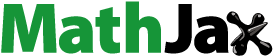
Abstract
Magnetosomes are iron oxide or iron sulphide nano-sized particles surrounded by a lipid bilayer synthesised by a group of bacteria known as magnetotactic bacteria (MTB). Magnetosomes have become a promising candidate for biomedical applications and could be potentially used as a drug-carrier. However, pharmacokinetics and immunogenicity of the magnetosomes have not been understood yet which preclude its clinical applications. Herein, we investigated the pharmacokinetics of magnetosomes including Absorption, Distribution, Metabolism, and Elimination (ADME) along with its immunogenicity in vitro and in vivo. The magnetosomes were conjugated with fluorescein isothiocyanate (Mag-FITC) and their conjugation was confirmed through fluorescence microscopy and its absorption in HeLa cell lines was evaluated using flow cytometry analysis. The results revealed a maximum cell uptake of 97% at 200 µg/mL concentration. Further, the biodistribution of Mag-FITC was investigated in vivo by a bioimaging system using BALB/c mice as a subject at different time intervals. The Mag-FITC neither induced death nor physical distress and the same was eliminated post 36 h of injection with meagre intensities left behind. The metabolism and elimination analysis were assessed to detect the iron overload which revealed that magnetosomes were entirely metabolised within 48-h interval. Furthermore, the histopathology and serum analysis reveal no histological damage with the absence of any abnormal biochemical parameters. The results support our study that magnetosomes were completely removed from the blood circulation within 48-h time interval. Moreover, the immunogenicity analysis has shown that magnetosomes do not induce any inflammation as indicated by reduced peaks of immune markers such as IL 1β, IL 2, IL 6, IL8, IFN γ, and TNF α estimated through Indirect ELISA. The normal behaviour of animals with the absence of acute or chronic toxicities in any organs declares that magnetosomes are safe to be injected. This shows that magnetosomes are benign for biological systems enrouting towards beneficial biomedical applications. Therefore, this study will advance the understanding and application of magnetosomes for clinical purposes.
Introduction
Advances in nanotechnology have completely transformed the twentieth century due to the progression in science and engineering leading to the synthesis and development of nanoparticles [Citation1]. Recent developments in nanoparticles revealed their dual capabilities such as diagnosis and treatment for a disease. There has been a steadily growing interest in the field of nanosystems to implement them in several biomedical applications. Also, the concept of nano pharmaceuticals has been in use by employing additional functionalities to the existing active compound. Being in a size range of 10 nm–100 nm makes them advantageous to circulate in the system and penetrate the targeted tissues through capillaries [Citation2].
With this as a criterion, research has been undertaken to put forth pharmacologically active biomolecules through biologically synthesised nanoparticles. They have been a potential candidate for the delivery of various biomolecules such as drugs, nutraceuticals, vaccines, DNA/RNA extraction, anti-cancer activity, anti-microbial activity and have been implemented in designing biosensors too [Citation3–5]. However, there was no anticipated success in nano-delivery systems due to specific organ damage, environmental impact, aggregation/disintegration of particles post-delivery, and the unforeseen reactions remain unpredictable [Citation6,Citation7]. It is important to emphasise these aspects as prolonged exposure of metallic nanoparticles changes the liver function that may trigger hepatic injury. This process collapses the entire system due to the enormous release of oxidative free radicals, disrupting the oxidation-deoxidation balance [Citation8,Citation9]. Also, the requirement of strong reducing agents for some nanoparticles failed the procedure, and the binding of active biomolecules to the active component was not always achieved [Citation10]. However, nanomedicine enables the accurate diagnosis, treatment, and ongoing monitoring of diseases as well as their early detection and prevention. Researchers are working towards more effective targeted drug delivery due to the rise in demand for highly effective medications. Even while many diseases can be treated, the adverse side effects have been growing at a rate equal to the therapeutic effect. Therefore, biologics are being targeted to be modified easily and accurately [Citation11].
Among various nanoparticles, iron-oxide (magnetic) nanoparticles have received greater attention due to their magnetic properties and guided drug delivery. Here, magnetic nanoparticles encapsulated in biological membranes can also be employed for comparable operations because nanotechnology has been proven to be promising in the medical industry and to replace with a greater efficacy over artificially synthesised nanoparticles. They gain special importance as their inherent magnetism eases targeted drug delivery [Citation12]. They initiate by magnetically binding to particular tissues or by having a strong connection between the ligand and the receptor facilitating the release of drugs to specific organs [Citation13]. With this as a basis, magnetic nanoparticles have been implemented in tumour imaging, contrast agents in Magnetic Resonance Imaging and are being implemented in conjugation with antibodies and chemotherapeutic drugs [Citation14]. Still, their toxicity, agglomeration in the biological system, bio-incompatibility, and the need for crosslinkers to functionalise biomolecules make them unfit for biomedical applications [Citation15].
Magnetosomes are nanoparticles that synthesise either iron oxide (Fe3O4) or iron sulphide (Fe3S4) under strict genetic control by Magnetotactic Bacteria (MTB) [Citation16]. The size of the magnetosomes (iron oxide or iron sulphide) ranges between 35 nm and 120 nm and is naturally surrounded by the organic membrane [Citation17]. The magnetosome membrane that envelopes the crystal contains phospholipids and other specific proteins; This structure provides a better platform for immobilising the biomolecules that can be eventually used as carriers for drug, antibodies, etc., [Citation18, Citation19]. The membrane makes them highly bio-compatible in nature as they are rich in amino acids and aid in the binding process. Also, amino groups of the magnetosome proteins can easily bind with any active ingredient and can be initiated for drug delivery [Citation19]. The role of magnetosomes have already been established in oral insulin delivery, magnetic hyperthermia, detection of food pathogens using biosensors, and wound healing activity [Citation20–22].
