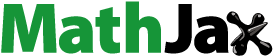
ABSTRACT
The co-precipitation method is used in the lab to create a sample of the ferromagnetic 45CaO-30P2O5-15SiO2-10Fe2O3 (mol%). In simulated body fluid (SBF), the ability of the ferromagnetic sample to regenerate bone was examined, and XRD and FTIR graphs demonstrate that bone formation has taken place. The Vibration Sample Magnetometer has confirmed that the sample’s ferromagnetic behavior was brought on by the presence of iron (VSM). When an external magnetic field is present, the resulting sample may be utilized to treat bone cancer due to its ferromagnetic nature (hyperthermia). The sample’s drug release is therefore tested both with and without the help of an external magnetic field, and the findings reveal that when the magnetic field is present, the sample displays good drug release. BET analysis also supports the capacity of drugs to load and release. The sample’s ability to distribute drugs is tested using the antibiotic gentamicin. The prolonged release of the medication in SBF solution is investigated using a first-order kinetic equation. Being a drug-loaded ferromagnetic sample with high magnetic attraction, makes it simple to target any nearby spot. With a cytotoxicity rate of more than 90%, the produced sample is seen to interact amicably with the MG-63 human cell line. According to antimicrobial research, samples also guard against both gram-positive and gram-negative germs in the implant region. By lowering the cell’s oxidative stress, the sample’s antioxidant activity aids in cell proliferation, which increases the implanted site’s recuperation.
1. Introduction
When Professor Larry Hench found glasses that could create interfacial contact with bone in 1970, he heralded the dawn of a new era in the study of biomaterials. The main concept behind Professor Hench’s development of the glasses was to create a material that would, rather than generating an interfacial layer of tissue, make a strong and live link with the host tissues when implanted (Hench 2006). It was a simple but brilliant notion to create a material with the aforementioned properties: since hydroxyapatite (HA) is the mineral makeup of bone, the implant material should necessitate generating the HA mineral on the hosted site. In light of this circumstance, several tests were conducted, and ultimately Hench and colleagues created a 45S5 bioglass® composition that effectively formed the bone-like structure on the implanted location. Glass composition needs to be chosen in the context of a particular application and is wholly dependant on a deep understanding of the effect of all primary components. Several writers then attempt to enhance the different qualities of Bioglass® by including other elements such as Zn, Mg, Cu, Mn, etc [Citation1–3]. Even if certain novel compositions need to be developed with some cutting-edge qualities like targeted drug delivery, antibacterial, and effective bone regeneration, Bioglass® and many other bioactive materials utilized for bone regeneration are commercially accessible on the market. Infection is the most significant of the several variables that affect how quickly bones regenerate. Implanted material may be rejected as a result of infection. When dealing with this issue, materials that have been loaded with antibiotics are utilized, and the drugs are released at the implanted location, but the sustained release of the drugs is a crucial issue to deal with as sometimes a high drug release might harm the host tissue. Ferromagnetic bioactive materials are frequently employed as catalysts, imaging components in Ferromagnetic Resonance imaging systems, and notably in drug administration because of their diverse features. The two main problems that must be solved for the continuous release of medications to further contribute to a patient’s speedy recovery are control release and targeted drug delivery. The risk of overdose is also reduced by the drug’s regulated or sustained release. Nanoparticles may be utilized to modify the characteristics of medication release since they have a high zeta potential and can store the drug for a longer period [Citation3,Citation4]. Ferromagnetic materials that can be targeted magnetically have recently been put to the test as targeted medicine delivery systems. The potential of the generated sample to activate Fenton’s reaction and generate reactive oxygen species (ROS) makes it beneficial in cancer therapeutic techniques, according to Farad Kerman et al. who doped iron in 45S5-derived glasses [Citation5]. The impact of iron in the presence of copper was also studied by Yaya Li et al. They discovered that iron is beneficial for the osteogenesis process and also increases the bioactivity of the sample [Citation3,Citation6]. The impact of iron doping on the sintering and mechanical behavior of a silica-based bioactive glass system was examined by Alizadeh et al. in 2004 [Citation7]. The function of iron particles as nucleation agents has been discussed by the authors [Citation8]. They have shown that a glass network containing 5.0 weight percent Fe2O3 can result in sinterable glass ceramic that has a high density and reasonably good mechanical strength [Citation8]. Mehmet Saatc et. al. 2022 [Citation9] investigate the γ-Fe2O3 effect in bioactive glass-ceramics and found that they have high bioactivity, with up to 5 wt% maghemite content. Osteosarcoma and pre-osteoblast cells are not cytotoxic to prepared bioactive glass composites at low concentrations. As the iron level rises by 5 weight percent, sample toxicity rises correspondingly. While a high concentration of magnetic materials can be harmful, a low amount of ferromagnetic material reduces the magnetic effect during hyperthermia. We require a composition with significant hyperthermia and low toxicity in view of this condition.
