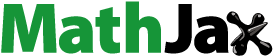
ABSTRACT
Additive manufacturing is a fabrication technique where materials are built up layer-by-layer allowing the realization of complex designs. Ceramic additive manufacturing is steadily increasing in the market and industries are developing 3D printers that are economical and efficient to print single/multi-material. The development of free-form extrusion-type printers has been of great significance due to their wide range of materials used, availability of technology, low development cost, and design freedom in developing the structures. We have successfully developed a hybrid multi-head 3D printer with four print heads capable of operating individually or together to print multiple layers using different materials. The three mechanically actuated print heads are provided to print ceramic, metallic slurry, and polymeric materials, and one pneumatic actuated print head is for printing bio-ink. The developed hybrid multi-head 3D printer can be beneficial in printing a wide range of materials such as metals, ceramics, polymers, and composites due to its flexibility in printing materials of different viscosities at different pressure. The optimization of the printing of calcium silicate bio-ceramics and multi-material is also studied and reported.
1. Introduction
The ceramic additive manufacturing sector is a booming industry with $402 million in revenue generated worldwide in 2021, even though the pandemic struck the economy badly and it is expected to reach $3.678 billion by 2028 [Citation1,Citation2]. High-performance ceramics are used in diverse applications for their desirable properties like high hardness, resistance to heat and abrasion, corrosion, and oxidation, in addition to electrical and thermal insulation effects [Citation3,Citation4]. The ceramic additive manufacturing process can be broadly categorized into Vat polymerization, Material Extrusion, Powder Bed Fusion, Binder Jetting, and Material Jetting [Citation5]. Techniques such as Vat polymerization, Binder jetting, and powder bed fusion have certain disadvantages associated with the high equipment cost, limitations associated with particle size and particle size distribution, and high material waste. Whereas, material extrusion-based systems are economical and combat material waste. In the material extrusion-based additive manufacturing technique the material is extruded through a nozzle under the applied mechanical force coupled with temperature and pneumatic pressure. Some of the material extrusion-based techniques include fused filament deposition (FDM) and robocasting/direct ink writing [Citation6]. Robocasting is established as a more suitable method for 3D printing with the leverage to use a wide range of materials, and the ability to print smaller to largest-sized parts with good precision. It can facilitate multi-material printing with the incorporation of multiple print heads, multiple materials can be printed at different layers at the same time. Powder particles with a heterogeneous size distribution can be printed by optimizing the viscosity of the employed slurries. Most robocasting-based printers available in the market consist of single extruder heads and a limited pressure range. Few multi-material print head designs are available in the literature, and the merits and demerits of the reported designs are discussed as follows. A single print head with multiple inlets for material administration multi-material printing is enabled by making multiple material holders that are connected to a single extruder through which different materials flow at different times or all the materials can be extruded together. Some multi-material printers have two material inlets through which two different materials are mixed using a mixer blade to form functionally graded materials. This model comes with a bulky head design and cannot print multiple materials on multiple layers. Another printer design with three different material inlets M1, M2, and M3 pushed out of the nozzle using a screw extruder. It offers to print highly viscous materials but is difficult to control the flow control while printing subsequent materials. In a simple screw-based extruder system, multiple material inlets were placed adjacent to the extruder pipe. This printer has few parts compared to other designs, but controlling the inlet flow pressure is difficult. In another printer design with three material inlets M1, M2, and M3, the flow is controlled by the pneumatic piston arrangement. It creates different dynamics inside the extruder causing inconsistent material flow [Citation7].