Despite several advantages of magnetosomes that have been mentioned earlier, the pharmacokinetics and immunogenicity of magnetosomes have not been investigated in detail till date and therefore cannot be recommended for further clinical applications. These parameters should be considered as they can also pose serious threats by depositing in the body and induce embolism [Citation19]. The proteins and lipids in the magnetosome membrane should be assessed for immunotoxicity to acquire the approval of magnetosomes as a carrier molecule. This research article reveals the pharmacokinetics and immunogenicity of magnetosomes directing its usefulness for biomedical applications, especially for drug/biomolecule delivery. Therefore, this study will advance the understanding and applications of magnetosomes for clinical purpose.
Materials and methods
Materials
Magnetospirillum gryphiwaldense (MSR-1) was purchased from Deutsche Sammlung von Mikroorganismen und Zellkulturen (DSMZ) Germany. HeLa cells were purchased from the American Type Culture Collection (ATCC) centre and cultured in Dulbecco’s Modified Eagles Medium (Himedia, India). They were supplemented in 10% FBS (Sigma-Alhrich, India) with 50 U/mL penicillin-streptomycin (Himedia, India). Fluorescein Isothiocyanate (FITC) was purchased from Sigma-Aldrich, India. The other chemicals used in the study were of analytical grade. Also, to carry out the in vivo experiments, Wistar albino rats and BALB/c mice were used to assess the pharmacokinetics of magnetosomes and approval was obtained from the Institutional Animal Ethical Committee (VIT/IAEC/20/DEC2021/03); (VIT/IAEC/23/May/02)
Extraction of magnetosomes from magnetotactic bacteria
The strain Magnetospirillum gryphiwaldense (MSR-1) was cultured in magnetospirillum growth medium (MSGM) by Hungate anaerobic technique [Citation20]. After 48 h of bacterial growth, the bacterial pellet was obtained by centrifugation (Refrigerated centrifuge L1-HRC-16K, Lark Innovative Fine Teknowledge, India) followed by sonication process (Sonics & Materials, Inc, India) to extract the magnetosomes from magnetotactic bacteria [Citation21]. The obtained magnetosomes were freeze-dried using a lyophilizer (Lark, Penguin Classic Plus, India). The primary freezing step was carried out for 2 h at − 45 °C, followed by primary and secondary drying for 12 h and 6 h at −30 °C and 20 °C, respectively. The lyophilised magnetosomes were stored in air-tight vials at room temperature for further applications.
Characterisation of magnetosomes
To analyse the morphology and size of magnetosomes, Scanning Electron Microscopy (SEM) (Carl Zeiss, SUPRA 55VP) was operated with a magnification of 50 kx obtained at 20 keV along with Energy-dispersive X-ray spectroscopy (EDS) (Zeiss EV018, Germany) to measure the energy distribution and intensity generated by the electron beam striking the surface of magnetosomes. To assess the 3-dimensional structure of magnetosomes, Atomic Force Microscopy (AFM) (Nanosurf Easy Scan 2, SPM Electronics, Liestal, Switzerland) was carried out. AFM was performed using cantilevers, which included silicon nitride probes from Bruker (DNP-10; tip radius of 20–60 nm) and tips from Olympus AC160TS and AC240TS (tip radii <10 nm) [Citation21]. At a line frequency of less than one hertz (<1 Hz), images were captured. Also, Zeta potential analysis (Horiba Scientific SZ-100) was performed to assess the potential stability of magnetosomes operated at 200.0 V. Fourier Transform Infra-red Spectroscopy (FT-IR) (Schimadzu, Japan) was evaluated to identify the functional group of magnetosomes wherein the lyophilised magnetosomes were mixed with KBr in 1:100 ratio and the spectrum was chosen between 4000 and 500 cm−1 [Citation23]. X-ray Diffraction analysis (Anton Paar, Switzerland) was imaged to assess the variation in diffraction moment and lattices of magnetosome structure. The dried magnetosomes were analysed under Cu Ka radiation, 25 mA, at 35 kV [Citation24].
Conjugation of magnetosomes with fluorescein isothiocyanate
Magnetosomes (1 mg/mL) were conjugated with fluorescein isothiocyanate (FITC) molecule (2 mg/mL) by incubating them at 16 °C overnight in 0.5 M HEPES + 0.1 M EDTA. The component was further purified by placing them near the magnet for removal of free FITC and was washed several times with PBS solution. The conjugate was analysed through fluorescence microscopy (Weswox, FM, 3000) at 100× magnification measured at 1 mm scale bar. The results were validated through FT-IR analysis.
Magnetosome absorption- mechanism of uptake of magnetosome by cells
The in vitro absorption study was carried out on cervical cancer cell lines (HeLa). The confluent cells were passaged and maintained in Dulbecco’s Modified Eagles Medium (DMEM) at 37 °C. The medium was seeded with 10% foetal bovine serum (FBS) and 50 U/mL penicillin-streptomycin maintained in a humidified atmosphere [Citation25]. HeLa cells were grown in six well plates to become confluent. After which varying concentrations of Magnetosome- FITC (Mag-FITC) was added into each well (200 µL, 150 µL, 100 µL, 50 µL, 10 µL). After the incubation period, the media was removed from the six-well plate and rinsed with PBS solution. The plates were screened through fluorescence microscopy at 100 X magnification to analyse the absorption process.
To confirm the cell uptake, the analysis was further proceeded with the addition of 250 µL of 0.25% of trypsin to each well and incubated for 2–3 min. To stop the reaction, 1 mL of FACS buffer was added, and the component was centrifuged at 1500 rpm for 5 min at room temperature. The acquired pellet was loosened, and 1 mL of FACS buffer was added to the loosened pellet. The percentage of cell uptake was assessed using flow cytometry (Cytoflex, Beckman coulter, US) analysis.
