Abstract
Carbon quantum dots (CQDs) were synthesized from blue honeysuckle (Lonicera caerulea) berry fruit extracts using a well-known, cost-effective, and environmental friendly hydrothermal process. The material was characterized using UV-vis spectroscopy, photoluminescence (PL), XPS, and TEM studies. The as-synthesized carbon dots exhibit excellent PL properties, with a quantum yield of ∼35.92%. CQDs vary in size from ∼2 nm to 9 nm. This study established the neuroprotective effects of CQDs against lipopolysaccharide (LPS)-induced human microglial cell model. LPS was found to induce cytotoxicity, reactive oxygen species, and pro-inflammatory cytokines interleukin (IL)-1β, IL-6, and tumour necrosis factor-α) and downregulated enzymatic antioxidants such as nuclear factor-erythroid factor 2-related factor 2 (Nrf2), superoxide dismutase, catalase, haem oxygenase (HO)-1, HO-2, and glutathione peroxidase, while CQDs treatment reversed LPS induced cytotoxicity, induced anti-inflammatory cytokines (IL-4, IL-10, and transforming growth factor β) and induce enzymatic antioxidants both at transcriptional and translational levels. The study suggested the potential role of CQDs prepared from Lonicera caerulea, as anti-inflammatory and antioxidative agents in neuroinflammatory and neurodegenerative diseases. In addition, CQDs could be exploited in various biomedical applications such as biosensing, drug delivery and tissue engineering.
Graphical Abstract
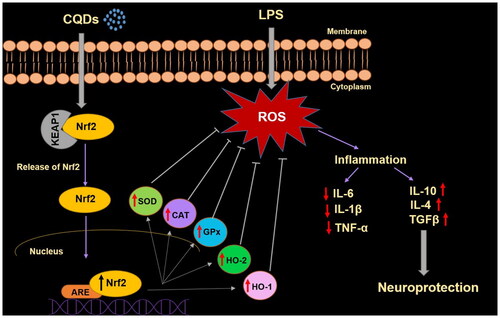
Carbon quantum dots (CQDs) were synthesized and characterized using honeyberries.
CQDs showed excellent antioxidant ability in LPS-induced HMC3 cells.
CQDs decreased DPPH and ABTS levels in comparable to controls.
Expressions of antioxidant enzymes were upregulated by CQDs.
CQDs reduced proinflammatory markers and upregulated anti-inflammatory markers.
CQDs can activate Nrf2/HO-1 signalling pathway.
Highlights
Introduction
Reactive oxygen species (ROS) under normal and controlled conditions mediate and regulate physiological functions of the body, such as microorganism removal and immune response regulation. Breaking the balance by over-accumulation of ROS and/or reduction of antioxidants can be deleterious and is termed ‘oxidative stress’. This oxidative stress (OS) is balanced with antioxidant systems to keep their level constant in living organisms, categorized as both enzymatic and non-enzymatic [Citation1]. The enzymatic antioxidant system includes superoxide dismutase (SOD), catalase (CAT), glutathione peroxidase (GPx), and glutathione reductase (GR), while the non-enzymatic antioxidants include glutathione (GSH), retinol (vitamin A), and ascorbic acid (vitamin C) [Citation2]. A substantial body of evidence supports a link between excess OS and the development of neurodegenerative disorders [Citation3]. Thus, OS is critical in the evolution of neurodegenerative illnesses [Citation4], and antioxidants are necessary for their prevention and/or treatment. It has been established that in chronic inflammatory situations, such as lipopolysaccharide (LPS)-induced inflammation, massive free radical formation outnumbers the endogenous antioxidant capacity of cells, resulting in OS and irreversible damage [Citation5]. Despite the fact that endogenous enzymes and antioxidants have antioxidant activity, their evolution is hampered by their selectivity and limited activity against ROS. As a result, there is a pressing need for us to create novel antioxidants to mitigate oxidative stress in an attempt to overcome these limits. Recent breakthroughs in this line have switched the focus to enzyme-like nanomaterials and nanozymes, which are more stable, less expensive, and easier to make, modify, and regulate than natural enzymes [Citation6].
In 2004, carbon dots (CDs) were first discovered by Xu et al. during the electrophoretic purification ofsingle-walled carbon nanotubes [Citation7]. They are spherical fluorescent nanoparticles with sizes of below 10 nm and are mainly composed of carbon, oxygen, hydrogen, and/or nitrogen in terms of the substrate used for their preparation [Citation8]. The thermal processes used in their production have included pyrolysis/combustion [Citation9], solvothermal [Citation10], hydrothermal [Citation11], and microwave [Citation12] techniques. Hydrothermal synthesis is the most effective, producing high quantum yield carbon quantum dots (CQDs) while improving control over size, composition, purity, energy, and cost-effectiveness. Carbon dots are thought to be better fluorescent materials than organic fluorophores and metal-based quantum dots because they have stable optical properties, high solubility in water, chemical stability, tuneable excitation and emission, low cytotoxicity, are easy to make and modify, and have antioxidant properties [Citation13]. Because of these properties, CQDs were also tested for their environmental applications [Citation14], biosensing, drug discovery, tissue engineering, and so on [Citation15–17]. CQDs also showed some potential roles as antitumor, antiviral, anti-inflammatory, and antituberculosis factors [Citation18]. Of note, reports have also shown their use as prospective military textiles using UV-protective/fluorescent cotton fabrics that have microbicidal activity [Citation19, Citation20].To date, most antioxidant research has concentrated on the radical scavenging activities of DPPH, hydroxyl (•OH) radicals [Citation21], superoxide anion radical (O2•−) [Citation22], ABTS [Citation23] and sensing of non-radical hydrogen peroxide (H2O2) [Citation24]. However, a few studies have shown that CQDs can protect against oxidative stress and inflammation by targeting enzymatic oxidants and inflammatory cytokines. CDs made with a carbonization product (from Radix Sophorae Flavescentis) showed anti-inflammatory and antioxidant effects in ethanol-induced gastric injury by lowering levels of nuclear factor kappa-light-chain-enhancer of activated B cells (NF-κB), tumour necrosis factor-α (TNF-α), interleukin (IL)-6, malondialdehyde (MDA), and inducible nitric oxide synthase (iNOS) and raising levels of SOD, CAT, GPx, and GSH [Citation25]. Similarly, CDs derived from Nelumbinis rhizomatis nodus carbonisata have anti-inflammatory and antioxidant properties in gastric ulcer-induced animal models [Citation26]. Prof. Huang’s group prepared p-phenylenediamine and ethylenediamine based CDs as nanocatalytic medicine with good antioxidant properties (peroxidase (POD)-, CAT-, and SOD-like) for inflammatory diseases of the liver [Citation27]. Carbon dots based on citric acid and glutathione have been shown to have a radical scavenging capacity in reducing LPS-induced inflammation in macrophages [Citation5]. Thus, antioxidant activities based on CQDs have a promising potential, yet green synthesized CQDs and their effects on LPS-induced OS and inflammation using a human microglial model (HMC3), are rarely described.