Though single-head multi-material printer arrangement has a simple design and construction, they cannot precisely control the flow pressure for each inlet, and printing functionally graded material is not possible. Wenbin Li et al used the CODE (Ceramic On-demand extrusion) based printer to print functionally graded materials using a dynamic mixing tool head, but it lacks multi-material multi-layer printing. Thus, there is a need for developing a 3D printer that is efficient in printing different materials simultaneously [Citation8]. A 3D multi-material plotting system developed at Fraunhofer IWS Dresden has three print heads arranged linearly that move along 3 axes. This design occupies more space and extrudes with the maximum pressure of 2.2 bars, thus not suitable for high viscous material extrusion [Citation9]. When the printer has linearly arranged print heads, there is a limitation in printing multi-materials. When the first and last heads are operated in a single print cycle, the print heads require more travel time with the linear head setup [Citation10]. In some contemporary printer machines, the arrangement of each extruder along the X-axis reduces the maximum range it can have along X-axis, thereby effectively reducing the print volume [Citation11]. R-GEN and BIO-X are bio-printers specifically designed for catering the tissue engineering applications. They are capable of printing only low viscous materials and are not suitable for high viscous ceramic slurries [Citation12]. The printing operation includes the application of an exact amount of pressure, usage of the correct nozzle size, speed of the print head movement, and amount of material delivered [Citation13,Citation14]. Considering the advantages and disadvantages associated with the slurry-based 3D printers, available in the market, a hybrid multi-material, multi-layer Robocasting type 3D printer was developed and reported in this paper. Robocasting-type printers are generally equipped with three different types of material extrusion systems simple pneumatic extruders, piston-actuated, or screw-based extruders. A pneumatic extruder involved the application of compressed air applied for material extrusion the material. The rate of extrusion can be varied by regulating the air pressure. This type of extruder system can be easily incorporated into multiple head printers. But it is mostly suitable for extruding low viscous materials than high viscous materials. A piston-actuated extruder is one where the material extrusion is controlled by a solenoid input. The rate of extrusion is varied by changing the solenoid input. It requires multiple motors for operating a single print head, thereby increasing the printing payload. In a screw-assisted extruder system, the screw is actuated by a stepper motor, and the material is pushed out through a nozzle [Citation15,Citation16]. This extruder arrangement ensures constant extrusion of even highly viscous materials in comparison to pneumatic and piston-assisted extruders [Citation17].
compares the important parameters of some of the commercially available ceramic 3D printers. Among the below-mentioned ceramic 3D printers, StoneFlower 4.0 3D printer, Delta WASP 2040 Clay, and 3DPOTTER 3D PotterBot 10 XL are single-head extruder systems capable of printing one material at a time. Pollen AM Pam series MC, Rapidia 3D printer, VormVrij BRUTUM, and Lynxter S600D are dual-head extruder printers that can print more than one material at a time.
Table 1. Comparison of commercial robocasting printers with hybrid multi head 3D bio-printer.
In this work, the optimization and 3D printing of calcium sulfate (CS) based scaffold structures were performed using the developed printer. Additive manufacturing of CS-based bone scaffolds by Robocasting or Direct ink writing is extensively reported for its ability to produce scaffolds with interconnected porosity for bone regeneration applications [Citation22,Citation23]. CS is reported to have good osteoconductivity, osteoinductivity, and biocompatibility thus making it an ideal candidate for bone regeneration applications [Citation24]. The release of Ca and Si from the incorporated CS is reported to extensively regulate cell proliferation and osteoblast differentiation [Citation25].
2. Methodology
2.1. Product definition, concept, and design
We adopted a product development strategy suggested by Ulrich and Eppinger [Citation26] to develop the hybrid 3D Bio-printer with multiple print heads. The product development process begins with product definition based on the need, then conceptual design, followed by detailed design with product specifications, fabrication, and finally printing of ceramic structures.
The user requirements of 3D printers are listed with proper definitions and the option for the functional requirement is identified [Citation27]. Then, the final product specification is selected based on cost, and technological feasibility (). Based on the final product speciation, the conceptual design is formulated ().
Table 2. User-oriented product definition and specification.
2.2. Machine specification
The objective was to develop an extrusion-type 3d printer capable of printing multi-materials on multiple layers with a print resolution of 5Х5Х5 mm.
The entire machine system is made up of sub-systems such as a material extrusion system, material dispensing system, positioning system, purification system, Interface and controls, and casing as shown in . The machine specification of the hybrid multi-head Bio-printer is shown in .
Figure 2. CAD model of (A) mechanical-pneumatic extruder assembly, (B) Z- gantry support column, (C) bed assembly, (D) X- gantry with extruder assembly, and (E) fully assembled hybrid multi-head 3D printer.

Table 3. Machine specification.