Assessment of magnetosome-FITC biodistribution process
Forty BALB/c mice, weighing 40–60 g, were obtained from the animal house of VIT (ethical clearance number – VIT/IAEC/23/May 23/02). The animals were maintained at 25–30° C with 12 h light and dark cycle maintained at ad libitum conditions. To assess the biodistribution of the Mag-FITC, the conjugant was injected intraperitoneally in the subject and assessed through different time frames (5 min, 30 min, 60 min, 90 min, 12 h, 24 h, 36 h, 48 h, 60 h and 72 h). Here, the control subjects were injected with sodium chloride to detect autofluorescence. The animal was anaesthetised by inducing 2–4% of isoflurane, and the anaesthetised subject was placed in the whole-body fluorescence imaging system (IVIS spectrum, USA). The image acquired was screened for the distribution of Mag-FITC in the body of the subject.
In another study, 12 female and 12 male Wistar albino rats weighing around 180–220 g were used for the pharmacokinetics analysis (ethical clearance number: VIT/IAEC/20/DEC2021/03). 5 mg/mL of Mag-FITC was injected at the tail vein and blood was drawn at 0th minute, 10 min, 30 min, 1 h, 2 h, 4 h, 8 h, 12 h, 24 h, 36 h, 48 h. Also, the injected subjects were sacrificed at 4 h, 8 h, 12 h, 24, 36, and 48 h to assess the biodistribution process. The rats were placed in metabolic cages for the collection of urine samples, and faecal samples were collected from the bed of the subject at regular intervals.
The organs from the sacrificed animals were collected and preserved with 10% buffered formalin for histological analysis. Organ tissues were sectioned, embedded in paraffin blocks, and set on glass slides. Haematoxylin and eosin were used to stain the slides, which were then examined under a microscope.
To estimate the biodistribution of Mag-FITC quantitatively, the organs were isolated from the euthanized animals at definitive time intervals. Organs such as the heart, intestine, kidney, lungs, liver, and spleen were isolated, washed several times with distilled water and homogenised with PBS solution using a homogeniser (Remi RQT- 127 A/D Homogeniser, India). 100 µL of the homogenised sample were transferred into 96 well microtiter plates and the fluorescence intensity was measured at 590 nm using an ELISA plate reader (LIMR96, Lark innovative fine teknowledge, India). To nullify the effect of autofluorescence 15 mM of sodium chloride was injected to the control group [Citation26]. The percentage of the measured dose was defined as a criterion quantifying Mag-FITC biodistribution, and the total fluorescence measured was calculated according to the given formula:
Metabolism – assessment of iron-overload
To estimate the presence of iron at different time intervals, the serum samples were analysed through a standard iron detection colorimetric assay (Elabscience, USA). Briefly, 1 mL of the blood samples were collected at different time intervals (0th minute, 10 min, 30 min, 1 h, 2 h, 4 h, 8 h, 12 h, 24 h, 36 h, 48 h). The samples were centrifuged and the acquired serum were mixed with the standard reagents and incubated for 40 min. The incubated samples were measured spectrophotometrically at 593 nm using an ELISA plate reader (LIMR96, Lark innovative fine teknowledge, India).
Elimination- fate of magnetosomes
To estimate the presence of magnetosomes, faecal and urine samples were collected at definite intervals. 2 mL of acid digested faecal and urine samples were used for the analysis. The samples were taken at 0th hour, 10 min, 30 min, 1 h, 2 h, 4 h, 8 h, 12 h, 24 h, 36 h and 48 h and were subjected to acid digestion. The fate of the magnetosomes was analysed using flame atomic absorption spectrophotometer (FAAS) (Agilent 240 FS AA, India).
Immunogenicity of magnetosomes
To determine the immunogenicity of magnetosomes, indirect ELISA was performed with IL-1 β, IL-2, IL-6, IL-8, IFN-γ and TNF α. Briefly, 100 µL of the serum sample prepared in coating buffer was loaded into 96 well titre plate and incubated at 4° C overnight. After incubation, the serum samples were removed and washed with PBST solution 3 times. Further, 100 µl of 1:1000 dilution of primary antibody diluted in PBS was added to the washed titre plate and incubated at 37° C for 1 h. Later, the primary antibody was discarded and washed with PBST solution three times. After this, 100 µL of 1:1000 dilution of secondary antibody labelled in sample diluent was added to the washed wells and incubated in room temperature for 1 h. The incubated secondary antibody was discarded and washed three times with PBST solution, and 100 µL of ABTS substrate solution was incubated in washed wells for 30 min and measured spectrophotometrically at 405 nm using an ELISA plate reader (LIMR96, Lark innovative fine teknowledge, India).
Results
Extraction and characterisation of magnetosomes
The extracted magnetosomes procured from Magnetospirillum gryphiwaldense (MSR-1) weighed approximately 5–7 mg/L. The Scanning Electron Microscopy results reveal the cubo-octahedral shape of the magnetosomes with sizes ranging up to 120 nm as represented in and EDS analysis explains the presence of iron in magnetosomes (). Whereas the FT-IR analysis measured the spectrum between 400 cm−1 and 4000 cm−1 (). The Fe-O bond represents the presence of iron oxide; the other stretching vibrations are due to the presence of proteins, lipopolysaccharides, and phospholipids present on the magnetosome membrane. represents the Atomic Force Microscopy analysis revealing the uniform particle structure and arrangement of a chain-like organisation. In XRD () analysis, the sequence of peaks was recorded at 27.05, 30.30, 35.71, and 43.31, correlating the standard pattern of Fe3O4. The magnetosome’s Zeta potential given in measures approximately −23.8 mV suggesting their surface charge and distribution of small sizes. The negative charge is due to the presence of a lipid membrane surrounding the magnetosomes.