By creating ferromagnetic biomaterials, the author of this work hopes to achieve prolonged medication release, low toxicity, and antibacterial characteristics. An external magnetic field that is employed for targeted medicine administration draws the sample in. SBF solution is used to test the sample’s in vitro bioactivity. At two distinct pH levels, the sample’s rate of deterioration is also examined (7.4 and 3). To determine the rate at which hemoglobin aggregates while a sample is present, hemolysis research was conducted.
2. Materials and methods
2.1. Sample preparation
45CaO-30P2O5–15SiO2–10Fe2O3 (mol %) The coprecipitation approach has been used in the lab to create ferromagnetic bioactive materials. As sources for SiO2, P2O5, CaO, and Fe2O3 respectively, tetraethyl orthosilicate (TEOS), triethyl phosphate (TEP), calcium nitrate tetrahydrate (CNT), and iron nitrate nona hydrate have all been employed. A solution of IM NaOH is utilized as a precipitating agent. The reagents are purchased as analytical-grade substances (Sigma-Aldrich, USA). In the presence of ethanol and water, TEOS is first hydrolyzed. After a 15-min gap between each addition, a stoichiometric amount of TEP, calcium nitrate tetrahydrate, and iron nitrate nonahydrate are added. IM Drop by drop, NaOH is added to the solution until the pH is between 12 and 13. Whatman Filter paper No. 1 is used to filter precipitates. Precipitate is washed out several times until the pH reduces to 7. To allow for the complete evaporation of nitrates and other contaminants, precipitates are dried in an oven at 90°C for 3 hours, followed by 3 hours at 300°C and 3 hours at 700°C.
3. In vitro bioactivity
3.1. XRD
The development of the hydroxyapatite phase in the sample is evidence of its capacity to regenerate bone (HAP). X-Ray diffraction is investigated to examine the HAP growth. shows the analysis and outcomes of the XRD graph of the bioactive sample before and after in vitro testing. The typical JCPDF results are closely matched with the calcium iron phosphate phase before in vitro examination (JCPDS no. 49–1223). Following the second and seventh days, research is also done to support the HAP phase diffraction patterns. The calcium iron phosphate phase’s peaks have been seen to dissolve after 2 days due to the exchange of ions at the interface between the sample and SBF solution, and practically all of the calcium iron phosphate phase’s peaks have vanished after 7 days, leading to the growth of the HAP phase on the surface of a sample.
3.2. FTIR
FTIR is a method for verifying the sample’s bioactivity. The Perkin Elmer spectrometer, located in Germany, analyses the material using FTIR. This is done by doing FTIR on the material in transmission mode with a 400–2000 cm−1 range. The graphs of FTIR data before and after the in vitro examination are shown in . The following conclusions are drawn from the graphs:
3.2.1. Before In vitro study
Before in vitro analysis, the presence of SiO2 phases was established by the presence of Si-O rocking at 465 cm−1. In addition to these vibrations, silica was also present at 1040 cm−1 due to the presence of a two-dimensional silica network and at 872 cm −1 due to the presence of four non-bridging oxygen in SiO44-. The existence of PO4 vibrations on peaks at 558 and 601 cm−1 confirms the presence of phosphorus, another chemical ingredient. The HAP layer forms on the sample’s surface as a result of an increase in the (PO4 vibration. At 1040 cm−1, some phosphorous vibrations have been seen. Peaks at 1380, 1440 (3CO32- vibration), 872 (assume.CO32-), around 1805 (+ sym. CO3), and at 1090 (sym. CO3 sym. vibration) further support the existence of calcite. Hydroxyl (OH) molecule vibrations are verified at 1640 and 3640 cm−1. Vibration peaks at 2920–2940 and 2860 cm−1 provide proof that iron (Fe) is present.