Design for Assembly concept [Citation28,Citation29] [has been employed in the designing of the reported printer. The developed printer has been designed with a diagonal arrangement (45°) print head arrangement and combined YZ bed assembly. Whereas in most printers, the Y and Z gantry is designed separately and requires more components and separate actuators in comparison to the combined YZ bed assembly design used in our printer. Also, in multi-material printers, print heads are arranged linearly, increasing the gantry length. Therefore, arranging of print heads diagonally at 45° to each other in the X-gantry brings counterbalance to the printer, with equal load distribution, and reduces the torsional stiffness. The diagonal arrangement of print heads has reduced the X gantry length by 35% and thereby increasing the print volume to machine build volume ratio. This gantry arrangement makes our printer more compact than other multi-head printers with reduced manufacturing cost, machining difficulty, and wide application range [Citation27]. Design optimization with reduced components, use of self-fastening features, and modular design have helped reduce design complexity, development time, and cost of the developed printer [Citation26]. Also, the following design steps have simplified the printing operation,
The print heads move only along the X-axis movement and the bed moves along Y-axis and Z-axis thus making homing and printing process simpler and more efficient.
The extruder and feeder setup are assembled with a simple self-locking feature which makes the cleaning process easy and less time-consuming. As the employed slurry needs to be properly cleaned post-printing to avoid contamination or clogging due to the dried slurry while printing a different slurry.
The Delrin plastic lining of the auger-screw wall makes the removal and cleaning process easy.
Self-leveling screws provided in the print bed effectively level the bed before printing.
Personnel and machine safety is important to be followed while operating the printer. Therefore, design for Safety concepts incorporated in the design are listed as follows,
The UV light source is provided with an ON/OFF switch is used to protect the working individual from direct exposure to UV rays.
The printer interface is provided with the STOP option to discontinue the printing process at the time of emergency.
Limit switches provided at the ends of the X, Y, and Z-axis to prevent the collision of the print heads and bed.
2.3. Material extrusion unit
The material extrusion system in the developed printer consists of three hybrid pneumatic-mechanical extruders and a pneumatic extruder.
2.3.1. Hybrid pneumatic-mechanical extruders
The hybrid pneumatic-mechanical extruder arrangement includes an auger screw, extruder pipe, syringe, syringe holder that holds the syringe in position, NEMA17 stepper motor, motor shaft, motor mount, sleeve bearing, nozzle, and heater. The viscous slurry is loaded into the syringe and is transferred to the extruder pipe through a 6 mm hose upon applying external pneumatic pressure. The rotating auger screw actuated by the stepper motor helps in the homogenous extrusion of the slurry through the nozzle.
The extruder pipes are fitted with an O-ring lined with Derlin plastic to restrict the reverse flow of material due to the back pressure. It also reduces the height of the extruder by about 45%, making the head more compatible. An induction heater coil is positioned coaxially, at the bottom of the extruder pipes, just 5 mm above the nozzle to extrude high melting point materials up to 200°C. Metallic and plastic tapper nozzles of various diameters can be easily fitted to the end of the extruder pipe easy.
The auger screw assembly ensures steady state extrusion of highly viscous materials ensuring a smooth and constant flow of material. This assembly could print different materials such as metals, ceramics, polymers, or hydrogels in the form of slurry/paste, provided it has sufficient flow, viscosity, and homogeneity. The appropriate auger screw configuration and actuation are said to enhance flow consistency, extrusion speed, and product quality. Albar et al used Auger screw-based extruder in a 3D printing system for the continuous output of materials [Citation30]. The screw extrusion system allows for more pressure buildup than FDM thereby increasing the rate of extrusion. These screw extruders favor the use of smaller nozzle diameters, therefore increasing the resolution of the prints. The reduction in screw size is also said to improve print speed [Citation31]. The production diagram of the Auger screw used in our printer is shown in and design specifications of the same are given in .
Understanding the advantages and disadvantages of the reported extruder assemblies, screw-extruder assemblies exhibiting less shear rate in extrusion and pneumatic extruder assemblies have been incorporated in the developed hybrid multi-material multi-layer 3D printer. For their ability to print a wide range of highly viscous and low viscous materials.
2.3.2. Pneumatic extruder
The fourth pneumatic extruder is a simple setup consisting of the dispensing syringe. The slurry/paste to be extruded is taken in the syringe and external pneumatic pressure is applied to directly extruded through the fitted plastic tapper nozzle. Additionally, the syringe is fitted with a removable UV light to cure the photo-polymeric materials shows the UV curing light assembly was specially designed for the developed printer and mounted on a holder developed using FDM ().