Figure 1. Characterisation of magnetosomes representing (a) SEM analysis revealing the nanoscale size of magnetosomes with chain-like structures ranging around 200 nm; (b) EDS analysis confirms the presence of iron in magnetosomes; (c) FT-IR spectrum measured between 500 cm−1 to 4000 cm−1 confirms the presence of the Fe-O bond. This confirms the presence of iron oxide from the extracted magnetosomes; (d) AFM represents the uniform particle structure and is organised in a chain arrangement. The cuboctahedron shape is evident in this image with size ranging from 100 to 120 nm. The size of the magnetosomes was corrected and measured using image J software; (e) XRD analysis of magnetosomes; (f) Zeta potential analysis of magnetosomes.
Conjugation of magnetosomes with fluorescein isothiocyanate and their mechanism of uptake by cells
represents the conjugation of magnetosomes with fluorescein isothiocyanate, observed through fluorescence microscopy at 100×. To confirm the chemical bonding between magnetosomes and fluorescein isothiocyanate, FT-IR spectroscopy was performed. High surface-to-volume atom ratio, high nanoparticle surface energy, and number of surface bonds are a few significant determining factors for the adsorption of aqueous solution ions on the surface of magnetic nanoparticles. depicts free Fe and O atoms near the surface of a neutral solution containing dispersed magnetosomes that adsorb H + and OH+, explaining the density of the functional group (−OH).
Figure 2. (a) Represents control- a microscopic image of magnetosomes, and (b) represents the conjugation of Mag-FITC analysed through fluorescence microscopy (c) schematic illustration of magnetosomes conjugation with FITC molecule.
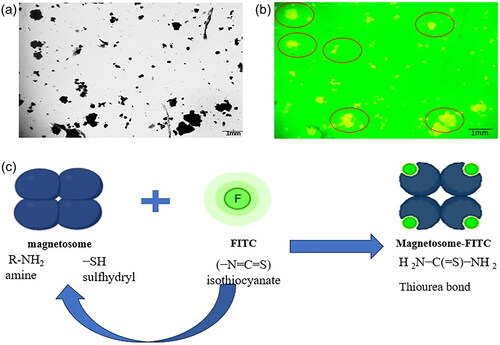
Figure 3. FT-IR spectrum representing the chemical bonding between magnetosomes and fluorescein isothiocyanate.
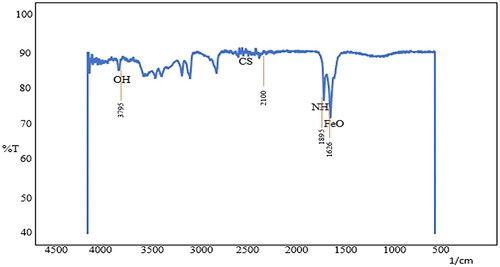
To assess the in vitro analysis of magnetosome absorption through cell lines, Mag-FITC was treated at various concentrations (200 µg/mL, 150 µg/mL, 100 µg/mL, 50 µg/mL, 10 µg/mL) with HeLa cells and analysed through fluorescence microscopy and the cell uptake was observed from 100 to 200 µg/mL concentration of Mag-FITC as shown in . To confirm the percentage of cell uptake, flow cytometry analysis was performed, which revealed the cell uptake with increasing concentrations ranging from 1.23%, 16.25%, 93.28%, 96.29%, 97.41% in 10 µg/mL, 50 µg/mL, 100 µg/mL, 150 µg/mL, 200 µg/mL respectively. represents the percentage of cell uptake in HeLa cell lines.
Assessment of magnetosome-FITC biodistribution process
The biodistribution of magnetosomes- FITC through bioimaging system was assessed in the subject at different time intervals. Initially, to nullify the effect of autofluorescence, the control rats were injected with a higher concentration of sodium chloride (20 mg/mL). At 5 min time interval, a high intensity of fluorescence was detected which decreased with increase in time interval. After 2 h, meagre intensities of fluorescence were imaged in the system. With this as a parameter, Mag- FITC were injected into the tested subjects. At 5-min time interval, Mag-FITC was found to accumulate in the intraperitoneal region and spread at the site of injection. After 30 min, Mag-FITC was found to spread in the intestine and amassed in the regions of intraperitoneal fluid. Whereas, after 60 min Mag-FITC was identified at the lungs, intestine and liver. At 90 min time interval Mag-FITC was accumulated in the lungs, intestine, kidney and liver. The biodistribution of magnetosomes was seen at the maximum of 12 h interval, and the distribution fades out with an increase in time with meagre intensities found after 36 h. At the end of 72 h, the total Mag-FITC has been totally washed out from the system. Therefore, it can be used as an effective carrier for diverse biomedical applications. represents the biodistribution of Mag-FITC in BALB/c mice.This experiment could form a basis to clarify that magnetosome-FITC conjugant is not harmful to the subject and is eliminated within 36-h time interval, with few traces left behind.
Figure 6. Biodistribution of Mag-FITC assessed at different time intervals (Scale bar: 5.0 cm) (a) control (sodium chloride) (5 min) (b) control (sodium chloride) (2 h) (c) 5 min (d) 30 min (e) 60 min (f) 90 min (g) 12 h (h) 24 h (i) 36 h (j) 48 h (k) 60 h (l) 72 h.
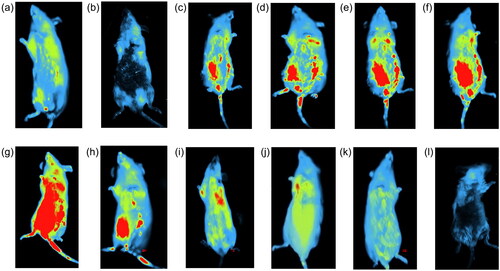
Similarly, when tested with the biodistribution of magnetosomes in Wistar albino rats, any differences in water intake and food consumption were not observed in the treated and control groups. The rats were sacrificed at 4th hour, 8th hour, 12th hour, 24 h, 36 h, 48 h. The serum samples, urine, and faecal samples were collected at definite intervals, and the organs collected were submitted for histopathology analysis. represents the histopathology analysis of the samples taken at different time intervals. The microscopic parenchymal architecture evidenced normal structures without any sign of inflammation or fibrosis. No significant differences in the tissue samples were observed, and no histological abnormalities were detected. represents the serum analysis taken at different time intervals with parameters falling within the normal range.