Herein, we exploited an underutilized blue suckle honeyberry (HB) (Lonicera caerulea) with massive antioxidant potential to synthesize CQDs in an attempt to combine the properties of both berry and CQDs as nano-oxidants against LPS-induced neuroinflammation in a unique experimental model (HMC3 cell line). This study demonstrates the effect of green synthesized CQDs on LPS-induced oxidative stress and inflammation. Concerning ROS mitigation, we evaluate the radical scavenging capacity of CQDs against DPPH and ABTS radicals. Meanwhile, the study establishes the antioxidant potential of CQDs by observing mRNA and protein expressions of enzymatic oxidants including SOD, CAT, haem oxygenase (HO)-1 and −2, GPx, and nuclear factor-erythroid factor 2-related factor 2 (Nrf2) in HMC3 cells. Further, the mRNA expressions of proinflammatory cytokines, including TNF-α, IL-6, IL-1β and anti-inflammatory cytokines, such as IL-10, IL-4, and TGFβ have also been verified. This study demonstrates the utility of CQDs as a capable free-radical scavenging system for eliminating ROSs in the LPS-induced oxidative stress disease model.
Materials and methods
Materials, chemicals, and instrumentation
The detailed information about the materials/chemicals and the instruments used in the study is given in supplementary Tables S1 and S2, respectively.
Preparation and characterization of CQDs from HB
Fresh honeyberries were cleansed, delicately rinsed in tap water to eliminate any dirt or soil residues, spread out on tissue paper sheets, and allowed to air dry. After that, cleaned honeyberry fruits (200 g) were immersed in 200 ml of double distilled water and ground for 1 min using a high-speed blender at room temperature, then filtered via a filter paper (Toyo Advantec Filter Paper-300 mm). The CQDs from HB extract (filtrate) was synthesized by a hydrothermal approach at 200 °C for 24 h. The solution thus obtained was filtered through a 0.22 μM filter, dialyzed, and lyophilized as reported previously [Citation14,Citation28]. The as-prepared CQDs were characterized using various tools. Quantum yield was measured on the previously established protocol [Citation14,Citation17]. The details have been provided in the supplementary file.
Viability assessment through cell counting kit (CCK)-8
The CCK-8 kit was used to test the CQDs’ cytotoxicity on HMC3 cells. HMC3 cells were grown in DMEM with 10% FBS and 1% anti-anti solutions and incubated at 37 °C in a 5% CO2 incubator The 5 x104 cells/well were plated in a 96-well plate and cultivated for 24 h. Each well received 100 μl of culture medium with gradient concentrations of HB-derived CQDs (3.90625, 7.8125, 15.63, 31.25, and 62.50 μg/mL). The CQD-containing media was discarded after 24 h. Cells were treated 100 μl of DMEM containing 10 μl of CCK for roughly 2 h. The transmittance for each well was recorded using a microplate reader at a 450 nm wavelength. After selecting the ideal concentration 62.50 μg/mL of CQDs, HMC3 was treated with 1 μg/mL in the presence and absence of 62.50 μg/mL of CQDs. And cell viability was observed using CCK assay as well as through phase contrast microscopy.
Radical scavenging assay
For radical scavenging activity DPPH and ABTS assays were used, and the detailed methodological approach has been provided in the supplementary data file [Citation29,Citation30].
Inhibition of ROS generation in LPS-treated HMC3 cells
LPS-induced ROS and its elimination by CQDs were spotted by DCFH-DA analysis. Intracellular fluorescence intensity is proportional to the amount of ROS generated in the LPS-activated HMC3 cells. Using a microplate fluorescence reader (EnSpire® Multimode Plate Reader, PerkinElmer, Inc., USA), fluorescence was viewed at 488 nm (excitation) and 525 nm (emission) wavelengths and the fluorescence intensity of the control, LPS-activated, and LPS + CQDs treated HMC3 cells was imaged by an Olympus fluorescence microscope (Olympus, Tokyo, Japan).
Total RNA isolation and real time analysis of cellular antioxidant enzymes, inflammatory, and anti-inflammatory genes in CQDs-treated HMC3 cells
Total RNA from control, LPS-treated and co-treated (LPS + CQDs) HMC3 cells was isolated using a commercial kit by following the information brochure. The isolated RNA was quantified using Nanodrop to check the quality of the isolated RNA. Quantified RNA (50 ng) was subjected to complementary DNA (cDNA) synthesis using kit materials: random hexamers (4 μl) and enzyme mix (2 μl) (TaKaRa Bio, Otsu, Japan). The synthesized cDNA (5.5 μl) was used for mRNA expression analysis using an ABI QuantStudio3 (Applied Biosystems, Foster City, CA) and SYBR Green Master Mix (TaKaRa Bio, Otsu, Japan) using the selected gene primer pairs (forward and reverse) as shown in Supplementary Table S3. The target gene expression levels were adjusted to that of β-actin mRNA.
Flow cytometry analysis of enzymatic antioxidants in CQDs-treated HMC3 cells
HMC3 (control, LPS-treated (1 μg/mL) and LPS (1 μg/mL) + CQDs (62.5 μg/mL) co-treated) cells were incubated for 24 h and harvested. After washing with DPBS, the cell pallet was treated with 4% formaldehyde (100 μl) solution for 10 min and washed with DPBS. Following this, cells were treated with 100 μl of triton (0.1%) for 10 min and washed. Then, 50 μl of staining solution containing 1 μl of primary antibody and 49 μl of DPBS was applied to each sample and incubated at 4 °C for 30 min before being washed with DPBS and centrifuged. The pellets were stained with a staining solution (50 μl, 1 μl of PE secondary antibody + 300 μl of DPBS), then incubated at 4 °C for 30 min and washed. Finally, 400 μl of DPBS was added to round bottom tubes and used for FL2 log reading with a flow cytometer.