3.2.2. After in vitro analysis
Samples were taken following an in vitro analysis at 2, and 7 days. Most peak locations remained constant; however, peak intensities varied as a result of phase deterioration in the presence of SBF. The graph shows that the peaks at 558 and 601 cm−1 (caused by (PO4 vibration)) become increasingly noticeable when the HAP layer forms. The production of a silica gel layer, which facilitates the formation of the HAp layer, is the cause of the existence of SiO2 content.
3.3. SEM-EDX
The development of new phases may be verified by changes in the sample’s morphology, and the composition can be verified using EDX. SEM pictures are collected on the second and seventh days of in vitro analysis since it has been established through XRD data that the HAP phase begins to expand after 2 days. confirms that the morphology has changed. By the second day, it was seen that the calcium and phosphate content began to rise, which suggests that the apatite layer (calcium phosphate) has begun to form on the samples’ surfaces. The development of the layer has been seen to accelerate after 7 days, and the sample’s nearly entire surface is covered by an apatite layer. The sample’s EDX is also performed to validate the compositional change (particularly of calcium and phosphorus), and it is discovered that the concentration of phosphorus and calcium increases with time. This happens as a result of the sample’s surface developing a coating of hydroxyapatite. Due to the durability of silicon and the heavy metal iron at pH 7.4, it is also noted that the dissolution of iron and silicon occurs extremely slowly. An essential quality of the sample that promotes the creation of a strong scaffold is its delayed disintegration.
t3.4. Vibrating Sample Magnetometer
Magnetic materials, such as soft ferromagnetic materials, are frequently employed in the medical industry for a variety of medicinal applications, including image sensing, cancer cell therapy, and drug delivery [Citation10,Citation11]. The sample has been uniformly magnetized using a sinusoidal vibrator field at room temperature. The detected hysteresis loop is shown in . A narrow hysteresis loop has a low saturation magnetization value and a high coercive force value, which may be deduced from the loop. Hysteric loops depicted in figure supported the prepared sample’s behavior as a soft ferromagnetic material. These top soft ferromagnetic materials may be used in a variety of medical settings, including the administration of medications and the treatment of hyperthermia [Citation12]. The interaction of iron with other elements, such as calcium and phosphorus, to generate the calcium iron phosphate phase may be the cause of the samples’ low saturation magnetization values (as inferred from the XRD study).
3.5. Drug Delivery
The drug release study of the produced sample is examined under both the influence of a natural magnetic field and an external magnetic field to test the drug carrier capacity of magnetic particles. Under typical circumstances, drug release occurs as described elsewhere [Citation3]. The results of a quick experiment are shown below. The drug release analysis of the samples has been conducted using gentamicin, an antibiotic. One gram of the sample was submerged in 20 ml of the drug (gentamicin) solution for 24 hours to analyze the drug release rate. The sample was then filtered and dried at 400 C (2° per minute rate) for the remaining 24 hours. One gram of the material was dissolved in 20 ml of SBF and incubated at 37° Celsius in an incubator to see if the loaded medicine was being released from it. The release has been investigated using ultra-visible analysis. Two milliliters of the SBF solution was removed from the analysis after the predetermined period, and the same quantity of new SBF solution was added each time. The sample, which has been soaked in SBF, is exposed to a magnetic field of 30 G for 10 minutes before sampling to see how the medication releases under the effect of the magnetic field. The pattern of drug relapse is provided. According to the observed findings in , a fast release of the loaded drug was seen during the first hour of the inviter study, and afterward, the rate of drug release in the SBF decreased (shown in ), although the drug release rate of magnetic material-doped samples rose by roughly 8% to 12% when the sample was exposed to a magnetic field. The sample’s rapid rate of drug release may be a result of the magnetic field’s heating effect on the vibrating magnetic particles. This study demonstrates that prepared samples may be employed as drug carriers since they respond well to drug delivery phenomena.