Figure 7. (a) Effect of magnetosomes in Wistar albino rats. Histopathology images (Scale bar: 800 um) of organs stained by H and E staining of magnetosomes injected at different time intervals. (b) Blood factors, renal factors and hepatic factors of animals treated with magnetosomes taken at different time intervals. BG: blood glucose (mg/dL); U: urea (mgs/dL); C: creatinine (gm/dL); UC: uric acid (mgs/dL); TC: total cholesterol (mg/dL); TG: triglycerides (mg/dL); BR: bilirubin (mgs/dL); ALAT: Alanine Aminotransferase (U/L); ASAT: Aspartate Aminotransferase (U/L); TP: total protein (gms/dL); SA: serum albumin (gms/dL). (c) Biodistribution of Mag-FITC assessed at different time intervals in heart, intestine, kidney, liver, lungs and spleen.
The quantitative estimation of Mag-FITC biodistribution reveals that the highest fluorescence intensity was recorded at 12-h time interval, with an initial fast distribution phase followed by a slow distribution phase and a rapid reduction of biodistribution recorded after 12 h. Stable signals were recorded till 12 h with fewer detectable signals received after the maximum biodistribution of Mag- FITC in the subject. Among several organs detected, the fluorescence intensity was maximum recorded in the liver with 82.4% till 12-h time interval with gradual reduction of the intensities correlating with the metabolism process. The fluorescence intensities observed in the kidneys rapidly decreases after 12 h of Mag-FITC administration with meagre intensities observed till 48 h. The overall pharmacokinetic profile corresponds with the biodistribution process observed through the in vivo bioimaging system with a rapid reduction of Mag-FITC with an increase in time of exposure. explains the percentage of fluorescence detected in the organs during the assessment of the biodistribution process.
Metabolism of magnetosome-FITC
The estimation of iron overload reveals the presence of iron in the serum samples with respect to time. Samples restrained from the control group, 0th minute, 10th minute, 30th minute, 1 h, 2 h, 4 h, 8 h, 12 h, 24 h, 36 h, 48 h estimated the presence of iron with the maximum iron concentration at the 2th hour with 4 µg/mL of iron concentration. clearly explains the gradual increase in iron concentration with respect to time and gradually decreases with an increase in time. This expresses that the injected magnetosomes are being eliminated from the subject and the present iron can also be utilised as a cofactor by the body as it directly undergoes redox cycling [Citation27].
Elimination of magnetosome-FITC
The acid-digested faecal and urine samples collected at different time intervals (0–4 h, 4–8 h, 8–12 h, 12–24 h, 24–48 h) were assessed using flame atomic absorption spectrometry. represents a graph plotted with the percentage of iron detected against time, wherein maximum iron is eliminated from the body through faeces and urine within 48 h.
Immunogenicity of magnetosomes
The immunogenicity of magnetosomes was tested using serum samples taken at different time intervals and tested primarily for pro-inflammatory cytokines such as IL-1ß, IL-2, IL-6, IL-8, IFN-γ, TNF- α using the Indirect ELISA method. All the pro-inflammatory markers expressed immunogenic reaction with less than 1%. These results express that these nanoscale structures cannot result in embolism in the later stages, as it is known that magnetosome-associated proteins are of non-human origin [Citation28]. represents the immunogenicity of magnetosomes tested at different time intervals.
Discussion
Analysing pharmacokinetic profiles and biodistribution is an essential stage in the development of nanocarriers before evaluating their biosafety and efficacy in drug delivery. In this regard, magnetosomes have gained a lot of attention recently, particularly in the field of drug delivery. This study focuses on the assessment of pharmacokinetics and immunogenicity of magnetosomes to pave way for a promising role towards drug delivery and other biomedical applications. Initially, magnetosomes were characterised through microscopic (SEM/EDS) and spectroscopic observations (XRD, FT-IR, AFM, Zeta potential) that confirm the uniform size and cubo-octahedral shape of magnetosomes. The analysis revealed the presence of a magnetosome membrane and a high-purity crystal ranging up to 120 nm and the presence of a Fe-O bond. The previously reported strains of MSR-1 belonging to the genus Magnetospirullum possess similar morphology and crystal structure [Citation22]. Also, the reported strains produce magnetosomes of sizes ranging from 30-120 nm [Citation29] The electron-dense region representing the inorganic part is found to be enclosed within the magnetosome membrane as represented in . Also, the EDS () analysis represents the presence of iron, carbon and oxygen highlighting the inorganic region of the magnetosomes. representing the FT-IR analysis has shown the presence of CH2, FeO, C-C, CH, CO, and CO-NH2 at 1250 cm−1, 1670 cm−1, 3529 cm−1, 3970 cm−1, 3268 cm−1, 1831 cm−1 respectively corresponding to the presence of proteins, lipopolysaccharides, phospholipids present on the magnetosome membrane. Specifically, the stretching vibrations of carboxylic, amine, and amide groups represent the magnetosome-associated proteins, according to Taher et al. [Citation30]. The AFM analysis represented in reveals the chain arrangement of magnetosomes with regular chains corresponding to the pattern mentioned by Revathy et al. [Citation21]. 3–4 magnetosomes are formed in a short chain-like structure that resulted due to the sonication, during the extraction of magnetosomes from magnetotactic bacteria. The negative topographic image represents the skewness of the surface measured in the scanned sample. The X-ray diffraction pattern mentioned in records the Fe-O vibration imparting the ferromagnetic property of magnetosomes. The Zeta potential analysis () exhibited an overall negative charge of −28.4 mV, inferring the polarisable amino group present in the magnetosome membrane. The acquired results resemble the report given by Raguraman et al. [Citation20].
