 2020 Oct 9:2020.09.30.318972.
- Lim S, Chen B, Kariolis MS, et al. Engineering high affinity protein–protein interactions using a high-throughput microcapillary array platform. ACS Chem Biol. 2017;12(2):336–341.
- Zhai J, Yi S, Jia Y, et al. Cell-based drug screening on microfluidics. TrAC - Trends Anal Chem. 2019;117:231–241.
- Yanakieva D, Elter A, Bratsch J, et al. FACS-based functional protein screening via microfluidic co-encapsulation of yeast secretor and mammalian reporter cells. Sci Rep. 2020;10(1):1–13.
- Segaliny AI, Li G, Kong L, et al. Functional TCR T cell screening using single-cell droplet microfluidics. Lab Chip. 2018;18(24):3733–3749.
- Gérard A, Woolfe A, Mottet G, et al. High-throughput single-cell activity-based screening and sequencing of antibodies using droplet microfluidics. Nat Biotechnol. 2020;38(6):715–721.
- Sadelain M, Papapetrou EP, Bushman FD. Safe harbours for the integration of new DNA in the human genome. Nat Rev Cancer. 2011;12(1):51–58.
- Jain T, Sun T, Durand S, et al. Biophysical properties of the clinical-stage antibody landscape. Proc Natl Acad Sci U S A. 2017;114(5):944–949.
- Josephides D, Davoli S, Whitley W, et al. Cyto-mine: an integrated, picodroplet system for high-throughput single-cell analysis, sorting, dispensing, and monoclonality assurance. SLAS Technol. 2020;25(2):177–189.
- McDaniel JR, Ippolito GC, Georgiou G. Mapping the secrets of the antibody Pool. Nat Biotechnol. 2017;35(10):921–922.
- Kim S, Park I, Park SG, et al. Generation, diversity determination, and application to antibody selection of a human naïve fab library. Mol Cells. 2017;40(9):655–666.
- Erasmus MF, D’Angelo S, Ferrara F, et al. A single donor is sufficient to produce a highly functional in vitro antibody library. Commun Biol. 2021;4(1):350.
- Tanno H, McDaniel JR, Stevens CA, et al. A facile technology for the high-throughput sequencing of the paired VH:VL and TCRβ: TCRα repertoires. Sci Adv. 2020;6(17):eaay9093.
- Nguyen AW, Le K, Maynard JA. Engineering antibodies on the surface of CHO cells. Methods Mol Biol. 2020;2070:397–422.
- Gaa R, Menang-Ndi E, Pratapa S, et al. Versatile and rapid microfluidics-assisted antibody discovery. MAbs. 2021;13(1):1978130.
- Melidoni AN, Dyson MR, Mccafferty J. Selection of antibodies interfering with cell surface receptor signaling using embryonic stem cell differentiation. Methods Mol Biol. 2016;1341:111–132.
- Han KH, Gonzalez-Quintial R, Peng Y, et al. An agonist antibody that blocks autoimmunity by inducing anti-inflammatory macrophages. FASEB J off Publ Fed Am Soc Exp Biol. 2016;30:738–747.
- Zheng T, Xie J, Yang Z, et al. Antibody selection using clonal cocultivation of Escherichia colieukaryotic cells in miniecosystems. Proc Natl Acad Sci U S A. 2018 Jul 3;115(27):E6145-E6151.
- Robertson N, Lopez-Anton N, Gurjar SA, et al. Development of a novel mammalian display system for selection of antibodies against membrane proteins. J Biol Chem. 2020 Dec 25;295(52):18436–18448.
- Rajendra Y, Peery RB, Barnard GC. Generation of stable Chinese hamster ovary pools yielding antibody titers of up to 7.6 g/L using the piggyBac transposon system. Biotechnol Prog. 2016;32(5):1301–1307.
- Turan S, Zehe C, Kuehle J, et al. Recombinase-mediated cassette exchange (RMCE) - a rapidly-expanding toolbox for targeted genomic modifications. Gene [Internet. 2013;515(1):1–27.
- Yanakieva D, Pekar L, Evers A, et al. Beyond bispecificity: controlled fab arm exchange for the generation of antibodies with multiple specificities. MAbs. 2022;14(1):2018960.