2.4. Fabrication
The fabrication of a hybrid multiple-head 3D printer includes several components that are additively manufactured, conventionally manufactured and some procured. The syringe holder, UV light holder, and limit switch were made of acrylonitrile butadiene styrene (ABS) and 3D printed using FDM technology. The components included in the extruder assembly like the motor mount, screw bearing, and induction heater, made of stainless steel were manufactured using a CNC milling process. The extruder pipe is made of aluminum and was manufactured using CNC turning process. A specially designed Auger screw, made of stainless steel was manufactured using CNC turning process. The components such as the X-gantry rail and Y-gantry rail, and rail support column are made of aluminum and were manufactured using the aluminum extrusion process and cut into the required dimension using a precision cutting process. The bed mount, bed base, and stiffener are made of aluminum and were fabricated using CNC milling and precision cutting processes. The body/enclosure of the printer is manufactured from aluminum using CNC bending, Laser cutting, and powder coating processes. The door of the printer enclosure has a glass window made of acrylic plexi glass sheet manufactured using a laser cutting process. It filters out 98% of damaging UV rays thereby protecting the user from direct UV exposure. Other components used in the assembly were procured and the components are assembled using suitable fasteners. The aluminum enclosure also houses a HEPA filter, that is employed to keep the filter free from dust particles, contaminants, and micro-organisms. Four global cooling systems made of 24 V (120 × 120 x 60 mm) DC brushless cooling fans are also present to circulate air into the chamber of the printer. The Philips round UV lamp (wavelength = 265 nm) is also incorporated for sterilization purposes for 3D bio-printing applications.
shows the assembly of the Hybrid multi-head 3D bio-printer with X-gantry, extruder assembly, enclosure, and YZ bed assembly. shows the multi-heads in operation facilitating multi-material printing. The electronic circuit assembly positioned at the rear side of the machine controls the X-gantry, YZ bed assembly, extruder heads, and other parts on the front side of the machine. The fabricated 3D printer is subjected to various performance tests such as multi-material printing, and optimization of printing parameters according to material properties. The details of the printing studies were discussed in section 5.
2.5. Printing optimization studies
For 3D printing of bio-ceramic scaffold structures, the above-designed extrusion type printer was used. In order to achieve effective 3D printing of bio-ceramic bone scaffolds, a highly viscous ceramic slurry is prepared using calcium silicate (CS) and calcium phosphate (CP) along with carboxymethyl cellulose (CMC) as a binder. The CS is purchased from Sigma Aldrich, India having a mesh size of 200, and CP is purchased from SD Fine, India Limited having a mesh size of 230. The binder material CMC is purchased from SD Fine, India Limited. The binder solution is prepared by having 2.3 wt% of CMC powder mixed with 10 ml of distilled water and stirred using a magnetic stirrer at 750 rpm for 20 min with a temperature of 80°C. After allowing it to cool down for 30 min, this solution is used as a binder. The 64 wt% of CS is mixed with 36 wt% of CMC solution using a mechanical stirrer (Remi 122/D) until it mixes uniformly. Similarly, 52 wt% of CP is mixed with 48 wt% of CMC solution to get a homogenous mixture favorable for printing. Following with rheological behavior of the slurry and the optimization of printing parameters such as nozzle diameter, extrusion pressure, extrusion amount, print speed, strand stability, and infill ratio were carried out.
2.5.1. Rheological characterization
The rheological behavior of the prepared CS and CP bio-ceramic slurries was estimated using RST Rheometer (Ametek, Brookfield). The prepared slurries were tested using a parallel plate with cross-hatch geometry. And the change in viscosity of the prepared slurries concerning increasing shear rate (0–1000 s-1) was estimated.
2.5.2. Nozzle diameter optimization
It is important to identify the suitable nozzle for Robocasting to achieve proper and continuous extrusion of the slurry to achieve proper structures. Therefore, the prepared CS and CP slurries were extruded using nozzles of different diameters, and strands were deposited. The nozzle diameters employed in this study were 0.26, 0.41, 0.6, 0.84, and 1.2 mm.
2.5.3. Strand stability test
This study was performed to determine the stability of strands deposited with different nozzle diameters. CS and CP slurries were extruded using different nozzle diameters (ND) and the strands were deposited on an FDM-printed structure with pillar-like structures methodically spaced at 1, 2, 4, 8, and 16 mm intervals [Citation32].