With the acquired results, we proceeded with the conjugation of FITC with magnetosomes. describes the conjugation of M-F observed through fluorescence microscopy. FITC, a derivative of fluorescein with an isothiocyanate reactive group, is reactive towards amine and sulfhydryl groups, and the amino group of the magnetosomes can easily bind with any active ingredient and therefore, has aided in the binding process efficiently. To confirm with the binding process, FT-IR analysis was performed () which derives further information about the vibrational groups of − OH and − CS present in the FITC molecule, −NH and FeO of the magnetosomes representing the reaction between isothiocyanate and the amine group of the magnetosome [Citation31].
To obtain exhaustive information regarding the pharmacokinetics of magnetosomes, ADME studies were performed. The absorption studies through HeLa cell lines revealed the internalisation of Mag-FITC with HeLa cells observed through fluorescence microscopy () and a promising cell uptake of 97% with 200 µg/mL concentration as mentioned in . The data suggests that endocytosis-based magnetosome internalisation with magnetosomes is stored in vesicle-like structures [Citation32], and the results are in accordance with previous reports [Citation33]. Also, cell uptake studies with magnetosomes performed by Taher et al. [Citation30] reveal the efficient cell uptake with MDA- MB-231 epithelial breast cancer cell lines. Our analysis with HeLa cell lines provides the capacity to deliver therapeutic substances directly into cells and to use magnetism to steer magnetosomes within the body. With this advantage, magnetosomes can be combined with therapeutics which can be supplied directly to the desired site [Citation34].
The biodistribution studies of magnetosome- FITC in BALB/c mice () revealed the maximum biodistribution at 12-h interval, and the distribution fades out with an increase in time with meagre intensities found after 36 h. At the end of 72 h, total Mag-FITC has been totally washed out from the system. The results have been compared with the control by injecting sodium chloride with autofluorescence diminishing totally within 2 h interval. These results are correlating with the quantification of magnetosome biodistribution as mentioned in . The statistical analysis derived through multiple t-test shows a significant difference in the fluorescence intensities of biodistribution in heart, liver, intestine, kidney, lungs and spleen at different time points as the significance of p < 0.0001 fitted. Therefore, it can be used as an effective carrier for diverse biomedical applications. Also, when assessed with the biodistribution using Wistar albino rats (), the histopathology analysis revealed that there was no histological abnormality. The sections from the heart showed normal cardiomyocytes with intact myonuclei and intermysial connective tissue spaces. The sections from the intestine reveal normal crypt epithelium and glands with normal lamina propria and normal-appearing muscular layers. Whereas the glomeruli sections from the kidney showed normal capillary structures, with typically appearing tubules and interstitium showing normal blood vessels. Sections from the liver showed normal hepatocytes, with intact sinusoids, normal portal triad, and central vein. The lung tissues expressed normal bronchial epithelium and focal normal lymphoid aggregates in the interstitium with normal appearing alveoli. The sections from the spleen showed normal white pulp with central arteriole and normal appearing red pulp with sinuses. The overall analysis revealed no significant pathologiesThe biodistribution studies performed in dendrimer-coated iron oxide nanoparticles revealed the bioaccumulation in lungs and kidney tissues claiming the opsonisation process by the macrophages in the reticuloendothelial system and the intracellular catabolism process by the kidney [Citation35]. This comparison reveals that magnetosomes when present in the system did not cause any bioaccumulation or histological damage and have been efficiently removed from the system with fewer trace elements left. However, further studies could be elaborated by studying the mechanism of the reticuloendothelial system with regard to magnetosome interaction within the system. Also, the biochemistry of blood factors, renal factors, and hepatic factors exhibited no significant difference in the parameters revealing the safe exposure of magnetosomes in the system (). The multiple t-test was used to determine the statistical significance of each parameter taken at different time intervals. The blood factors, renal factors and the hepatic factors showed a statistical significance of p < 0.05, with all the values comprising within the threshold range and so are not considered to be a risk factor. Therefore, the biodistribution of magnetosomes assessed using rat and mouse models shows good agreement when compared with the outcome of other studies.
When comparing with the metabolism and elimination studies of magnetosomes ( and ), the preferred excretion route of magnetosomes is through kidneys, rendering negligible intracellular catabolism with a minimal probability of reactive oxygen species [Citation36]. Statistical analysis was performed from the data derived from urine and faecal samples at different time intervals using multiple t-test. A highly significant value was reached with a p-value of 0.01. The threshold of statistical significance was set to p < 0.05.
This shows that the metabolism route can sequester nanoparticles chemically and physically which influences the elimination process [Citation37]. The immunogenicity analysis tested for specific pro-inflammatory cytokines exposes no/very mild immunogenic reaction of 1% (), declaring it to be safe for them to be present inside the system. The data derived from the tested cytokines were found to be comprising within the non-immunogenic threshold. The multiple t-test was analysed and revealed a slight but statistical significance with p-value < 0.05.
On the other hand, magnetosomes have been reported with the production of endotoxins that is found surrounded by the part of organic mineral core. On exceeding the desired threshold, might result in inducing toxicity in the system. Therefore, further research is required in quantifying the protein secreted cells of magnetosomes in order to overcome the detrimental effects [Citation38]. Since the magnetosome membrane proteins are of a non-human origin, it is a very crucial parameter to study their safety profile. Studies have suggested that engineered nanoparticles in conjugation with potent adjuvants did not revert any immunogenic reactions, which is in accordance with our study [Citation39,Citation40]. Therefore, the overall pharmacokinetics and immunogenicity process reveals a good profile that can be further escalated for biomedical applications. This shows that the bioavailability, distribution, metabolism, and permeability of the magnetosomes are better in comparison with other magnetic nanoparticles and micro-sized drugs. Further studies can transform the usage of magnetosomes towards personalised medicine.
