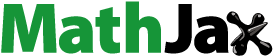
ABSTRACT
This study developed magnetically separable CeO2/CoFe2O4 heterojunction photocatalysts for dye degradation to reduce water pollution. The CeO2/CoFe2O4 nanocomposites with different CeO2 ratios (10, 20, and 30 wt%) were prepared by a precipitation method. The nanocrystalline 20%CeO2/CoFe2O4 photocatalyst with a surface area of 117 m2.g−1, Eg 2.37 eV, and uniform distribution of quasi-spherical CeO2 minor phase (cubic fluorite structure with particle size 3 ± 1 nm) throughout the distorted spherical CoFe2O4 major phase (cubic spinel structure with particle size 9 ± 3 nm) showed the best photocatalytic performance for methylene blue (MB) degradation under UV light irradiation at the efficiency of 88.7% and the apparent rate constant (k) of 0.0138 min−1. The 20%CeO2/CoFe2O4 photocatalyst had a good magnetic response and could be easily separated from the dye solution by applying an external magnetic field. The reusability test showed lower photodegradation efficiency due to the adsorption of residual MB dye on the photocatalyst surface. The formation of heterojunction at the interfaces of CeO2/CoFe2O4 facilitated the photogenerated charge separation and increased availability of active species of holes (h+) and hydroxy radicals (∙OH), which were the key factors in improving the photocatalytic activity.
HIGHLIGHTS
Improved photocatalysis in CeO2-coupled CoFe2O4 composites.
Heterojunctions in CeO2/CoFe2O4 increase the availability of active species.
External magnetic field separates the photocatalyst from the dye solution.
1. Introduction
Water pollution associated with the growth of industrialization and urbanization can cause several adverse effects on ecosystems and human health [Citation1,Citation2]. The use of synthetic dyes in various industries, including the textile, printing, tannery, paint, paper, pulp, cosmetic, food, pharmaceutical, and photoelectrochemical industries, is one of the main causes of water pollution as the dyeing process consumes and discharges a large amount of water and dye effluent [Citation2–4]. Many treatment processes and technologies have been used to remove synthetic dyes from industrial wastewater to reduce their environmental impact [Citation2]. Semiconductor photocatalysis is a green and cost-effective technology [Citation5] that can be used to eliminate organic synthetic dyes from wastewater by photo-inducing charge generation of electron and hole pairs (e−/h+) at semiconductor surfaces to produce reactive oxygen species (ROS) through oxidation and reduction reactions. Subsequently, these reactive species react with the dye molecules and decompose them into less toxic products [Citation1,Citation4,Citation6,Citation7].
Heterogeneous photocatalysts, e.g. TiO2, ZnO, CdSe, WO3, Bi2WO6, BiVO4, and Fe2O3, have been used to treat organic dye wastewater [Citation4,Citation8–14]. Magnetic nanosized spinel ferrite photocatalysts, e.g. CoFe2O4, NiFe2O4, CuFe2O4, ZnFe2O4 and SnFe2O4 [Citation1,Citation15–18], have recently received great attention as they can overcome a technical problem in separating and recovering the photocatalyst from a dye solution after the photodegradation reaction that limits the practical application and may cause secondary pollution to the environment [Citation1,Citation15,Citation16]. The suspended magnetic photocatalyst particles can simply be separated by applying an external magnetic field [Citation1,Citation19]. CoFe2O4, an n-type semiconductor with a cubic inverse spinel structure, is a ferrimagnetic material with high coercivity, moderate saturation magnetization, high absorption capability, high physical and chemical stability, and non-toxicity [Citation1,Citation20–22]. Even though CoFe2O4 photocatalysts possess a high potential to be used as magnetically separable photocatalysts, their photocatalytic activity is relatively low due to a narrow band gap energy. The low Eg of CoFe2O4 at 1.1–2.3 eV [Citation1,Citation19,Citation22,Citation23] causes a fast electron-hole pair recombination rate and poor charge separation performance [Citation1,Citation24]. Coupling CoFe2O4 with other dissimilar band structure semiconductors to form heterojunction photocatalysts is an effective approach to improve the photocatalytic efficiency since the formation of heterojunction interface can prolong the photo-induced electron–hole pair recombination, which interns increasing the charge participation in the photocatalytic reactions [Citation1,Citation9,Citation25–27].
