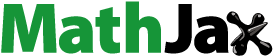
ABSTRACT
A barium titanium oxide/silver (BaTiO3/Ag) nanocomposite powder was synthesized using a sonochemical process. By irradiating an ethanol solution of BaTiO3 and silver oxide (Ag2O), Ag2O thermally decomposed at 40°C and Ag-loaded BaTiO3 nanocomposites powder was obtained. Nanocomposites containing 30 vol% Ag sintered at 1000°C showed near densities and higher dielectric constants compared to those of BaTiO3 sintered at 1300°C. Moreover, percolation did not occur because the Ag nanoparticles were uniformly dispersed and loaded onto the surface of BaTiO3. This sonochemical method is expected to be a new process for obtaining uniformly dispersed and loaded nanocomposite powders without percolation, even in large amounts.
1. Introduction
Barium titanium oxide (BaTiO3) is a perovskite-type crystal not found in nature. Since the discovery of the ferroelectric BaTiO3, its characteristics, and applications have been extensively studied [Citation1]. BaTiO3 has cubic, tetragonal, orthorhombic, and rhombohedral crystal structures. In particular, the tetragonal structure is crucial for the ferroelectric properties of BaTiO3. In the tetragonal structure, the Ti4+ exists in an off-center position, and the resulting spontaneous polarization in the crystal causes ferroelectricity [Citation2].
Multilayer ceramic capacitors (MLCCs) are applications of BaTiO3. MLCCs have a layered structure comprising dielectric and internal electrodes. This characteristic structure enables a large capacitance and downsizing of products [Citation3,Citation4]. Moreover, by compositing with metal, the dielectric constant and capacitance increase according to the effective dielectric field theory [Citation5] and internal electrode effects [Citation6], respectively. However, the manufacture of MLCC requires complicated processes and high sintering temperatures above 1200°C [Citation4].
From a practical perspective, its mechanical and electrical properties are important, with the nanocomposite technique being an effective method for these properties [Citation7–10]. Several studies have been conducted on BaTiO3 nanocomposites. For example, the fracture strength and toughness increases when silicon carbide is dispersed in BaTiO3 [Citation11]. Moreover, the nanocomposites of BaTiO3 and nickel improve the dielectric constant [Citation6,Citation12,Citation13].
Although nanocomposites exhibit many attractive characteristics, their synthesis requires multistep, high-temperature, and long-term processes. In general, nitrates, which lead to NOx generation, are required when synthesizing nanocomposites of metals and ceramics [Citation14–17]. Sonochemical processes are effective in overcoming these disadvantages. Ultrasound has a frequency above 20 kHz and cannot be heard by humans [Citation18]. Cavitation bubbles are generated when liquids are irradiated with ultrasound. The repeated rapid contraction and expansion caused by cavitation generates high temperature (~5000 K) and high pressure (~100 atm) hot spots [Citation19]. Hot spots can result in both physical and chemical effects. Physical effects include crushing and mixing caused by microjets, while chemical effects include the induction of radical reactions and thermal decomposition [Citation20]. Sonochemical processes with these effects have been used to synthesize nanoparticles [Citation21–23] and nanocomposites [Citation24–26].
In this study, we synthesized BaTiO3/Ag nanocomposites using ultrasonic irradiation. Silver oxide (Ag2O) was used as the starting material. Noble oxides such as Ag2O are readily reduced by heating without emitting toxic by-products, which can occur at room temperature under ultrasonic irradiation [Citation27]. It is expected that dispersing silver (Ag) nanoparticles enables low-temperature sintering by liquid phase diffusion and its high thermal conductivity, resulting in high dielectric constant [Citation28]. Therefore, we attempted to synthesize dielectrics for a chip capacitor with a high dielectric constant by sintering BaTiO3/Ag nanocomposites at temperatures lower than 1200°C.
2. Materials and methods
2.1. Materials
BaTiO3 (Purity 99.9%, Wako Pure Chemical Industries, Ltd.) and Ag2O (Purity 99%, Koujundo Chemical Laboratory Co., Ltd.) were used to synthesize the BaTiO3/Ag nanocomposites. Ethanol (C2H5OH) was used as the solvent. Dotite (HUJIKURA KASEI Co., Ltd.) was used as the Ag electrode to measure the dielectric constant.