2.5.4. Extrusion pressure optimization
From the strand stability test, it was identified that the extrusion volume of the slurry increases with an increase in nozzle diameter. Therefore, CS slurry was extruded using a 0.6 mm nozzle at different extrusion pressures such as 0.05, 0.1, 0.15, 0.2, 0.25, 0.3, 0.35, 0.4, 0.45, and 0.5 MPa. Constant extrusion pressure was applied continuously for 5 seconds using the pedal connected to the dispensing unit. 0.6 mm nozzle exhibiting steady and continuous extrusion of the slurry and depositing stable strands was chosen to determine the extrusion volume at various extrusion pressure in this study. The volume of the slurry extruded was weighed to calibrate the extrusion volume concerning the applied extrusion pressure.
2.5.5. Print speed optimization
The diameter of the deposited strand depends on the speed at which the nozzle travels. Slower nozzle movements could lead to the deposition of large volumes of material at a particular region thereby increasing the diameter of the deposited strand. At the time deposition of discontinuous strands could be observed as a result of faster nozzle movements. Therefore, to optimize the ideal print speed for printing using the developed printer, different print speeds of 1, 5, 10, 15, and 20 mm/s were optimized in this experiment.
2.5.6. Filament fusion test
In order to access the fusion of the deposited filaments leading to pore closure a filament fusion test reported by Ribeiro et al. was adopted in this study [Citation32]. Following this, a pattern consisting of parallel lines with increasing spaces was created. A 0.5 mm increment was set between the parallel lines.
2.5.7. Repeatability and accuracy
The repeatability and accuracy of the printed structures were quantified as follows,
Repeatability of the prepared slurry (Eq 1),
(1)
Accuracy of the printed scaffold structures (Eq 2),
Where AV is the actual value of the CAD model dimensions and OV is the obtained values of scaffold structure dimensions [Citation33].
2.6. Sintering optimization
The developed structures were sintered using a microwave furnace (VB Ceramics, India) to impart strength. To identify the optimal sintering temperature, the CS and CP structures were sintered at three different temperatures. CS structures were sintered at 1000, 1100, and 1200°C, and CP was sintered at 1100, 1200, and 1300°C. The sintering temperatures for the developed bio-ceramic structures were chosen based on the literature. Since microwave sintering is a known fast sintering technique, a 5 min dwell time was observed to be sufficient to harden the developed structures at the reported temperatures [Citation34].
3. Results and discussion
The obtained results demonstrate that the prepared slurries of CS and CP exhibit a shear-thinning behavior. The viscosity of the administered slurries was found to reduce under increasing shear rate. Thus, the characteristics exhibited by the prepared slurry were considered optimal to achieve uniform extrusion under the applied pressure through the administered nozzle. The initial viscosity recorded for CS and CP slurries were found to be 2905 and 1896 Pa., respectively ().
It was identified that the prepared CS and CP slurries were easily extrudable using a nozzle diameter of 0.41 mm and above. It was completely in-extrudable while using a 0.26 mm nozzle due to its very narrow tip. On the other hand, even though the slurry was extrudable using a 0.41 mm nozzle, the flow was not continuous. The diameter of CS () and CP () strands deposited using different nozzle diameters were examined using a digital microscope and calibrated using ImageJ software.
The stability of the strands was examined using nozzles of different diameters. In , the CS and CP strands deposited using the wider diameter nozzles (1.2 and 0.84 mm) exhibited sagging at the maximum interval of 16 mm. When closely observed, the strand deposited using a 1.2 mm nozzle diameter was found to sag even at smaller intervals of 4 mm and 8 mm. When the nozzle diameter was reduced to 0.6 and 0.41 mm, the sagging was not noticed. This experiment further helps us to understand that with an increase in nozzle diameter, the extrusion volume also increases, thereby compromising the stability of the printed structures. And as reported earlier, no extrusion was observed with a 0.26 mm nozzle.
The extrusion volume was zero for extrusion pressure below 0.2 MPa. At the extrusion pressures of 0.2 MPa and above 0.2 MPa, the extrusion volume was found to steadily increase with the increase in extrusion pressure (). This shows that it is necessary to identify the ideal extrusion pressure to obtain stable print structures. An increase in extrusion pressure may lead to over-extrusion of the material resulting in inaccurate printing. The extrusion study was carried out for CP slurry, and it was identified that ideal extrusion was achieved at an extrusion pressure of ~ 0.2 MPa ().