Statistical analysis
GraphPad Prism software (version 9.4) was used for statistical analysis, and comparisons were made using a one-way analysis of variance. Studies were replicated in a set of three. The level of significance was set at *p < 0.05, **p < 0.01, ***p < 0.001, ****p < 0.0001.
Results
CQDs characterization
The morphology and internal microstructure of as-synthesized CQDs were investigated by transmission electron microscopy (TEM) and high-resolution TEM (HR-TEM). The TEM images in show that the CQDs have a practically spherical shape and homogeneous dispersibility with a size distribution of ∼2 nm to 9 nm. Moreover, as shown in , the estimated value of lattice spacing is 0.24 nm, which corresponds to the (100) facet of graphitic carbon and represents the graphitic construction of the produced CQDs. Likewise, shows the well-resolved lattice fringes of the honeyberry-derived CQDs [Citation20,Citation31].
Figure 1. (A, B) Low-resolution TEM images of CQDs showing spherical morphology and dispersed nature; (C) high resolution TEM image of CQDs, showing spherical morphology, lattice fringes and graphitic nature; and (D) Zoom HRTEM image of CQDs showing clear lattice fringes.
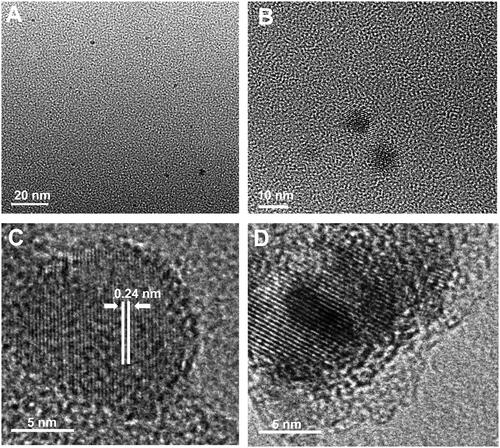
The FTIR spectrum shows the functional groups on the surface of CQDs compared to blank. As shown in , a wide absorption peak at around 3290.7 cm−1 corroborated the presence of stretching vibration of O − H [Citation14]. A tiny band located at 2927.4 cm−1 was ascribed to the symmetric/asymmetric stretching vibrations of − CH2 − [Citation5]. The peaks at 1689.3 and 1563.8 cm−1 were due to the presence of vibrational stretching of C = O and C = CO, respectively [Citation32]. The peak at 1038 cm−1 was identified as a vibrational stretch of C − O−C [Citation32]; the peaks at 1397.3 cm−1 and 1303.5 cm−1 were ascribed to C − N and C − O stretching vibration mode, respectively [Citation10,Citation33,Citation34]. The peak at 878.79 cm−1 could be ascribed to C–H bending vibration () [Citation14].
Figure 2. (A) FTIR spectrum of blank and CQDs (B) a Full XPS spectrum. The high-resolution deconvoluted XPS peaks of CQDs for (Ba) C1s, (Bb) O1s, and (Bc) N1s.
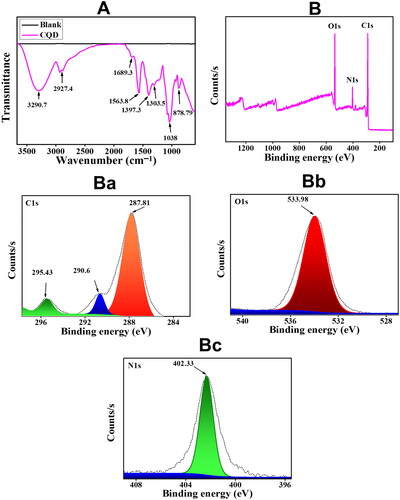
XPS analysis of as-synthesized CQDs from HB extract is shown in . The survey scan of CQDs XPS in shows that the CQDs are primarily composed of carbon (C), oxygen (O) and nitrogen (N), with C1s (67.58 atomic percent), O1s (22.43 atomic percent), and N1s (10.0 atomic percent) being the most common elements. There was no indication of any other residual ingredients, indicating the purity of CQDs. Deconvoluted images of C1s show the presence of three peaks at 287.81 eV, 290.6 eV, and 295.43 eV, respectively (). The presence of a peak at 295.43 eV signified the sp2 carbon structure [Citation35], while peaks at 287.81 eV, 290.6 eV ascribed to the presence of C = O and COOH, respectively [Citation14,Citation32]. O1s deconvolution showed a single peak at 533.98 eV which can be assigned to C − OH/C − O−C () [Citation32]. The N1s XPS spectrum deconvolution shows the presence of a single peak at 402.33 eV which can be assigned to pyrrolic nitrogen [Citation5] (). The results of the FTIR spectrum complement those of the XPS spectrum, implying that CQDs have a lot of functional groups on their surface.
Optical properties of CQDs
The optical properties of fluorescent CQDs in distilled water were recorded using an ultraviolet-visible (UV-vis) spectrum and photoluminescence spectra. The UV − vis absorption spectrum in has a stronger peak at 275.9 nm and a minor peak at 300.2 nm which can be assigned to the n − π* transition and π − π* transition of C = O and C = C, respectively of the as-prepared CQDs, implying the intricate structures and energy levels (random) of CQDs [Citation32,Citation36]. presents the PL emission spectra of the CQDs obtained by gradually increasing the excitation wavelength from 300 to 490 nm by an increment of 10 nm. The spectra display a typical excitation wavelength-dependent phenomenon, a characteristic feature of CQDs [Citation37]. When excited at 360 nm, the CQDs showed very strong PL in the range of 300 nm to 490 nm, with the maximum located at around 436.02 nm. Using quinine sulphate as a base, the fluorescent quantum yield was found to be 35.92%. To examine the colloidal stability of CQDs, the zeta (ζ) potential of CQDs was computed and found to be -11 mV, indicating the existence of a negative charge on the surface of CQDs. The negative value represents a repulsive electrostatic force; the stronger the negative value, the greater the repulsion and the greater the colloidal stability [Citation20].