2.2. Preparation of BaTiO3/Ag nanocomposites
shows the method used to prepare the BaTiO3/Ag nanocomposites. BaTiO3 and Ag2O were added to 100 mL C2H5OH in a flask. The volume of Ag was 10 or 30% of that of BaTiO3. The mixture was sonicated (44.13 kHz, 100 W) in air for 1 or 3 h using an ultrasound reactor (Honda Electronics Co., Ltd.). During sonication, the flask was placed in a constant temperature water bath maintained at 40°C using a low temperature circulator (CTP-3000, TOKYO RIKAKIKAI Co., Ltd.). The sonicated sample was dried under vacuum (30°C) to obtain the BaTiO3/Ag nanocomposite powder.
2.3. Forming and sintering pellets of BaTiO3/Ag nanocomposites
The nanocomposite powder was formed into pellets via uniaxial pressing at 200 MPa. The diameter of each pellet was 8 mm. Pellets were sintered at 1000 or 1100°C (5°C/min temperature ramp rate) for 3, 6, 9, and 12 h in air in an electric furnace. To compare with the effect of the BaTiO3/Ag nanocomposites, the BaTiO3 pellets were formed and sintered under the same conditions and at 1300°C for 6 h.
2.4. Characterization
The using powder X-ray diffraction (XRD) patterns of the BaTiO3/Ag nanocomposites were recorded using a RINT 2000 diffractometer (Rigaku Corporation). The surface morphologies of the BaTiO3/Ag nanocomposites and pellets were observed by field-emission scanning electron microscopy (FE-SEM, Model-S-48 Hitachi High-Technologies Corporation). The density of the pellets was measured using Archimedes’ method (n = 3).
2.5. Measurement of the dielectric constant
One side of the sintered pellets was coated with dotite to synthesize the Ag electrodes. An electrode was synthesized by sintering the pellets at 300°C for 1 h with a temperature ramp rate of 10°C/min in an electric furnace. The same procedure was performed on the other side of the pellets. The dielectric constants of the prepared pellets were measured using a precision impedance analyzer (Agilent 4294A, Agilent Technologies Ltd.). The temperature range of this measurement was 40 to 150°C and the frequency was 100 kHz.
3. Results and discussion
3.1. Sample characterization
The XRD patterns of BaTiO3/Ag nanocomposites containing 10 vol% Ag are shown in . For the sample sonicated for 1 h, certain peaks were assigned to Ag. This suggests that the thermal decomposition of Ag2O progressed. Ultrasound irradiation in ethanol produces OH radicals, which generates reducing agent of Ag2O. This chemical equation is shown below.
In addition to the sonochemical reduction shown above, thermal decomposition may be also considered. OH radicals are also generated during ultrasound irradiation of water, but the decomposition of Ag2O by ultrasound does not occur in water. The thermal decomposition temperature of Ag2O about 280°C, so it is considered that thermal decomposition by hot spots produced by ultrasound irradiation is dominant reaction in this study [Citation19,Citation27]. For the sample sonicated for 3 h, the peaks assigned to Ag2O disappeared, indicating that Ag2O completely decomposed. Peaks assigned to silver acetate (CH3COOAg) were observed in the samples sonicated for 3 h. Ultrasonic irradiation promoted the reaction between C2H5OH and Ag2O, so that Ag2O was reduced and C2H5OH was oxidized simultaneously. When C2H5OH is oxidized, acetic acid is formed via acetaldehyde. It was assumed that CH3COOAg was formed by the reaction of acetic acid with silver oxide [Citation29].
shows the XRD patterns of the BaTiO3/Ag nanocomposites containing 30 vol% Ag. Similar to the nanocomposites containing 10 vol% Ag, peaks assigned to Ag2O (1 h), Ag (1, 3 h), and CH3COOAg (3 h) are observed. The diffraction peaks of the BaTiO3/Ag nanocomposites containing 30 vol% Ag are higher in intensity than those of the BaTiO3/Ag nanocomposites containing 10 vol% Ag. This is attributed to an increase in the amount of Ag2O.
The morphologies and particle size distributions of the BaTiO3 and Ag nanoparticles of BaTiO3/Ag nanocomposites are shown in , respectively. The small Ag nanoparticles were uniformly dispersed and loaded onto the BaTiO3 surface. Hot spots produced by ultrasonic irradiation were generated randomly in the C2H5OH solution; thus, the heterogeneous nucleation of Ag on the surface of BaTiO3 was promoted while uniformly dispersing it. The size of the Ag nanoparticle increased with increasing Ag2O amount and ultrasonic irradiation time. This may have been due to an increase in the amount of reacted Ag2O.