The printing speed is another factor governing the accuracy of the printed structures. It was observed that when the print speed was set as 1 mm/s, very slow nozzle movement lead to over extrusion of CS under the applied constant pressure. The deposited strand was 5.8 times wider than the actual nozzle diameter (0.6 mm). The strand diameter was observed to decrease with an increase in print speed. The last strand diameter of 0.54 mm was observed for a print speed of 20 mm/s. At 20 mm/s, the deposited strand was observed to be stretched due to the fast movement of the nozzle that eventually reduce the diameter of the deposited strand. The results demonstrated that at a print speed of 15 mm/s, the average diameter of the deposited strand achieved (0.62 mm) was close to the actual nozzle diameter (0.6 mm) (). Similar results were observed when using the CP slurry. The deposited strand diameter of 0.65 mm close to the actual nozzle diameter of 0.6 was observed at a print speed of 15 mm/s for CP slurry (). Therefore, 15 mm/s has been identified as the ideal print speed.
Figure 10. Average diameter of strands deposited at different print speeds (A) CS and (B) CP slurries.

The filament fusion test was conducted to optimize the distance between the strands which leads to a unique infill arrangement (). The results demonstrated that the deposited strands were observed to fuse when the spacing between them was 0.5 mm, and they refrained from fusing when a gap of 1 mm and above was maintained. shows the filament fusion test conducted for CS and CP, respectively.
From the above-performed optimizations, a 0.6 mm nozzle was chosen as the ideal nozzle diameter, extrusion pressure as 0.2 MPa for the prepared CS-CMC, with a print speed as 15 mm/s. These parameters were employed to print cubic scaffold structures of 10 × 10 × 10 mm and 10 × 10 × 1.5 mm with 50% infill (). Similarly, CP () structures were also printed using a 0.6 mm nozzle at an extrusion pressure range between 0.2 and 0.25 MPa. The dimensions were set as 10 × 10 × 10 mm, at a constant print speed of 15 mm/s with 50% infill. The repeatability and accuracy achieved in the printing of CS and CP are listed below in .
Table 4. Repeatability and accuracy of the printed CS and CP structures.
These optimized parameters were further employed in developing a femur bone section using CS as displayed in .
Upon performing compressive strength analysis for 10 × 10 × 10 mm CS and CP structures using a universal testing machine (INSTRON, 8801), it was identified that better strength was achieved at specific temperatures for each material. For instance, maximum compressive strength of 15.54 and 9.9 MPa was recorded for CS 100 and 50% infill structure sintered at 1200°C. CP 100 and 50% infill structures sintered at 1200°C exhibited a maximum compressive strength of 8.6 and 7.1 MPa, respectively. represent the surface of the sintered CS and CP structures at 1000 X.
Figure 14. (A) and (B) are the surfaces of CS and CP sintered structures recorded using FESEM at 1000X.

Multi-material printing refers to developing structures with two or more materials. The developed printer enables the development of multi-material structures with the aid of screw-assisted hybrid extruders. To demonstrate the multi-material printing, the undyed calcium silicate paste (Material A) and the calcium silicate paste dyed with natural yellow dye (Material B) were taken in two different print heads 1 and 2, respectively, and a structure was printed. Material A was used to print the outer dimensions of the structure and the infill patterns were printed using Material (B) as shown in .
The advantages and disadvantages of the hybrid multi-head printer are discussed below in ,
Table 5. Advantages and disadvantages of the developed printer.
4. Conclusion
The hybrid multi-head bio-printer was successfully developed meeting all the defined needs. The developed Extrusion deposition bio-printer demonstrated its ability to print architecture porous green scaffold for bone tissue regeneration applications for the selected bio-ceramic material like CS and CP. The issues related to printing the structures with various polymers are also discussed in detail. The hybrid print head design facilitates multi-material multi-layer printing and a pneumatic extruder is used for low-viscosity bio-inks. The scaffolds are developed by optimizing the printing parameters such as nozzle size, nozzle travel, and extrusion rate. The high accuracy, printability, and repeatability of the scaffold structures with CS and CP were successfully demonstrated with 2.3 wt% CMC in hydroxyapatite and calcium silicate. Besides, microwave sintering of the developed structures was found to effectively impart mechanical strength to the developed structures. The multi-material printing capability was also successfully tested by printing material A in the outer core and material B in the inner core of a square. The combination of material adaptability and versatility in printing precise design constructs has proved the ability of the hybrid multi-head printer as a potential candidate for developing structures in bone tissue regenerative applications. Bio-ceramic scaffolds, multi-material scaffolds, and Bio ink-based scaffolds are the outcomes that can be developed with the extrusion deposition process.