Effect on cell viability
Following a 24-h exposure to CQDs, we observed no significant difference in the viability of HMC3 (). The viability was observed to be ∼ 100%, so we used 62.5 μg/mL concentrations for the mRNA and protein experiments. The effects of CQDs at 62.5 μg/mL were also evaluated in the presence of LPS. As shown in , LPS treatment decreased the viability of HMC3 cells while the reducing trends were significantly less in HMC3 cells treated with CQDs and LPS. Also, images taken through phase contrast microscopy showed larger rounded structures with an amoeboid shape in LPS-treated HMC3 cells, as illustrated in . However, similar structures are less common in HMC3 cells treated with both LPS and CQDs. Previous research has also demonstrated morphological changes in microglial cells after LPS treatment and explained how LPS treatment caused ROS formation in HMC3 cells after 24 h [Citation38]. These findings suggest that CQDs protect LPS-stimulated HMC3 cells.
Figure 4. (A) Cell viability assay of human microglial cells (HMC)3 in the presence of CQDs. (B) Effect of CQDs on LPS-induced HMC3 cells. Radical scavenging activity of (C) DPPH and (D) ABTS radials. LPS, lipopolysaccharide; CQDs, carbon quantum dots; DPPH, 2,2-diphenyl-1-picrylhydrazyl; ABTS, 2,2'-azino-bis (3-ethylbenzothiazoline-6-sulfonic acid).
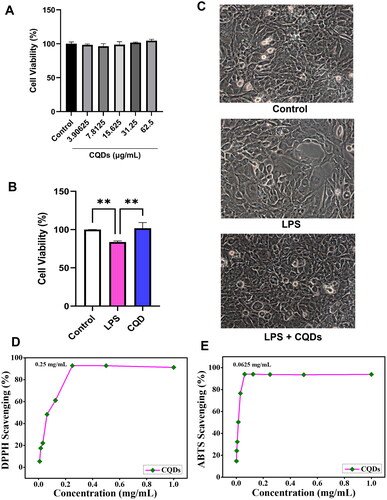
DPPH and ABTS scavenging by CQDs
The antioxidant study evaluates the capability of the material to scavenge free radicals and neutralize them. The radical scavenging ability of the as-prepared CQDs was evaluated using DPPH and ABTS radicals. shows the DPPH radical scavenging ability of the CQDs using UV–Visible absorption spectrophotometry. The IC50 value of CQDs was found to be 0.25 mg/mL. At 1 mg/mL, the DPPH (%) scavenging activity of CQDs was found to be 91.2%, which was found to be comparable to the standard ascorbic acid. The IC50 value of the CQDs for ABTS was observed to be 0.625 mg/mL (). At 1 mg/mL concentration, the ABTS (%) scavenging ability of CQDs was found to be 93.8%, comparable to standard ascorbic acid.
HB-derived CQDs prevent cellular ROS level in LPS-induced HMC3 cells
Since oxidative stress is linked to inflammation, this study looked at whether CQDs may reduce LPS-induced ROS in HMC3 cells. LPS treatment enhanced ROS levels in HMC3 cells, as seen in , but ROS content was dramatically reduced following CQD treatment in LPS-activated HMC3 cells. Furthermore, without CQDs treatment, cell fluorescent intensity was higher in the LPS-only treated group, but a lower fluorescent intensity was observed in HMC3 cells treated with LPS and CQDs. ().
Figure 5. Evaluation of intracellular ROS by DCFDA assay. (A) Effects of CQDs on LPS-activated HMC3 cells. (B) The fluorescent microscopic images of control, LPS-activated and LPS + CQDs co-treated cells. The data are mean ± SD values of triplicates from a representative of three experiments. **** signifies p value < 0.0001. ROS: reactive oxygen species; LPS, lipopolysaccharide; CQDs, carbon quantum dots; DCFDA: 2',7'-dichlorodihydrofluorescein diacetate.
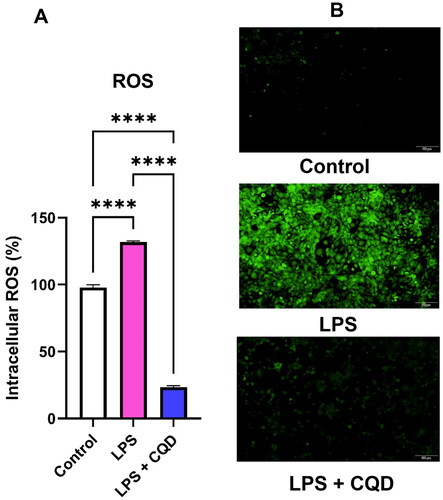
CQDs upregulate the mRNA and protein expressions of antioxidant enzymes in LPS-induced HMC3 cells
To further evaluate the impact of CQDs on the enzymatic oxidant system, the transcriptional and translational levels of Nrf2, HO-1, HO-2, SOD, CAT, and GPx were examined. Except for GPx, the mRNA expressions of Nrf2, HO-1, HO-2, SOD, and CAT were shown to be downregulated in LPS-treated cells but were reversed in LPS and CQD co-treated cells (). The protein expressions of Nrf2, HO-1, HO-2, SOD, and CAT, as expected, confirm the mRNA results and reveal considerably elevated expressions in HMC3 cells co-treated with LPS and CQDs (). Notably, GPx protein expression was considerably increased in the CQDs-treated group ().
Figure 6. Effects of CQDs on intracellular antioxidant defense system in LPS-stimulated HMC3 cells. Control is representing HMC3 cells without any treatment. The relative mRNA expressions levels of (A) nuclear factor erythroid 2–related factor 2 (nrf2), (B) haem oxygenase (HO)-1, (C) HO-2, (D) superoxide dismutase (SOD), (E) catalase (CAT), (F) glutathione peroxidase (GPx). The symbols **, ***, ****signify p value < 0.01 < 0.001, < 0.0001, respectively. The expressions were normalized to β actin.
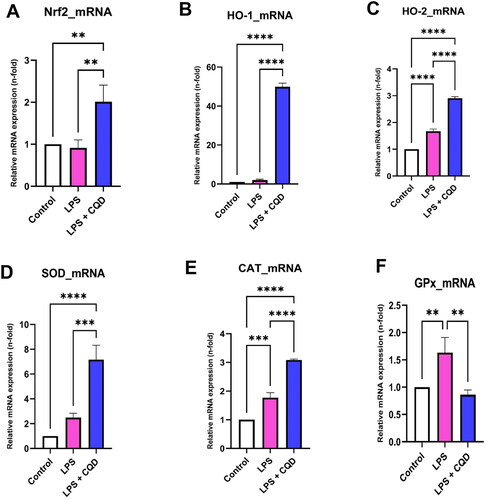
Figure 7. Effects of CQDs on protein expression profiles of antioxidant defense system in LPS-stimulated HMC3 cells using flow cytometry. Control is representing HMC3 cells without any treatment. The relative protein expressions levels of (A) nuclear factor erythroid 2–related factor 2 (nrf2), (B) haem oxygenase (HO)-1, (C) HO-2, (D) superoxide dismutase (SOD), (E) catalase (CAT), (F) glutathione peroxidase (GPx). The symbols *, **, ***, ****signify p value <0.05, < 0.01 < 0.001, < 0.0001, respectively.