3.2. Characterization of sintered BaTiO3/Ag nanocomposites
and show the relative densities of the sintered BaTiO3/Ag nanocomposites synthesized from the samples sonicated for 3 h and BaTiO3. The relative densities were calculated using the theoretical densities. The theoretical densities of BaTiO3 and the 10 and 30 vol% Ag BaTiO3/Ag nanocomposites were 6.08, 6.19, and 6.40 g/cm3, respectively. shows that the BaTiO3/Ag nanocomposite containing 10 vol% Ag sintered at 1100°C for 6 h has almost the same relative density as BaTiO3 sintered at 1300°C for 6 h; therefore, nanocompositing is an effective method to achieve low-temperature sintering.
Table 1. Relative densities (%) and porosities (%) of BaTiO3/Ag nanocomposites sintered at 1000°C.
Table 2. Relative densities (%) and porosities (%) of BaTiO3/Ag nanocomposites sintered at 1100°C.
The relative densities of the nanocomposites containing 10 vol% Ag were higher than these of the nanocomposites containing 30 vol% Ag at both sintering temperatures (1000 and 1100°C, , respectively). There are two reasons for the decrease in the density of the nanocomposites containing 30 vol% Ag. First, Ag leaked onto the pellet surface because of the high temperature and long sintering time. Ag was observed on the pellet’s surface of the 30 vol% Ag pellets sintered at 1100°C for 12 h. It is conceivable that Ag melted and moved to the pellet surface. Second, CH3COOAg was formed by ultrasonic irradiation (). shows the SEM images of the BaTiO3/Ag nanocomposite containing 30 vol% Ag. The rod-shaped CH3COOAg [Citation30] that is present before sintering () thermally decomposed after sintering (). As a result, it was inferred that rod-shaped vacancies formed in the nanocomposite, resulting in the density decrease.
Figure 6. SEM images of the BaTiO3/Ag nanocomposite containing 30 vol% Ag (a) Before and (b) after sintering at 1100°C for 6 h.

shows the SEM images of the 10 or 30 vol% Ag pellets sintered at 1100°C for 6 h and BaTiO3 pellets sintered at 1300°C for 6 h. The particle size of the BaTiO3/Ag nanocomposites was smaller than that of BaTiO3. It is assumed that the particle growth of BaTiO3 was suppressed by nanocompositing owing to the pinning effect of the Ag nanoparticles [Citation31,Citation32].
3.3. Mechanism of sintering nanocomposites
shows a schematic of the sintering of BaTiO3. Similar to general sintering, it proceeds through solid-phase diffusion. During sintering, particle growth progresses, and necks or pores form. The solid-phase diffusion rate is very slow; therefore, high-temperature and long-term reactions are required to synthesize densely sintered bodies [Citation33,Citation34]. In addition, smaller initial particle size results in higher relative density after sintering [Citation35]. In this study, BaTiO3/Ag nanocomposites sintered body have higher relative density than raw BaTiO3 sintered body. BaTiO3 is one of the ceramics and hard, so it is difficult to downsize the BaTiO3 particles by ultrasound irradiation. Thus, it is considered that initial particle size of BaTiO3 does not change with or without ultrasound irradiation in this study. We think that relative density increased by compositing with Ag nanoparticles. Detailed discussion is shown below.
A schematic of the sintering of the BaTiO3/Ag nanocomposites is shown in . Densely sintered BaTiO3/Ag nanocomposites were obtained by low-temperature sintering. This was attributed to the melting and then diffusion of Ag, during sintering (melting point: 962°C). Thus, BaTiO3/Ag nanocomposites were sintered in the liquid phase where rapid diffusion enables sintering at a lower temperature [Citation36]. Moreover, the higher thermal conductivities of Ag (429 W m−1 K−1) compared with BaTiO3 (6 W m−1 K−1) contributed to low-temperature sintering. Therefore, it was inferred that the addition of Ag caused faster thermal conduction, which progressed quickly to the interior of the pellet, resulting in low-temperature sintering. The growth of BaTiO3 particles was suppressed by the pinning effect of the Ag nanoparticles. However, Ag nanoparticles exist at the BaTiO3 particle interface, and the presence of Ag in the liquid state causes particle rearrangement. Thus, a dense and uniformly sintered body was obtained via low-temperature sintering. Furthermore, in general, contact flattening effects also contribute to densification in liquid phase sintering [Citation37]. Therefore, contact flattening of BaTiO3 by dissolution and precipitation in molten silver rarely occurs, and it is assumed that particle rearrangement is the main cause of densification.