Heterojunction systems of TiO2/CoFe2O4 [Citation28–30], ZnO/CoFe2O4 [Citation31], AgBr/CoFe2O4 [Citation32], BiVO4/CoFe2O4 [Citation33], Ni/CoFe2O4 [Citation34], graphene/CoFe2O4 [Citation35], rGO/CoFe2O4 [Citation36], Ag3PO4/CoFe2O4 [Citation19,Citation37], g-C3N4/CoFe2O4 [Citation38], and polyaniline/CoFe2O4 [Citation39] composite photocatalysts were reported to increase the degradation efficiency of organic dyes, e.g. methylene blue (MB), methyl orange (MO), rhodamine B (RhB), reactive red 120 (RR-120) crystal violet (CV), acridine orange (AO), rhodamine 6 G (R-6 G), active black (BL-G), and active red (RGB), as well as showing a good magnetic response. CeO2, an n-type semiconductor with an Eg of 2.3–3.2 eV [Citation40–43], is an interesting choice for coupling with CoFe2O4 [Citation44,Citation45] since it has desirable photocatalytic properties, including high UV absorption, physiochemical stability, and large oxygen storage and release capacity [Citation46,Citation47]. Moreover, CeO2 has suitable band edge positions to form heterojunction at the interface of CoFe2O4, which can increase the charge separation, so coupling CeO2 with CoFe2O4 would benefit to optimize the photocatalytic activity of CoFe2O4. The synthesis of CoFe2O4/CeO2 nanocomposites was studied for possible application in photocatalysis [Citation44], and core-shell CoFe2O4/CeO2 photocatalyst was reported to effectively activate sodium persulfate (Na2S2O8) for orange II dye degradation [Citation45]. However, the nanocomposite system of minor phase CeO2 with CoFe2O4 heterojunction photocatalyst for direct dye degradation has not been studied in detail yet.
In this study, magnetically separable CeO2/CoFe2O4 heterojunction photocatalysts were prepared and characterized. The photocatalytic efficiency and rate constant for dye degradation were examined, and the possible mechanism for the photocatalytic degradation over the CeO2/CoFe2O4 system was also proposed.
2. Material and methods
2.1. Photocatalyst preparation
CoFe2O4 nanoparticles were prepared by the sono-assisted-precipitation method [Citation21]. Firstly, 0.1 mol of cobalt(II) nitrate hexahydrate (Co(NO3).6 H2O; 99% purity, Carlo Erba) and 0.2 mol of ferric(III) nitrate nonahydrate (Fe(NO3).9 H2O; 99% purity, Carlo Erba) were dissolved in 100 mL of deionized water and stirred with a magnetic bar at 80°C for 2 h. The mixed solution was added dropwise into 100 mL of 10 M sodium hydroxide solution (NaOH; 98% purity, Carlo Erba) that was simultaneously irradiated with the high-intensity ultrasonic wave at 20 kHz 150 W/cm2 (Ti-horn, Sonics model VCX 750, Vibracell). Notably, the highly basic concentration of precipitation medium at 10 NaOH was used as it could increase the nucleation rate, pH, and ionic strength, which resulted in CoFe2O4 with a small crystallite size [Citation48]. The reaction was conducted for 30 min. After that, the black precipitated were separated in a centrifuge, washed with distilled water until neutral (pH ~ 7), and dried at 90°C for 24 h to obtain CoFe2O4 nanoparticles. CeO2 nanoparticles were prepared by a precipitation method [Citation42,Citation43]. 100 mL of 0.1 M ammonium cerium(IV) nitrate solution ((NH4)2Ce(NO3)6; 99% purity, Acros Organics) was adjusted to pH 12 with 0.5 M NaOH solution, followed by magnetic stirring at room temperature for 2 h. Next, the pale-yellow precipitate was separated in a centrifuge, washed with distilled water until neutral (pH ~ 7), and dried at 90°C for 24 h. To prepare CeO2/CoFe2O4 heterojunction photocatalysts, the required amounts of CeO2 (10, 20, and 30 wt%) and 2 g of CeFe2O4 powders were dispersed in 100 mL deionized water under a high-intensity ultrasonic irradiation at 20 kHz 150 W.cm−2 for 15 min. The products were separated in a centrifuge, dried at 90°C for 24 h, and finally ground in an agate mortar to obtain 10%CeO2/CoFe2O4 and 20%CeO2/CoFe2O4 and 30%CeO2/CoFe2O4 nanocomposite photocatalysts.