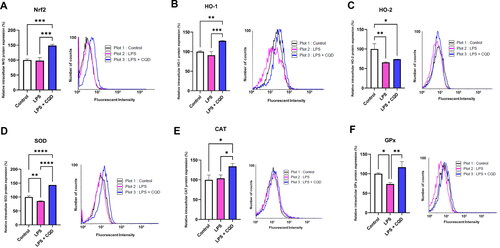
CQDs downregulate expression of inflammatory and upregulate anti-inflammatory genes expressions
We next investigate the effects of CQDs on the production of inflammatory and anti-inflammatory cytokines in LPS-induced HMC3 cells. Our results show that after LPS treatment, mRNA expression of proinflammatory cytokines like IL-1β, TNF-α, and IL-6 increased significantly. However, after CQD treatment, these increased mRNA expressions were reduced (). Subsequently, mRNA expressions of anti-inflammatory cytokines, including IL-4, IL-10, and TGFβ were down regulated in LPS-stimulated HMC3 cells, while these expressions were upregulated in LPS-stimulated HMC3 cells treated with CQDs ().
Figure 8. Effects of CQDs on mRNA expression levels of inflammation-related genes. (A-C) The mRNA expression levels of pro-inflammatory genes (A) interleukin (IL)-1β, (B) tumour necrosis factor-alpha (TNF-α), (C) IL-6. The mRNA expression levels of genes related to anti-inflammation, including (D) IL-10, (E) IL-4, and (F)transforming growth factor beta (TGFβ). Control is representing HMC3 cells without any treatment. The symbols *, **, ***, ****signify p value <0.05, < 0.01 < 0.001, < 0.0001, respectively. The expressions were normalized to β actin.
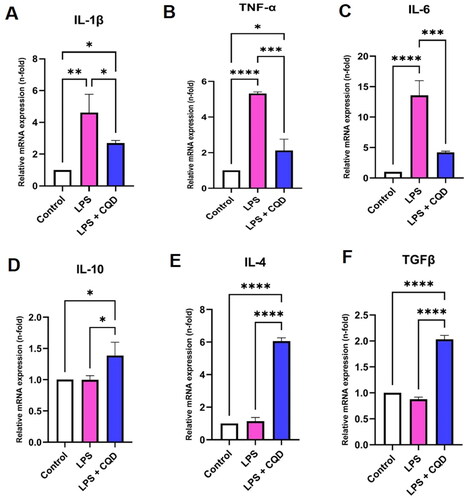
Discussion
Lonicera caerulea is a honeysuckle plant with a long development history. It is known to be rich in bioactive substances [Citation39]. The major constituents in honeyberry with the greater proportion, ranging between 36% and 51% observed for anthocyanins- glycosides, peonidin, petunidin and cyanidin. The main anthocyanin identified was cyanidin-3-O-glucoside (C3G) [Citation40]. C3G has been already reported to play an essential role in the prevention of neurodegenerative diseases [Citation41,Citation42]. In light of the significance of the berry, this work used honeyberry for the production of CQDs and assessed their capacity to modify inflammatory markers, free radicals, and enzymatic oxidants in an LPS-induced HMC3 in vitro model.
Over the past number of years, particular focus has been placed on materials with luminous characteristics. The synthesis of photoluminescent CQDs from biological resources and their use in biomedical applications have been extensively discussed in the literature [Citation14,Citation17].
In this study, the antioxidant, and anti-inflammatory potentials of HB-derived CQDs were demonstrated in an LPS-activated HMC3 cell line. CQD treatment reduced LPS-induced ROS levels in HMC3 cells as demonstrated by augmented expressions of antioxidant enzymes such as Nrf2, SOD, CAT, GPx, HO-1 and HO-2, implying the possible activation of the Nrf2/HO-1 signalling pathway. The radical scavenging potential (IC50) of CQDs against DPPH and ABTS radicals was found to be 0.25 mg/mL and 0.625 mg/mL, respectively. At the same time, CQDs could reduce the mRNA expression of proinflammatory cytokines like TNF-α, IL-1β, and IL-6 while increasing the expression of anti-inflammatory cytokines like IL-4, IL-10, and TGFβ in HMC3 cells, reducing the degree of damage caused by LPS-induced OS.
An abnormal rise in ROS often accompanies OS, which can result in neurodegenerative disorders [Citation43,Citation44]. Enzyme-mimicking nanomaterials for antioxidative therapy are emerging as promising stars to treat OS-related diseases [Citation20]. In this line, CD-based studies have been demonstrated to have protective potential against OS [Citation45,Citation46]. Inorganic nanoparticles such as manganese dioxide and cerium oxide, among others, reduce ROS levels and protect the in vitro system from oxidative damage [Citation47,Citation48]. Owing to these properties, these nanoparticles are getting significant attention as nanozymes. Depending on whether they can get rid of free radicals or make them, nanozymes can be either antioxidants or pro-oxidants.
In our study, exposure of HMC3 cells to LPS resulted in an increase in ROS generation. Preliminary analysis of ROS reduction by CQD treatment was evaluated by DCFH-DA assay, which is a widely used method to evaluate the ROS level [Citation23], but not without limitations [Citation49]. Moreover, as discussed previously, the copious functional groups, such as hydroxyl, amine, and carboxyl groups, with properties of electron acceptor/donor over the surface of CQDs endow them with good ROS scavenging activity [Citation50,Citation51]. CQDs are less explored and more suitable candidates for antioxidant studies due to their minimal toxicity [Citation11,Citation33]. This DCFH-DA assay does not provide any indication to specifically these species, and further exploration will be required. Moreover, the enzymatic antioxidant defense mechanisms, which are mediated through SOD, CAT, and GPx, are the most important mechanisms against OS [Citation27]. The mutations in SOD, CAT, and GPx genes or relative enzyme deficiency can lead to neurodegenerative disorders due to impaired antioxidant protection mechanisms.