3.4. Evaluation of electric characteristics
and show the dielectric constants of the samples sintered for 6 h. The dielectric constants of BaTiO3 and BaTiO3/Ag nanocomposites containing 10 vol% Ag increased with an increase in the sintering temperature. Additionally, the dielectric constants of the samples sintered at 1000°C increased with increasing Ag content. The dielectric constant of the BaTiO3/Ag nanocomposites containing 30 vol% Ag sintered at 1000°C is 9.2 × 103, which is larger than that of BaTiO3 sintered at 1300°C (6.2 × 103). This indicates that the nanocomposite fabrication technique is effective for lowering the sintering temperature and increasing the dielectric constant. The reason for the increase in dielectric constant with the addition of Ag nanoparticles can be attributed to the effective dielectric field theory and the contribution of the internal electrode effect. First, the dispersion of Ag nanoparticles in BaTiO3 is assumed to create an effective dielectric field around the Ag nanoparticles based on the Maxwell-Wagner effect. Therefore, it is presumed to increase the dielectric constant of sintered body. Second, the dispersed Ag nanoparticles in BaTiO3 behave like internal electrodes in MLCC. Thus, it is assumed that the addition of Ag nanoparticles increased the electrode area, resulting in an increase in the dielectric constant. Moreover, the BaTiO3/Ag nanocomposites containing 30 vol% Ag maintained their insulation. Thus, the nanocomposites prepared using sonochemical processes can uniformly disperse highly concentrated particles. Percolation occurred in the BaTiO3/Ag nanocomposites containing 30 vol% Ag sintered at 1100°C. It may be speculated that the high-temperature and long sintering time caused the melting and diffusion of Ag which resulted in the formation of conductive paths.
Figure 9. Temperature dependence of dielectric constant of 6 h sintered BaTiO3/Ag nanocomposites and BaTiO3.

Table 3. Dielectric constants of BaTiO3/Ag nanocomposites and BaTiO3 (sample sintered for 6 h).
In , peak shifts were observed. It is known that the particle size of BaTiO3 and residual stress can change the Curie temperature of sintered body [Citation38,Citation39]. One of the reasons for the peak shift is that the pinning effect of Ag nanoparticles inhibits grain growth, resulting in a decrease in grain size and residual stresses. In the sintering of BaTiO3 at 1000°C, the densification did not progress sufficiently due to the lower sintering temperature, causing a change in the particle size and accumulating residual stresses which is assumed to be the peak shift.
4. Conclusion
In this study, we successfully synthesized BaTiO3/Ag nanocomposite powders near room temperature without producing hazardous waste. Under ultrasonic irradiation, hot spots formed, and Ag2O decomposed. As a result, the Ag nanoparticles were uniformly dispersed onto the surface of BaTiO3 particles. The melting and high thermal conductivity of Ag led to a lower sintering temperature of BaTiO3/Ag nanocomposites than that of BaTiO3. Sintered BaTiO3/Ag nanocomposites containing highly concentrated Ag nanoparticles provided electrical insulation because the Ag nanoparticles were uniformly dispersed. The low-temperature sintered BaTiO3/Ag nanocomposites have a higher dielectric constant than BaTiO3 based on the effective dielectric field theory and internal electrode effects by dispersing Ag nanoparticles. Currently, the synthesis of a high-performance SDGs-oriented material is required. The synthesis of nanocomposite powders by sonochemical processes and their sintering at low temperatures are expected to achieve high performance and SDGs.
Disclosure statement
No potential conflict of interest was reported by the author(s).