Conclusion
In conclusion, our study demonstrates the safety of magnetosomes and emphasises its usage for effective biomedical applications. This research indicates that magnetosomes can be used as an efficient carrier. The ADME analysis of magnetosomes assessed in vitro and in vivo reveals a safe and excellent pharmacokinetic profile with absence/minimal immunogenicity. Therefore, by understanding the safety profile of magnetosomes, future work can be directed towards the disease-targeting ability and pave way for an extensive research in scientific community and validate for human usage.
Author contributions
Dr. K. Suthindhiran conceptualised the work on pharmacokinetics and immunogenicity of magnetosomes and performed a formal analysis of every step. The project administration and concerns regarding the resources were provided by Dr. K. Suthindhiran. Ms. M. Haripriyaa proceeded with the acquisition of data for all the analysis and validated the results. Ms. M. Haripriyaa was involved in manuscript preparation followed by drafting the work. After a series of critical evaluations, the raw data was converted into a manuscript, which was overseen and validated by Dr. K. Suthindhiran.
Acknowledgment
The authors are thankful to Vellore Institute of Technology, Vellore for providing the necessary facilities to carry out this research.
Disclosure statement
No potential conflict of interest was reported by the author(s).
Data availability statement
The authors confirm that the data supporting the findings of this study are available within the article.
Additional information
Funding
References
- Nikalje AP. Nanotechnology and its applications in medicine. Med Chem. 2015;5(2):081–089. doi:10.4172/2161-0444.1000247].
- Mabrouk M, Das DB, Salem ZA, et al. Nanomaterials for biomedical applications: production, characterisations, recent trends and difficulties. Molecules. 2021;26(4):1077. [PubMed: 33670668]. doi:10.3390/molecules26041077.
- El-Zayat MM, Eraqi MM, Alrefai H, et al. The antimicrobial, antioxidant, and anticancer activity of greenly synthesized selenium and zinc composite nanoparticles using Ephedra aphylla extract. Biomolecules. 2021;11(3):470. [PubMed: 33809976]. doi:10.3390/biom11030470.
- Magro M, Venerando A, Macone A, et al. Nanotechnology-based strategies to develop new anticancer therapies. Biomolecules. 2020;10(5):735. [PubMed: 32397196]. doi:10.3390/biom10050735.
- Nikolova MP, Chavali MS. Metal oxide nanoparticles as biomedical materials. Biomimetics. 2020;5(2):27. [PubMed: 32521669]. doi:10.3390/biomimetics5020027.
- Chenthamara D, Subramaniam S, Ramakrishnan SG, et al. Therapeutic efficacy of nanoparticles and routes of administration. Biomater Res. 2019;23(1):20. [PubMed: 31832232]. doi:10.1186/s40824-019-0166-x.
- Harish V, Tewari D, Gaur M, et al. Review on nanoparticles and nanostructured materials: bioimaging, biosensing, drug delivery, tissue engineering, antimicrobial, and agro-food applications. Nanomaterials (Basel). 2022;12(3):457. [PubMed: 35159802]. doi:10.3390/nano12030457.
- Mitchell MJ, Billingsley MM, Haley RM, et al. Engineering precision nanoparticles for drug delivery. Nat Rev Drug Discov. 2021;20(2):101–124. [PubMed: 33277608]. doi:10.1038/s41573-020-0090-8.
- Yao Y, Zang Y, Qu J, et al. The toxicity of metallic nanoparticles on liver: the subcellular damages, mechanisms, and outcomes. Int J Nanomedicine. 2019;14:8787–8804. [PubMed: 31806972]. doi:10.2147/IJN.S212907.
- Amina SJ, Guo B. A review on the synthesis and functionalization of gold nanoparticles as a drug delivery vehicle. Int J Nanomed. 2020;15:9823–9857. [PubMed: 33324054]. doi:10.2147/IJN.S279094.
- Tamargo J, Le Heuzey JY, Mabo P. Narrow therapeutic index drugs: a clinical pharmacological consideration to flecainide. Eur J Clin Pharmacol. 2015;71(5):549–567. [PubMed: 25870032]. doi:10.1007/s00228-015-1832-0.
- Komeili A. Molecular mechanisms of compartmentalization and biomineralization in magnetotactic bacteria. FEMS Microbiol Rev. 2012;36(1):232–255. doi:10.1111/j.1574-6976.2011.00315.x.
- Kianfar E. Magnetic nanoparticles in targeted drug delivery: a review. J Supercond Nov Magn. 2021;34(7):1709–1735. doi:10.1007/s10948-021-05932-9].
- Kalubowilage M, Janik K, Bossmann SH. Magnetic nanomaterials for magnetically-aided drug delivery and hyperthermia. Appl Sci. 2019;9(14):2927. doi:10.3390/app9142927].
- Materón EM, Miyazaki CM, Carr O, et al. Magnetic nanoparticles in biomedical applications: a review. Appl Surf Sci Adv. 2021;6:100163. doi:10.1016/j.apsadv.2021.100163].
- Schüler D, Frankel RB. Bacterial magnetosomes: microbiology, biomineralization and biotechnological applications. Appl Microbiol Biotechnol. 1999;52(4):464–473. doi:10.1007/s002530051547.
- Arakaki A, Nakazawa H, Nemoto M, et al. Formation of magnetite by bacteria and its application. J R Soc Interface. 2008;5(26):977–999. [PubMed: 18559314]. doi:10.1098/rsif.2008.0170.
- Farjadian F, Moghoofei M, Mirkiani S, et al. Bacterial components as naturally inspired nano-carriers for drug/gene delivery and immunization: set the bugs to work? Biotechnol Adv. 2018;36(4):968–985. [PubMed: 29499341]. doi:10.1016/j.biotechadv.2018.02.016.