SOD is the front-line barrier for ROS among enzymatic antioxidants, catalyzing the dismutation of the powerful endogenous radical, superoxide anion, and converting it to H2O2 and O2. At high H2O2 concentrations, CAT further metabolizes H2O2 into water and oxygen, whereas GPx is essential in removing H2O2 at relatively low concentrations [Citation52]. In the current study, CQDs significantly increased the expression of SOD and CAT at transcriptional and translational levels. Notably, the mRNA expression of GPx was downregulated following CQDs treatment. However, a significant rise can be seen at the GPx protein level (). The plausible reasons could be the lower expression of the GPx gene at the time of analysis or most of transcription product has been translated to the protein molecule. These results suggest that HB-derived CQDs could exert their antioxidant and/or radical scavenging activities through the regulation of the enzymatic antioxidant system, thus preventing the formation of the free radicals or ROS originated in LPS-activated HMC3 cells. This hypothesis is supported by the recent findings on the in vitro antiradical and antioxidative activities of CDs [Citation21,Citation23,Citation25,Citation26].
Furthermore, Nrf2, a transcription factor, acts as an important regulator, protecting cells from OS. This factor has been reported to prevent a number of OS-based disorders such as Alzheimer’s disease, cancer, and hypertension [Citation53]. Under homeostatic conditions, it is found in the cytoplasm coupled to the inhibitory protein Kelch-like ECH-associated protein 1 (Keap1) (Scheme 1). Under OS, however, Nrf2 is liberated from Keap1, and translocated into the nucleus, where it combines with antioxidant response elements (ARE), to regulate the transcription of antioxidant genes such as HO-1 (Scheme 1) [Citation54]. Nrf2 induces the HO-1 gene by increasing mRNA and protein expressions and it is one of the classic Nrf2 regulated genes which is widely used in numerous in vitro and in vivo studies. HO-1 is known to play important role by regulating levels of OS and apoptotic cell death [Citation55]. However, it has recently reported that HO-1 can also have immunomodulatory and anti-inflammatory properties [Citation56]. In this work, CQDs demonstrated their antioxidant effects by involving HO-1 as an antioxidant that regulated ROS levels and resulted in anti-inflammatory capabilities, resulting in neuroprotection (Scheme 1). Owing to the antioxidant and anti-inflammatory properties of HO-1, Nrf2/HO-1 is recognized as important signalling pathway dealing with OS.
Generally, haem oxygenase consists of two isoenzymes, inducible form (HO-1) and constitutively expressed form (HO-2), which share sequence homology and similar structures [Citation57]. Though little is known about HO-2, this constitutionally expressed form is highly expressed in the brain, testes, and neural tissues [Citation58]. In this study, the expressions of Nrf2 and downstream protecting genes, including HO-1 and HO-2 were reduced in the presence of LPS. However, CQDs treatment reversed the expression both at mRNA () and protein levels (). In a prior study, the role of gold nanoparticles (AuNPs) in inducing HO-1 production via Nrf2 activation was established [Citation59]. Noticeably, CDs have shown a high ability to pass the blood-brain barrier and target abnormalities such as brain cancer glioma tissue [Citation60]. Thus, these fluorescent carbon dots/CQDs could be utilized as nanomedicine in the near future.
LPS, a bacterial endotoxin, increases the production of ROS and pro-inflammatory mediators such as ILs and TNF-α in HMC3 cells, resulting in inflammatory reactions and cellular damage in microglial cells [Citation38,Citation61]. Thus, lowering LPS-inducible inflammatory mediators and cytokines is viewed as a significant tactic for preventing a range of inflammation-related disorders caused by microglial activation. In the present study, CQDs significantly reduced the levels of intracellular ROS and pro-inflammatory mediators, including IL-1β, TNF-α, and IL-6 in LPS-induced HMC3 cells. At the same time, a significant rise can be seen in mRNA expressions of anti-inflammatory cytokines, IL-4, IL-10, and TGFβ, indicating the antioxidant and anti-inflammatory properties of CQDs that could lead to neuroprotection in LPS-induced HMC3 cells. The role of CQDs in alleviating ROS and inhibiting activation of inflammatory pathway has recently been described [Citation5].
Conclusions
Overall, a one-step hydrothermal technique employing HB as the sole precursor resulted in novel luminous CQDs with inherent bioactivity, good biocompatibility, excellent QY, and low toxicity. To our knowledge, this is the first time that the neuroprotective effect of HB-derived CQDs has been proven in an LPS-induced human microglial cell model by focussing on ROS alleviation as demonstrated by DPPH and ABTS radical scavenging and upregulation of enzymatic oxidant expressions, including SOD, CAT, GPx, Nrf2, HO-1, and HO-2. Concurrently, CQDs inhibited LPS-induced inflammation in HMC3 cells by downregulating TNF-α, IL-1β, and IL-6 (proinflammatory cytokines) and increasing IL-4, IL-10, and TGFβ (anti-inflammatory cytokines). These findings suggest CQDs are a safe and effective nanomedicine that has good potential for therapeutic applications in the treatment of LPS-induced oxidative stress and inflammation in human microglial and other models. However, animal studies are warranted to evaluate their full therapeutic potential.
Author contributions
Hae-Jeung Lee and Anshul Sharma conceived the idea. Hae-Jeung Lee, Anshul Sharma, and Sanjay designed the experiments. Anshul Sharma and Sanjay performed the laboratory experiments, analyzed data and prepared the figures, and wrote the original draft. Hae-Jeung Lee revised the manuscript and supervised the study. All authors contributed to formal analysis and data curation and reviewed/edited the manuscript. All authors have read and agreed to the published version of the manuscript.
Supplemental Material
Download MS Word (28.3 KB)Disclosure statement
No potential conflict of interest was reported by the author(s).
Data availability statement
The data that support the findings of this study are available from the corresponding author, H-J. L., upon reasonable request.
Additional information
Funding
References
- Li J, O W, Li W, et al. Oxidative stress and neurodegenerative disorders. Int J Mol Sci. 2013;14(12):24438–24475.
- Ashrafizadeh M, Mohammadinejad R, Kailasa SK, et al. Carbon dots as versatile nanoarchitectures for the treatment of neurological disorders and their theranostic applications: a review. Adv Colloid Interface Sci. 2020;278:102123.