Additional information
Funding
References
- Haertling GH. Ferroelectric ceramics: history and technology. J Am Ceram Soc. 1999;82(4):797–818. doi: 10.1111/j.1151-2916.1999.tb01840.x
- Buscaglia V, Randall CA. Size and scaling effects in barium titanate. An overview. J Eur Ceram Soc. 2020;40(11):3744–3758. doi: 10.1016/j.jeurceramsoc.2020.01.021
- Kishi H, Mizuno Y, Chazono H. Base-metal electrode-multilayer ceramic capacitors: past, present and future perspectives. Jpn J Appl Phys. 2003;42(1):1–5. doi: 10.1143/JJAP.42.1
- Pan MJ, Randall CA. A brief introduction to ceramic capacitors. IEEE Electr Insul Mag. 2010;26(3):44–50. doi: 10.1109/MEI.2010.5482787
- Kwan SH, Shin FG, Tsui WL. Dielectric constant of silver-thermosetting polyester composites. J Mater Sci. 1984;19(12):4093–4098. doi: 10.1007/BF00980776
- Hyuga H, Hayashi Y, Sekino T, et al. Fabrication process and electrical properties of BaTiO3/Ni nanocomposites. Nanostruct Mater. 1997;9(1–8):547–550. doi: 10.1016/S0965-9773(97)00121-9
- Niihara K. New design concept of structural ceramics –ceramic nanocomposites–. J Ceram Soc Jpn. 1991;99(10):974–982. doi: 10.2109/jcersj.99.974
- Lamouroux F, Bertrand S, Pailler R, et al. Oxidation-resistant carbon-fiber-reinforced ceramic-matrix composites. Compos Sci Technol. 1999;59(7):1073–1085. doi: 10.1016/S0266-3538(98)00146-8
- Fukahori S, Ichiura H, Kitaoka T, et al. Photocatalytic decomposition of bisphenol a in water using composite TiO2-Zeolite sheets prepared by a papermaking technique. Environ Sci Technol. 2003;37(5):1048–1051. doi: 10.1021/es0260115
- Bai Y, Cheng Z-Y, Bharti V, et al. High-dielectric-constant ceramic-powder polymer composites. Appl Phys Lett. 2000;76(25):3804–3806. doi: 10.1063/1.126787
- Hwang HJ, Niihara K. Perovskite-type BaTiO3 ceramics containing particulate SiC: part II microstructure and mechanical properties. J Mater Sci. 1998;33(2):549–558. doi: 10.1023/A:1004365006839
- Emoto H, Hojo J. Sintering and dielectric properties of BaTiO3-Ni composite ceramics. J Ceram Soc Jpn. 1992;100(4):555–559. doi: 10.2109/jcersj.100.555
- Pecharromán C, Esteban-Betegón F, Bartolomé JF, et al. New percolative BaTiO3-Ni composites with a high and frequency-independent dielectric constant (εr ≈ 80000. Adv Mater. 2001;13(20):1541–1544. doi: 10.1002/1521-4095(200110)13:20<1541:AID-ADMA1541>3.0.CO;2-X
- Vasilaki E, Georgaki I, Vernardou D, et al. Ag-loaded TiO2/reduced graphene oxide nanocomposites for enhanced visible-light photocatalytic activity. Appl Surf Sci. 2015;353(30):865–872. doi: 10.1016/j.apsusc.2015.07.056
- Jianjun L, Xinping L, Shengli Z, et al. Preparation and photocatalytic activity of silver and TiO2 nanoparticles/montmorillonite composites. Appl Clay Sci. 2007;37(3–4):275–280. doi: 10.1016/j.clay.2007.01.008
- Zhang D, Sun Y, Jiang C, et al. Room-temperature highly sensitive CO gas sensor based on Ag-loaded zinc oxide/molybdenum disulfide ternary nanocomposite and its sensing properties. Sens Actuators B Chem. 2017;253:1120–1128. doi: 10.1016/j.snb.2017.07.173
- Zhao Y, Tao C, Xiao G, et al. Controlled synthesis and photocatalysis of sea urchin-like Fe3O4@TiO2@Ag nanocomposites. Nanoscale. 2016;8(9):5313–5326. doi: 10.1039/C5NR08624H
- Suslick KS. Sonochemistry. Science. 1990;247(4949):1439–1445. doi: 10.1126/science.247.4949.1439
- Bang JH, Suslick KS. Applications of ultrasound to the synthesis of nanostructured Materials. Adv Mater. 2010;22(10):1039–1059. doi: 10.1002/adma.200904093
- Hangxun X, Zeiger BW, Suslick KS. Sonochemical synthesis of nanomaterials. Chem Soc Rev. 2013;42(7):2555–2567. doi: 10.1039/C2CS35282F