2.2. Characterization
Crystal structures of photocatalysts were examined by an X-ray diffractometer (XRD; Rigaku Smartlab, CuKα, 40 kV 30 mA), using indices from the JCPDS (ICDD) database. The lattice parameter (a) was calculated from the Bragg equation, and the crystal spacing was determined by assuming a cubic symmetry. The crystallite size (D) was estimated using the Debye-Scherrer’s equation as shown in EquationEquation 1(1)
(1) [Citation49]:
where k is a constant taken as 0.94, λ is X-ray wavelength (CuKα radiation = 0.15418 nm), βhkl is the full width at half maximum (FWHM) of the intensity of the observed diffraction peak, hkl are the Miller indices of the planes being analyzed, which are (311) plane for CoFe2O4, (111) plane for CeO2 and (311) plane of CoFe2O4 phase for the CeO2/CoFe2O4 composites, and θ is the diffraction angle. Microstructures of the photocatalysts were studied by a scanning electron microscope (SEM; Hitachi SU3500) and transmission electron microscope (TEM; FEI Tecnai G2 20 Twin). Elemental compositions were examined by an energy-dispersive spectrometer (EDS; Oxford instrument X-MAX 20) equipped with SEM and energy-dispersive X-ray fluorescence spectrometer (EDXRF; Rigaku NEX CG). The average particle size and distribution were obtained from the ImageJ program by measuring at least 100 sample particles observed in TEM images. The Brunauer–Emmett–Teller (BET) specific surface area was measured by adsorption-desorption of nitrogen gas (N2) with Autosorb (Autosorb-1). The optical property was studied using a diffuse reflectance ultraviolet-visible spectrophotometer (UV-Vis DRS; Thermo Scientific evolution 201). The optical band gap energy (Eg) of semiconducting photocatalysts was calculated using the Tuac’s equation (EquationEquation 2(2)
(2) ) [Citation34,Citation50]:
When α is the absorption coefficient, h is the Plank’s constant (6.626 × 10−34 J.s), ν is the frequency of light (s−1), and n is a constant depending on the nature of band transitions, where n = 2 for the indirect transition in CoFe2O4, CeO2, and CeO2/CoFe2O4 composites [Citation51,Citation52], β is the absorption constant for an indirect transition metal, which is equal to 1. By plotting between the (αhν)2 in the y-axis versus hν in the x-axis, Eg in a unit of eV was evaluated from the intercept of the linear region with the x-axis ((αhν)2 = 0) in the plot. The magnetic property to evaluate the magnetic separability of the photocatalysts from the dye solution was tested at room temperature using a vibrating sample magnetometer (VSM, in-house Kasetsart University; Thailand) at a maximum applied field of 10,000 Oe. The molecular structure of photocatalysts before and after the photodegradation reaction was studied using a Fourier transform infrared spectrometer (FT-IR; Perkin Elmer, Spectrum Gx, potassium bromide (KBr) mixing in transmission mode).
2.3. Photocatalytic degradation of dye
The photocatalytic activity of CoFe2O4, CeO2, and CeO2/CoFe2O4 composites for degradation of methylene blue dye (MB; C16H18N3SCl.3 H2O, cationic dye, 93% purity, Carlo Erba) and Congo red dye (CR; C32H22N6Na2O6S2, anionic azo dye, 35% purity, Carlo Erba) in aqueous solution under UV light radiation was studied. 14 mg of photocatalyst powders were dispersed in 50 mL of 10 mg.L−1 (ppm) MB solution or 30 mg.L−1 CR solution by stirring with a magnetic bar, and the pH of the solution at which the reaction carried out was pH 7.3 for MB degradation and pH 7.1 for CR degradation. At the first 30 min, the photocatalyst suspension was stirred in the dark to reach adsorption-desorption equilibrium. After that, the suspension was irradiated with a UV-C mercury lamp (Tokiva, 15 W, luminous intensity 7800 cd, UV-C output 4.9 W with peak light emission intensity at a wavelength of 254 nm and other emissions at 365, 408, and 436 nm) for 240 min. The suspension was collected every 30 min and centrifugally separated to remove the suspended photocatalysts. The reusability was tested by magnetically separating the solid photocatalysts from the dye solution, washing them with distilled water, drying at 90°C for 24 h, and reusing in the same experimental procedures. The adsorption and photodegradation of dye were examined by measuring the absorbance of MB solution at λmax of 664 nm and CR solution at λmax of 493 nm using a UV-visible spectrophotometer (Thermo Scientific evolution 201). To optimize the photodegradation reaction, the effects of photocatalyst dosage and initial dye concentration were studied by varying the photocatalyst dosages at 7, 14, and 21 mg and the MB concentrations at 5, 10, 15, and 20 mg.L−1. The dye adsorption and photodegradation efficiency was calculated from EquationEquation 3(3)
(3) [Citation34,Citation50]:
When C0 is the initial concentration of the dye solution, and C is the concentration of the dye solution after the adsorption and photodegradation at a certain time. The kinetics of dye photodegradation were obtained by fitting the pseudo-first-order equation (EquationEquation 4(4)
(4) ) [Citation34,Citation50], where k is the apparent rate constant (min−1), and t is irradiation time (min). The apparent rate constant (k) was estimated from the slope of the plot between -ln (C/C0) on the y-axis versus t on the x-axis.