The first-order kinetic equation has been fitted to account for the amount of released gentamycin (). This model may be used to explain how medications are absorbed and eliminated from the body. To understand how gentamycin was released from our samples, the following equation was applied.
Table 1. Calculated values of R2 and K1 using the first principle.
where Qt = cumulative concentration is released in aqueous solution at time t
Q0 i = the initial amount of drug, t is the time in hrs
K1 = the release rate constant
To find the release profile, logQt is plotted against t, and the correlation coefficient (R2) has been evaluated as reported by [Citation13].
A ferromagnetic drug-loaded sample may also be employed in hyperthermia for the treatment of malignant cells by elevating the environment’s temperature around the affected body area to a range between 42°C and 45°C, according to the literature [Citation14–16]. Drugs of various sorts, such as anticancer or antibiotics, which can eradicate malignant cells or recover infected tissues nearby, may be added. A bioactive substance that not only destroys malignant bone cells but also promotes the synthesis of HAp at the site of infection of the bone is necessary for the treatment of cancerous bone cells. We can include some experimental research on the rising temperature caused by hyperthermia in our next studies.
3.6. BET
While a porous sample has a larger surface area than a nonporous sample, the surface area plays a significant role in enhancing the bioactivity of the material. Also, it is simple to put the medicine into the Porous sample. A BET analysis has been conducted to determine the prepared sample’s surface area and porous nature (). To get the required outcomes, N2 adsorption-desorption events have been investigated. All adsorption data points in the range of 0.01 to 1.0 in relative pressure (P/Po) are utilized to compute the BET surface area, whereas Barrett–Joyner–Halenda (BJH) is employed to determine the sample’s pore size. displays the results of the amount of gas absorbed by the sample, and provides details regarding the sample’s pore size. Data collected from the sample when it was prepared in the lab is compared with data collected after the medication was loaded and released for the BET test. It is discovered that when the drug release events occur in the presence of an external magnetic field, the sample’s pore volume is determined to be larger than it would be in the absence of a magnetic field. This impact of an external magnetic field is also explored. It results from the drug’s continuous and efficient release from porous materials when the magnetic field is present. It results from the drug’s continuous and efficient release from porous materials when the magnetic field is present. The existence of the Fe 2+-gentamicin link causes the sample to release drugs continuously in the presence of an external magnetic field. According to the representative figur, one of the two-electron donors in gentamicin is the 3-NH2 group, and the other is glycosidic oxygen coupled to the 2-deoxystreptamine and purpurosamine rings (a). According to the aforementioned findings, iron molecules not only produce heat during the hyperthermic therapy of malignant bone, but they also improve the sustained release of loaded medications.
4. Biological studies
4.1. Antimicrobial
The capacity of numerous microbes to withstand drugs is currently a key subject of concern [Citation17]. The only way to deal with this issue is through the creation of new antimicrobial agents to combat resistant microbes. The authors investigated the antibacterial potential of the produced ferromagnetic samples while keeping the resistance issue in mind. presents antimicrobial outcomes. Reference strains of the following bacteria were obtained from the Microbial Type Culture Collection (MTCC), Institute of Microbial Technology (IMTECH), Chandigarh, India: Pseudomonas aeruginosa (MTCC-741), Klebsiella pneumonia sub sp. pneumonia (MTCC-109), Staphylococcus aureus (MTCC-740), Salmonella typhimurium (MTCC-1251), and yeast strains such as Candida albicans. As described in our earlier study, inoculum preparation and antibacterial activity by agar well diffusion assay were carried out. The plates were incubated for 24 hours at 37°C, and the resulting inhibitory zone’s width was measured [Citation18]. Each extract and bacterial strain combination has been subjected to three separate experiments.
The generated sample has an inhibition zone between 18 and 22 mm and is effective against all of the tested pathogens, with S. aureus being the most susceptible (). The values of the inhibition zones for several gram-positive and gram-negative bacteria are shown in .
Table 2. Inhibition zones of different microorganisms.