- Yusuf M, Khan M, Robaian MA, et al. Biomechanistic insights into the roles of oxidative stress in generating complex neurological disorders. Biol Chem. 2018;399(4):305–319.
- Uddin M, Kabir M. Oxidative stress in Alzheimer’s disease: molecular hallmarks of underlying vulnerability. In: Ashraf, G., Alexiou, A. (eds) Biological, Diagnostic and Therapeutic Advances in Alzheimer’s Disease. 2019; pp: 91–115.Springer, Singapore. https://doi.org/10.1007/978-981-13-9636-6_5
- Wang H, Zhang M, Ma Y, et al. Carbon dots derived from citric acid and glutathione as a highly efficient intracellular reactive oxygen species scavenger for alleviating the lipopolysaccharide-induced inflammation in macrophages. ACS Appl Mater Interfaces. 2020;12(37):41088–41095.
- Zhao H, Zhang R, Yan X, et al. Superoxide dismutase nanozymes: an emerging star for anti-oxidation. J Mater Chem. B. 2021;9(35):6939–6957.
- Xu X, Ray R, Gu Y, et al. Electrophoretic analysis and purification of fluorescent single-walled carbon nanotube fragments. J Am Chem. Soc. 2004;126(40):12736–12737.
- Pal T, Mohiyuddin S, Packirisamy G. Facile and green synthesis of multicolor fluorescence carbon dots from curcumin: in vitro and in vivo bioimaging and other applications. ACS Omega. 2018;3(1):831–843.
- Jia J, Lin B, Gao Y, et al. Highly luminescent N-doped carbon dots from black soya beans for free radical scavenging, Fe3+ sensing and cellular imaging. Spectrochim Acta A Mol Biomol Spectrosc. 2019;211:363–372.
- Guo Y, Li T, Xie L, et al. Red pitaya peels-based carbon dots for real-time fluorometric and colorimetric assay of Au(3+), cellular imaging, and antioxidant activity. Anal Bioanal Chem. 2021;413(3):935–943.
- Sachdev A, Gopinath P. Green synthesis of multifunctional carbon dots from coriander leaves and their potential application as antioxidants, sensors and bioimaging agents. Analyst. 2015;140(12):4260–4269.
- Swathi R, Bhagavanth Reddy G, Rajkumar B, et al. Rapid fabrication of carbon dots from babul seed powder as green precursor: antioxidant activity and multicolor imaging. Mater Today Proc. 2021;43:1389–1397.
- Zuo P, Lu X, Sun Z, et al. A review on syntheses, properties, characterization and bioanalytical applications of fluorescent carbon dots. Microchim Acta. 2016;183(2):519–542.
- Ahmed HB, Emam HE. Environmentally exploitable biocide/fluorescent metal marker carbon quantum dots. RSC Adv. 2020;10(70):42916–42929.
- Molaei MJ. Carbon quantum dots and their biomedical and therapeutic applications: a review. RSC Adv. 2019;9(12):6460–6481.
- Janus Ł, Radwan-Pragłowska J, Piątkowski M, et al. Smart, tunable cqds with antioxidant properties for biomedical applications—ecofriendly synthesis and characterization. Molecules. 2020;25:736.
- Emam HE. Clustering of photoluminescent carbon quantum dots using biopolymers for biomedical applications. Biocatal Agric Biotechnol. 2022;42:102382.
- Emam HE, Ahmed HB. Antitumor/antiviral carbon quantum dots based on carrageenan and pullulan. Int J Biol Macromol. 2021;170:688–700.
- Emam HE, El-Shahat M, Hasanin MS, et al. Potential military cotton textiles composed of carbon quantum dots clustered from 4–(2, 4–dichlorophenyl)–6–oxo–2–thioxohexahydropyrimidine–5–carbonitrile. Cellulose. 2021;28:9991–10011.
- Ahmed HB, Abualnaja KM, Ghareeb RY, et al. Technical textiles modified with immobilized carbon dots synthesized with infrared assistance. J Colloid Interface Sci. 2021;604:15–29.
- Dehvari K, Chiu S-H, Lin J-S, et al. Heteroatom doped carbon dots with nanoenzyme like properties as theranostic platforms for free radical scavenging, imaging, and chemotherapy. Acta Biomater. 2020;114:343–357.
- Li Y, Li W, Yang X, et al. Salvia miltiorrhiza-derived carbon dots as scavengers of reactive oxygen species for reducing oxidative damage of plants. ACS Appl. Nano Mater. 2020;4(1):113–120.
- Dong C, Wang S, Ma M, et al. Inhibition of oxidative stress in vivo through enzyme-like activity of carbon dots. Appl Mater. Today. 2021;25:101178.
- Costas-Mora I, Romero V, Lavilla I, et al. In situ photochemical synthesis of fluorescent carbon dots for optical sensing of hydrogen peroxide and antioxidants. Talanta. 2015;144:1308–1315.
- Hu J, Luo J, Zhang M, et al. Protective effects of Radix Sophorae Flavescentis carbonisata-based carbon dots against ethanol‐induced acute gastric ulcer in rats: anti-Inflammatory and antioxidant activities. Int J Nanomed. 2021;16:2461.
- Luo J, Hu J, Zhang M, et al. Gastroprotective effects of Nelumbinis Rhizomatis nodus-derived carbon dots on ethanol-induced gastric ulcers in rats. Nanomedicine. 2021;16(19):1657–1671.
- Kong B, Yang T, Cheng F, et al. Carbon dots as nanocatalytic medicine for anti-inflammation therapy. J Colloid Interface Sci. 2022;611:545–553.
- Park M, Sharma A, Kang C Han J, et al. N-doped carbon nanorods from biomass as a potential antidiabetic nanomedicine. ACS Biomater Sci Eng. 2022;8(5):2131–2141.
- Sharma N, Das GS, Yun K. Green synthesis of multipurpose carbon quantum dots from red cabbage and estimation of their antioxidant potential and bio-labeling activity. Appl Microbiol Biotechnol. 2020;104(16):7187–7200.
- Bellumori M, Innocenti M, Michelozzi M, et al. Coloured-fleshed potatoes after boiling: promising sources of known antioxidant compounds. J Food Compost Anal. 2017;59:1–7.