- Sun J, Li Y, Liang XJ, et al. Bacterial magnetosome: a novel biogenetic magnetic targeted drug carrier with potential multifunctions. J Nanomater. 2011;2011(2011):469031–469043. [PubMed: 22448162]. doi:10.1155/2011/469031.
- Raguraman V, Jayasri MA, Suthindhiran K. Magnetosome mediated oral insulin delivery and its possible use in diabetes management. J Mater Sci Mater Med. 2020;31(8):75. [PubMed: 32761252]. doi:10.1007/s10856-020-06417-2.
- Revathy T, Jacob JJ, Jayasri MA, et al. Microbial biofilm prevention on wound dressing by nanobiocoating using magnetosomes-coupled lemon grass extract. IET Nanobiotechnol. 2017;11(6):738–745. doi:10.1049/iet-nbt.2016.0236].
- Sannigrahi S, Kumar A, Mathiyarasu S, et al. Detection of Escherichia coli in food samples by magnetosome-based biosensor. Biotechnol Bioproc E. 2023;28(1):152–161. doi:10.1007/s12257-022-0235-1].
- Raguraman V, Suthindhiran K. Comparative ecotoxicity assessment of magnetosomes and magnetite nanoparticles. Int J Environ Health Res. 2020a;30(1):13–25. [PubMed: 30714827]. doi:10.1080/09603123.2019.1570489.
- Sannigrahi S, Suthindhiran K. Metal recovery from printed circuit boards by magnetotactic bacteria. Hydrometallurgy. 2019;187:113–124. doi:10.1016/j.hydromet.2019.05.007.
- Raguraman V, Suthindhiran K. Comparative studies on functionalization of bacterial magnetic nanoparticles for drug delivery. J Clust Sci. 2020b;31(6):1275–1284. doi:10.1007/s10876-019-01737-y].
- Karabasz A, Szczepanowicz K, Cierniak A, et al. In vivo studies on pharmacokinetics, toxicity and immunogenicity of polyelectrolyte nanocapsules functionalized with two different polymers: poly-L-glutamic acid or PEG. Int J Nanomed. 2019;14:9587–9602. [PubMed: 31824153]. doi:10.2147/IJN.S230865.
- Abbaspour N, Hurrell R, Kelishadi R. Review on iron and its importance for human health. J Res Med Sci. 2014;19:164–174. [PubMed: 24778671].
- Sun JB, Tang T, Duan J, et al. Biocompatibility of bacterial magnetosomes: acute toxicity, immunotoxicity and cytotoxicity. Nanotoxicology. 2010;4(3):271–283. [PubMed: 20795909]. doi:10.3109/17435391003690531.
- Jacob JJ, Suthindhiran K. Magnetotactic bacteria and magnetosomes - Scope and challenges. Mater Sci Eng C Mater Biol Appl. 2016;68:919–928. doi:10.1016/j.msec.2016.07.049.
- Taher Z, Legge C, Winder N, et al. Magnetosomes and magnetosome mimics: preparation, cancer cell uptake and functionalization for future cancer therapies. Pharmaceutics. 2021;13(3):367. [PubMed: 33802121]. doi:10.3390/pharmaceutics13030367.
- Khosroshahi ME, Asemani M. Synthesis, characterization and imaging of fluorescine isothiocyanate conjugated magnetite nanoparticles in MCF 7 breast cancer cell lines. Int J Nanomater Nanotechnol Nanomed. 2017;3:044–050. doi:10.17352/2455-3492.000020].
- Mickoleit F, Jörke C, Geimer S, et al. Biocompatibility, uptake and subcellular localization of bacterial magnetosomes in mammalian cells. Nanoscale Adv. 2021;3(13):3799–3815. [PubMed: 34263139]. doi:10.1039/d0na01086c.
- Wang P, Chen C, Chen C, et al. The interaction of bacterial magnetosomes and human liver cancer cells in vitro. J Magn Magn Mater. 2017;427:105–110. doi:10.1016/j.jmmm.2016.10.106].
- Muthana M, Kennerley AJ, Hughes R, et al. Directing cell therapy to anatomic target sites in vivo with magnetic resonance targeting. Nat Commun. 2015;6(1):8009. [PubMed: 26284300]. doi:10.1038/ncomms9009.
- Salimi M, Sarkar S, Fathi S, et al. Biodistribution, pharmacokinetics, and toxicity of dendrimer-coated iron oxide nanoparticles in BALB/c mice. Int J Nanomed. 2018;13:1483–1493. [PubMed: 29559777]. doi:10.2147/IJN.S157293.
- Wahajuddin, S.A. & Arora, S. (2012) Superparamagnetic iron oxide nanoparticles: magnetic nanoplatforms as drug carriers.Int J Nanomedicine, 7, 3445–3471 [] [PubMed: 22848170]. doi:10.2147/IJN.S30320.
- Poon W, Zhang YN, Ouyang B, et al. Elimination pathways of nanoparticles. ACS Nano. 2019;13(5):5785–5798. [PubMed: 30990673]. doi:10.1021/acsnano.9b01383.
- Alphandéry E, Idbaih A, Adam C, et al. Biodegraded magnetosomes with reduced size and heating power maintain a persistent activity against intracranial U87-Luc mouse GBM tumors. J Nanobiotechnol. 2019;17(1):126. doi:10.1186/s12951-019-0555-2.
- Agashe HB, Dutta T, Garg M, et al. Investigations on the toxicological profile of functionalized fifth-generation poly (propylene imine) dendrimer. J Pharm Pharmacol. 2006;58(11):1491–1498. [PubMed: 17132212]. doi:10.1211/jpp.58.11.0010.
- Andreev SM, Babakhin AA, Petrukhina AO, et al. Immunogenic and allergenic properties of fulleren conjugates with aminoacids and proteins. Dokl Biochem. 2000;370(1–6):4–7. [PubMed: 11977250].