4.2. Cytotoxicity
Cytotoxicity results of the prepared sample are given in . Oxidative stress is investigated with the help of a cytoprotective assay test [Citation19,Citation20]. Hydrogen peroxide is used to carry out assay tests and investigate the oxidative stress in human MG-63 cell lines. The dose of H2O2 is calculated by treatment of cells at 50% confluence concerning H2O2 (7.5-1000 mM dilution in medium) for 12 hours in serum-free medium. Both a positive and a negative control of the sample are investigated. The well without sample and H2O2 were considered as a negative control, whereas cells with H2O2 along with the glass sample. After that, wells are filled with 500 μl of cell concentration with 2 × 104 cells/ml. Then samples are incubated at 37°C with 5% CO2 in the air and 90% humidity for 92 hours. H2O2 dose is given (except negative control) after 12 hours to provide oxidative stress on the cell lines. Four hours before the completion of the culture MTT (2 mg/ml) is added to each well. After 4 hours, 500 ml of DMSO was added to each well. The blue color formazan is studied under UV–vis light at the wavelength of 570 nm.
5. Statistical Calculations
Data for drug delivery, antimicrobial studies, and cell culture studies is the average of three times replication of the experiment. Error bars have been used in the corresponding figures with average ±SD.
6. Conclusion
From all the above discussion we can conclude as follows:
Nano bioactive magnetic material is confirmed by SEM study. The formation of HAp confirmed the bioactive nature along with the controlled degradation of samples in the SBF solution. A degradation study with a citric buffer also acknowledges the controlled degradation rate of the sample. Being an antimicrobial agent in the presence of heavy metal iron for a long time during in vitro analysis is good for the protection against gram-positive and gram-negative microorganisms. The VSM data show the ferromagnetic (soft) behavior of the prepared samples, which is a good indication for the use of these soft magnetic bioactive materials for clinical applications. Drug delivery tendency of magnetic particles has been from 8% to 12% under the influence of an external magnetic field. The prepared ferromagnetic sample is nontoxic and provides a healthy environment for the growth of MG 63 osteoblast cells. The cell viability of the sample is high (~91%) as compared to the negative control. The prepared sample is also good for the recovery of cells from oxidative stress and acts as a good cytoprotective material. Antimicrobial testing of samples confirms the strong tendency of samples to kill gram-positive and gram-negative microorganisms. From all these results, we can conclude that our prepared ferromagnetic nano bioactive sample is good bioactive with improved degradation and antimicrobial properties, this sample may also be used as a good drug carrier for any antibiotic or anticancer drug which enhances the rate of drug release when kept under the influence of magnetic field for hyperthermia treatment.
Declaration of conflicting interests
The Authors declare that there is no conflict of interest.
Disclosure statement
No potential conflict of interest was reported by the author(s).
References
- Kaur K, Singh KJ, Anand V, et al. Elucidating the role of size of hydroxyl apatite particles toward the development of competent antiosteoporotic bioceramic materials: in vitro and in vivo studies. J Biomed Mater Res. 2019;107(8):1723–1735. doi: 10.1002/jbm.a.36687
- Kaur K, Singh KJ, Anand V, et al. Lanthanide (=Ce, Pr, Nd, and Tb) ions substitution at calcium sites of hydroxyl apatite nanoparticles as fluorescent bio probes: Experimental and density functional theory study. Ceram Int. 2017;43(13):10097–10108. doi: 10.1016/j.ceramint.2017.05.029