- Bajpai VK, Khan I, Shukla S, et al. N,P-doped carbon nanodots for food-matrix decontamination, anticancer targeting, and cellular bio-imaging applications. J Biomed Nanotechnol. 2020;16:283–303.
- Wei X, Li L, Liu J, et al. Green synthesis of fluorescent carbon dots from gynostemma for bioimaging and antioxidant in zebrafish. ACS Appl Mater Interfaces. 2019;11(10):9832–9840.
- Zhao S, Lan M, Zhu X, et al. Green synthesis of bifunctional fluorescent carbon dots from garlic for cellular imaging and free radical scavenging. ACS Appl Mater Interfaces. 2015;7(31):17054–17060.
- Mitra T, Sailakshmi G, Gnanamani A, et al. Studies on cross-linking of succinic acid with chitosan/collagen. Mater Res. 2013;16(4):755–765.
- Fujimoto A, Yamada Y, Koinuma M, et al. Origins of sp3C peaks in C1s X-ray photoelectron spectra of carbon materials. Anal Chem. 2016;88(12):6110–6114.
- Li S, Wang L, Chusuei CC, et al. Nontoxic carbon dots potently inhibit human insulin fibrillation. Chem. Mater. 2015;27(5):1764–1771.
- Kanthi Gudimella K, Gedda G, Kumar PS, et al. Novel synthesis of fluorescent carbon dots from bio-based Carica papaya leaves: optical and structural properties with antioxidant and anti-inflammatory activities. Environ Res. 2022;204(Pt A):111854.
- Garcia-Contreras M, Thakor AS. Human adipose tissue-derived mesenchymal stem cells and their extracellular vesicles modulate lipopolysaccharide activated human microglia. Cell Death Discov. 2021;7(1):1–13.
- Cheng Z, Bao Y, Li Z, et al. Lonicera caerulea (Haskap berries): a review of development traceability, functional value, product development status, future opportunities, and challenges. Crit Rev Food Sci Nutr. 2022;18:1–25.
- Wojdyło A, Nuncio Jáuregui PN, Carbonell-Barrachina Oszmiański J, et al. Variability of phytochemical properties and content of bioactive compounds in Lonicera caerulea L. var. kamtschatica berries. J Agric Food Chem. 2013;61(49):12072–12084.
- Zhang J, Wu J, Liu F, et al. Neuroprotective effects of anthocyanins and its major component cyanidin-3-O-glucoside (C3G) in the central nervous system: an outlined review. Eur J Pharmacol. 2019;858:172500.
- Shin JH, Park M, Lee HJ. Cyanidin-3-O-Glucoside regulates the M1/M2 polarization of microglia via PPARγ and Aβ42 phagocytosis through TREM2 in an Alzheimer’s disease model. Mol Neurobiol. 2022;59(8):5135–5148.
- Kim GH, Kim JE, Rhie SJ, et al. The role of oxidative stress in neurodegenerative diseases. Exp Neurobiol. 2015;24(4):325–340.
- Shi D, Yang J, Jiang Y, et al. The antioxidant activity and neuroprotective mechanism of isoliquiritigenin. Free Radic Biol Med. 2020;152:207–215.
- Li F, Li T, Sun C, et al. Selenium‐doped carbon quantum dots for free‐radical scavenging. Angew Chem Int. Ed. 2017;56(33):9910–9914.
- Bitner BR, Marcano DC, Berlin JM, et al. Antioxidant carbon particles improve cerebrovascular dysfunction following traumatic brain injury. ACS Nano. 2012;6(9):8007–8014.
- Heckman KL, Estevez AY, DeCoteau W, et al. Variable in vivo and in vitro biological effects of cerium oxide nanoparticle formulations. Front Pharmacol. 2020;10:1599.
- Pardhiya S, Priyadarshini E, Rajamani P. In vitro antioxidant activity of synthesized BSA conjugated manganese dioxide nanoparticles. SN Appl Sci. 2020;2(9):1–12.
- Kalyanaraman B, Darley-Usmar V, Davies KJ, et al. Measuring reactive oxygen and nitrogen species with fluorescent probes: challenges and limitations. Free Radic Biol Med. 2012;52(1):1–6.
- Wang Y, Kong W, Wang L, et al. Optimizing oxygen functional groups in graphene quantum dots for improved antioxidant mechanism. Phys Chem Chem Phys. 2019;21(3):1336–1343.
- Ji Z, Sheardy A, Zeng Z, et al. Tuning the functional groups on carbon nanodots and antioxidant studies. Molecules. 2019;24(1):152.
- Ighodaro O, Akinloye O. First line defence antioxidants-superoxide dismutase (SOD), catalase (CAT) and glutathione peroxidase (GPX): their fundamental role in the entire antioxidant defence grid. Alexandria J Med. 2018;54(4):287–293.
- Al-Sawaf O, Clarner T, Fragoulis A, et al. Nrf2 in health and disease: current and future clinical implications. Clin Sci. 2015;129(12):989–999.
- Sun X, Wang Y, Ji K, et al. NRF2 preserves genomic integrity by facilitating ATR activation and G2 cell cycle arrest. Nucleic Acids Res. 2020;48(16):9109–9123.
- Li X, Ye F, Li L, et al. The role of HO-1 in protection against lead-induced neurotoxicity. Neurotoxicology. 2016;52:1–11.
- Abraham NG, Kappas A. Pharmacological and clinical aspects of heme oxygenase. Pharmacol Rev. 2008;60(1):79–127.
- Maines MD. The heme oxygenase system: update 2005. Antioxid. Redox Signal. 2005;7(11-12):1761–1766.
- Maines MD. The heme oxygenase system: a regulator of second messenger gases. Annu Rev Pharmacol Toxicol. 1997;37(1):517–554.
- Lai T-H, Shieh J-M, Tsou C-J, et al. Gold nanoparticles induce heme oxygenase-1 expression through Nrf2 activation and Bach1 export in human vascular endothelial cells. Int J Nanomed. 2015;10:5925.
- Lu S, Guo S, Xu P, et al. Hydrothermal synthesis of nitrogen-doped carbon dots with real-time live-cell imaging and blood–brain barrier penetration capabilities. Int J Nanomed. 2016;11:6325.
- Lu Z, Liu S, Lopes-Virella MF, et al. LPS and palmitic acid Co-upregulate microglia activation and neuroinflammatory response. Compr Psychoneuroendocrinol. 2021;6:100048.