Acknowledgments
The development of this Muti-Material Multi-Layer ceramic 3D Printer was funded by the Science and Engineering Research Board of India (DST-SERB), under its Start-up Research Grant (SRG) scheme [Grant number: SRG/2019/002038].
Disclosure statement
No potential conflict of interest was reported by the author(s).
Additional information
Funding
References
- Tofail SAM, Koumoulos EP, Bandyopadhyay A, et al. Additive manufacturing: scientific and technological challenges, market uptake and opportunities. Mater. Today. 2018;21(1):22–37. doi: 10.1016/j.mattod.2017.07.001
- Ceramics 3D Printing Market Revenues to Top 3.6 Billion by 2028, (n.d.). [accessed February 23, 2022]. Available from: https://www.smartechanalysis.com/news/ceramics-3d-printing-market/.
- Balasubramanian S, Gurumurthy B, Balasubramanian A. Biomedical applications of ceramic nanomaterials: a review. Int J Pharm Sci Res. 2017;8:4950–4959. doi: 10.13040/IJPSR.0975-8232.8(12).4950-59
- Goffard R, Sforza T, Clarinval A, et al. Additive manufacturing of biocompatible ceramics. Adv Prod Eng Manag. 2013;8(2):96–106. doi: 10.14743/apem2013.2.157
- Chen Z, Li Z, Li J, et al. Journal of the European ceramic society 3D printing of ceramics: a review. J Eur Ceram Soc. 2019;39(4):661–687. doi: 10.1016/j.jeurceramsoc.2018.11.013
- Faes M, Valkenaers H, Vogeler F, et al. Extrusion-based 3D printing of ceramic components. Procedia CIRP. 2015;28:76–81. doi: 10.1016/j.procir.2015.04.028
- Abilgaziyev A, Kulzhan T, Raissov N, et al. Design and development of multi-nozzle extrusion System for 3D printer. 2015. doi: 10.1109/ICIEV.2015.7333982.
- Li W, Martin AJ, Kroehler B, et al., Fabricating functionally graded materials by ceramic on-demand extrusion with dynamic mixing. Solid free. Fabr. 2018. Proc. 29th Annu. Int. Solid Free. Fabr. Symp. - An Addit. Manuf. Conf. SFF 2018. Austin, TX; 2018. p. 1087–1099. https://scholarsmine.mst.edu/cgi/viewcontent.cgi?article=3576&context=matsci_eng_facwork.
- Lode A, Meissner K, Luo Y, et al. Fabrication of porous scaffolds by three-dimensional plotting of a pasty calcium phosphate bone cement under mild conditions. J Tissue Eng Regen Med. 2012;8(9):682–693. doi: 10.1002/term
- Kačarević ŽP, Rider PM, Alkildani S, et al. An introduction to 3D bioprinting: possibilities, challenges and future aspects. Materials. 2018;11(11):2199. doi: 10.3390/ma11112199
- Daramwar V, Kadam S. Design and developemet of multi-material extrusion in FDM 3D printers. Int Res J Eng Technol. 2020;7:3634–3641.
- Tong A, Pham QL, Abatemarco P, et al. Review of low-cost 3D bioprinters: state of the market and observed future trends. SLAS Technol. 2021;26(4):333–366. doi: 10.1177/24726303211020297
- Berndsen JC, Forcellini F, Barbosa SB, et al. Design for Service (DFS) in the product development process (PDP). Braz. J. Dev. 2021;7(1):2627–2645. doi: 10.34117/bjdv7n1-179
- Rafiee M, Farahani RD, Therriault D. Multi-material 3D and 4D printing: a survey. Adv Sci. 2020;7(12):1902307–26. doi: 10.1002/advs.201902307
- Guo C, Zhang M, Bhandari B. A comparative study between syringe-based and screw-based 3D food printers by computational simulation. Comput Electron Agric. 2019;162:397–404. doi: 10.1016/j.compag.2019.04.032
- Manoel J, Netto J, Idogava HT, et al. Screw-assisted 3D printing with granulated materials: a systematic review. Int J Adv Manuf Technol. 2021;115(9–10):2711–2727. doi: 10.1007/s00170-021-07365-z
- Drotman DTJ. Design of a screw extruder for additive manufacturing. Master’s thesis. University of California, San Diego. 2015. https://escholarship.org/uc/item/5142579v.