- Anand V, Singh K, Kaur K, et al. Comparative study of Silver nanoparticles Coated and Uncoated NiO-Fe 2 O 3 -CaO-SiO 2 -P 2 O 5 ferromagnetic bioactive ceramics. J Am Ceram Soc. 2016;99(11):3632–3638. doi: 10.1111/jace.14389
- Park JH, Saravanakumar G, Kim K, et al. Targeted delivery of low molecular drugs using chitosan and its derivatives. Adv Drug Delivery Rev. 2010;62(1):28–41. doi: 10.1016/j.addr.2009.10.003
- Kermani F, Vojdani-Saghir A, Mollazadeh Beidokhti S, et al. Iron (Fe)-doped mesoporous 45S5 bioactive glasses: Implications for cancer therapy. Transl Oncol. 2022;20:30–41. doi: 10.1016/j.tranon.2022.101397
- Li Y, Ramesh V, Bider F, et al. Co-doping of iron and copper ions in nanosized bioactive glass by reactive laser fragmentation in liquids. J Biomed Mater Res Part A. 2022;110(9):1537–1550. doi: 10.1002/jbm.a.37393
- Alizadeh P, Yekta BE, Gervei A. Effect of Fe2O3 addition on the sinterability and machinability of glass-ceramics in the system MgO–CaO–SiO2–P2O5. J Eur Ceram Soc. 2004;24(13):3529–3533. doi: 10.1016/j.jeurceramsoc.2003.11.028
- Cannas M, Indemini E, Krajewski A, et al. In vitro observations of iron-doped bioactive glasses. Biomaterials. 1990;11(4):281–285. doi: 10.1016/0142-9612(90)90011-E
- Baino F, Fiume E, Miola M, et al. Fe-doped bioactive glass-derived scaffolds produced by sol-gel foaming. Mater Lett. 2019;235:207–211. doi: 10.1016/j.matlet.2018.10.042
- Li G, Feng S, Zhou D. Magnetic bioactive glass ceramic in the system CaO–P2O5–SiO2–MgO–CaF2–MnO2–Fe2O3 for hyperthermia treatment of bone tumor. J Mater Sci. 2011;22(10):2197–2208. doi: 10.1007/s10856-011-4417-1
- Jayalekshmi AC, Victor SP, Sharma CP. Magnetic and degradable polymer/bioactive glass composite nanoparticles for biomedical applications. Colloids Surf B Biointerfaces. 2013;101:196–204. doi: 10.1016/j.colsurfb.2012.06.027
- Wang T-W, Wu H-C, Wang W-R, et al. The development of magnetic degradable DP-Bioglass for hyperthermia cancer therapy. J Biomed Mater Res Part A. 2007;83A(3):828–837. doi: 10.1002/jbm.a.31411
- Anand V, Singh KJ, Kaur K, et al. B 2 O 3 –MgO–SiO 2 –Na 2 O–CaO–P 2 O 5 –ZnO bioactive system for bone regeneration applications. Ceram Int. 2016;42(2):3638–3651. doi: 10.1016/j.ceramint.2015.11.029
- Goel A, Kapoor S, Tilocca A, et al. Structural role of zinc in biodegradation of alkali-free bioactive glasses. J Mat Chem B. 2013;1(24):3073–3082. doi: 10.1039/c3tb20163e
- Goel A, Kapoor S, Rajagopal RR, et al. Alkali-free bioactive glasses for bone tissue engineering: a preliminary investigation. Acta Biomater. 2012;8(1):361–372. doi: 10.1016/j.actbio.2011.08.026
- Lesniak W, Pecoraro VL, Schacht J. Ternary complexes of gentamicin with iron and lipid catalyze the formation of reactive oxygen species. Chem Res Toxicol. 2005;18(2):357–364. doi: 10.1021/tx0496946
- Gould IM. Antibiotic resistance: the perfect storm. Int J Antimicrob Agents. 2009;34(Supplement 3):S2–S5. doi: 10.1016/S0924-8579(09)70549-7
- Bauer Aw Fau - Kirby WM, Kirby Wm Fau - Sherris JC, Sherris Jc Fau - Turck M, et al. Antibiotic susceptibility testing by a standardized single disk method. Am J Clin Pathol. 1966;45(4_ts):493–496. doi: 10.1093/ajcp/45.4_ts.493
- Li M, Zhao L, Liu J, et al. Hydrogen peroxide induces G2 cell cycle arrest and inhibits cell proliferation in osteoblasts. Anat Rec. 2009;292(8):1107–1113. doi: 10.1002/ar.20925
- Clover J, Gowen M. Are MG-63 and HOS TE85 human osteosarcoma cell lines representative models of the osteoblastic phenotype? Bone. 1994;15(6):585–591. doi: 10.1016/8756-3282(94)90305-0