- He C, Liu L, Fang Z, et al. Formation and characterization of silver nanoparticles in aqueous solution via ultrasonic irradiation. Ultrason Sonochem. 2014;21(2):542–548. doi: 10.1016/j.ultsonch.2013.09.003
- Abbas M, Takahashi M, Kim C. Facile sonochemical synthesis of high-moment magnetite (Fe3O4) nanocube. J Nanopart Res. 2013;15(1):1354–1365. doi: 10.1007/s11051-012-1354-y
- Alammar T, Hamm I, Wark M, et al. Low-temperature route to metal titanate perovskite nanoparticles for photocatalytic applications. Appl Catal, B. 2015;178:20–28. doi: 10.1016/j.apcatb.2014.11.010
- Zhang Y-P, Lee S-H, Reddy KR, et al. Synthesis and characterization of core-shell SiO2 nanoparticles/poly(3-aminophenylboronic acid) composites. J Appl Polym Sci. 2007;104(4):2743–2750. doi: 10.1002/app.25938
- Gao T, Li Q, Wang T. Sonochemical synthesis, optical properties, and electrical properties of core/Shell-type ZnO nanorod/CdS nanoparticle composites. Chem Mater. 2005;17(4):887–892. doi: 10.1021/cm0485456
- Karkeh-Abadi F, Ghiyasiyan-Arani M, Salavati-Niasari M. Sonochemical synthesized BaMoO4/ZnO nanocomposites as electrode materials: A comparative study on GO and GQD employed in hydrogen storage. Ultrason Sonochem. 2022;90:106107. doi: 10.1016/j.ultsonch.2022.106167
- Hayashi Y, Takizawa H, Inoue M, et al. Ecodesigns and applications for noble metal nanoparticles by ultrasound process. IEEE Trans Packaging Manuf. 2005;28(4):338–343. doi: 10.1109/TEPM.2005.858452
- Wang C, Fang QF, Zhu ZG. Enhanced dielectric properties of low-temperature sintered SrBi2Nb2O9/Ag composites. Appl Phys Lett. 2002;80(19):3578–3580. doi: 10.1063/1.1477616
- Yamada T, Hayashi Y, Takizawa H. Synthesis of carbon nanotube/silver nanocomposites by ultrasonication. Mater Trans. 2010;51(10):1769–1772. doi: 10.2320/matertrans.MJ201012
- Nakano M, Fujiwara T, Koga N. Thermal decomposition of silver acetate: Physico-geometrical kinetic features and formation of silver nanoparticles. J Phys Chem C. 2016;120(16):8841–8854. doi: 10.1021/acs.jpcc.6b02377
- Yang YF, Luo SD, Qian M. The effect of lanthanum boride on the sintering, sintered microstructure and mechanical properties of titanium and titanium alloys. Mater Sci Eng A. 2014;618(17):447–455. doi: 10.1016/j.msea.2014.08.080
- Wang C-J, Huang C-Y, Wu Y-C. Two-step sintering of fine alumina–zirconia ceramics. Ceram Int. 2009;35(4):1467–1472. doi: 10.1016/j.ceramint.2008.08.001
- Zheng H, Zhao L, Ma Z, et al. Synergic and competitive effect of A-site substitution on structure and electric property in BaTiO3-based ceramics. J Alloys Compd. 2023;937(15):168352. doi: 10.1016/j.jallcom.2022.168352
- Hoshina T, Takizawa K, Li J, et al. Domain size effect on dielectric properties of barium titanate ceramics. Jpn J Appl Phys. 2008;47(9):7607–7611. doi: 10.1143/JJAP.47.7607
- Yasui K, Hamamoto K. Simple physical model with empirical formulas for solid-state sintering of CaCO3 for estimation of porosity. AIP Adv. 2023;13(4):045222. doi: 10.1063/5.0141905
- German RM, Suri P, Park SJ. Review: liquid phase sintering. J Mater Sci. 2009;44(1):1–39. doi: 10.1007/s10853-008-3008-0
- Yasui K, Hamamoto K. Comparison between cold sintering and dry pressing of CaCO3 at room temperature by numerical simulations. AIP Adv. 2022;12(4):045304. doi: 10.1063/5.0087226
- Samara GA. Pressure and temperature dependences of the dielectric properties of the perovskites BaTiO3 and SrTiO3. Phys Rev. 1966;151(2):378–386. doi: 10.1103/PhysRev.151.378
- Zhu W, Wang CC, Akbar SA, et al. Fast-sintering of hydrothermally synthesized BaTiO3 powders and their dielectric properties. J Mater Sci. 1997;32(16):4303–4307. doi: 10.1023/A:1018663621241