- 3D Potterbot 10XL Ceramic 3D Clay Printer — Real Clay 3D Ceramic Printer - 3D Potter, (n.d.). [accessed March 1, 2022]. Available from: https://3dpotter.com/printers/potterbot-10-xl.
- Best Ceramic 3D Printers of 2022 | All3DP Pro, (n.d.). [accessed March 1, 2022]. Available from: https://all3dp.com/2/ceramic-3d-printer-ceramic-3d-printing/.
- Ceramic 3D printer 2022 - Guide and hardware selection, (n.d.). [accessed March 1, 2022]. Available from: https://www.aniwaa.com/buyers-guide/3d-printers/ceramic-3d-printer/.
- BRUTUM® Concept Large 3D Clay Printer. VormVrij. 2020. https://vormvrij.nl/download/datasheets/datasheet-BRUTUM.pdf.
- Xiurong Ke LZ, Qiu J, Wang X, et al. Modification of pore-wall in direct ink writing wollastonite scaffolds favorable for tuning biodegradation and mechanical stability and enhancing osteogenic capability. FASEB J. 2020;34(4):5673–5687. doi: 10.1096/fj.201903044R
- Shao H, Yang X, He Y, et al. Bioactive glass-reinforced bioceramic ink writing scaffolds: sintering, microstructure and mechanical behavior. Biofabrication. 2015;7(3):35010. doi: 10.1088/1758-5090/7/3/035010
- Hao F, Qin L, Liu J, et al. Assessment of calcium sulfate hemihydrate–tricalcium silicate composite for bone healing in a rabbit femoral condyle model, mater. Sci Eng C. 2018;88:53–60. doi: 10.1016/j.msec.2018.02.024
- Shie MY, Ding SJ, Chang HC. The role of silicon in osteoblast-like cell proliferation and apoptosis. Acta Biomater. 2011;7:2604–2614. doi: 10.1016/j.actbio.2011.02.023
- Ulrich KT, Eppinger SD, Yang MC. Product design and development. Seventh ed. New York (USA): McGraw-Hill Education; 2020.
- Ponche R, Mognol P, Kerbrat O, et al. A new global approach to design for additive manufacturing to cite this version: a new global approach to design for additive manufacturing. Virtual Phys Prototyp. 2012;7(2):93–105. doi: 10.1080/17452759.2012.679499
- Molloy O, Warman EA, Tilley S. Design for manufacturing and assembly: concepts, architectures, and implementation, First Edition. Berlin, Germany: Springer Science & Business Media; 2012. p. 220. doi: 10.1007/978-1-4615-5785-2
- Koraishy BM, Wood KL, Tilstra A. Des Manuf Assembly. 2011. doi: 10.1002/9780470400531.eorms0253
- Albar A, Chougan M, Kheetan M-J-A, et al. Results Eng effective extrusion-based 3D printing system design for cementitious-based materials. Results In Eng. 2020;6:100135. doi: 10.1016/j.rineng.2020.100135
- Bortolamasi M, Fottner J. Design and sizing of screw feeders. In: Proceedings of the Int. Congr. Part. Technol. Nuremberg, Germany. 2001;69:27–29. https://mediatum.ub.tum.de/doc/1188322/document.pdf
- Golafshan N, Vorndran E, Zaharievski S, et al. Tough magnesium phosphate-based 3D-printed implants induce bone regeneration in an equine defect model. Biomaterials. 2020;261:120302. doi: 10.1016/j.biomaterials.2020.120302
- Panaksri A, Tanadchangsaeng N. Evaluation of 3D-printing scaffold fabrication on biosynthetic medium-chain-length polyhydroxyalkanoate terpolyester as biomaterial-ink. Polymers. 2021;13(14):2222. doi: 10.3390/polym13142222
- Oghbaei M, Mirzaee O. Microwave versus conventional sintering: a review of fundamentals, advantages and applications. J Alloys Compd. 2010;494:175–189. doi: 10.1016/j.jallcom.2010.01.068