3. Results and discussion
3.1. Materials characterization
XRD patterns of CoFe2O4, CeO2 and CeO2/CoFe2O4 composites with different wt% of CeO2 prepared by the precipitation method without a calcination process are shown in . The XRD pattern of CoFe2O4 showed diffraction peaks at 2θ = 30.4°, 35.9°, 43.4°, 57.1° and 63.2° corresponded to the (220), (311), (400), (511), and (440) planes of CoFe2O4 with a cubic spinel structure (JCPDS No. 22–1086, space group Fd-3 m). The XRD pattern of CeO2 showed broad diffraction peaks at 2θ = 28.5°, 33.0°, 47.4°, and 56.3° corresponded to the (111), (200), (220), and (311) planes of CeO2 with a cubic fluorite structure (JCPDS No. 34–0394, space group Fm-3 m). For 10%CeO2/CoFe2O4, 20%CeO2/CoFe2O4, and 30%CeO2/CoFe2O4 composites, XRD patterns showed the diffraction peaks indexed to both major and minor phases of CoFe2O4 and CeO2 in which the intensity of CeO2 diffraction peaks increased with increasing %wt of CeO2. shows the calculated cubic lattice parameter (a) and crystallite size of CoFe2O4, CeO2, and CeO2/CoFe2O4 composites. The lattice parameters of pure CoFe2O4 (8.3235 Å) and CeO2 (5.4261 Å) were close to those reported in the JCPDS (ICDD) database (CoFe2O4, JCPDS No. 22–1086 a = 8.391Å and CeO2, JCPDS No. 34–0394 a = 5.4113Å). No significant change in the lattice parameters of the CeO2/CoFe2O4 composites (8.3213–8.3274 Å) compared to CoFe2O4, suggesting the formation of composites without cation substitution. The crystallite size of CoFe2O4 and the CeO2/CoFe2O4 composites, which were estimated from (311) plane of CoFe2O4, varied from 12.1–13.5 nm, while CeO2 ((111) plane) was 3.2 nm, which was in the range of nanocrystalline materials.
Table 1. Lattice parameter, crystallite size, and surface area of CoFe2O4, CeO2 and CeO2/CoFe2O4 composites * estimated from CoFe2O4 phase.
SEM and TEM images of pure CoFe2O4 and CeO2, with particle size distributions inset, are shown in . CoFe2O4 had distorted spherical particles, while CeO2 was non-uniform quasi-spherical particles. Particle agglomeration was observed in both CoFe2O4 and CeO2. The average particle size of CoFe2O4 (9 ± 3 nm) and CeO2 (3 ± 1 nm) agreed well with the crystallite sizes estimated by the XRD study (), confirming the formation of nanocrystalline CoFe2O4 and CeO2 [Citation53]. shows SEM images of 10%CeO2/CoFe2O4, 20%CeO2/CoFe2O4, and 30%CeO2/CoFe2O4 composites plus EDS elemental mappings and fractions (wt%). The EDS elemental mappings showed uniform distribution of the CeO2 minor phase throughout the CoFe2O4 major phase, and it is interesting to note that a higher degree of CeO2 agglomeration was observed in 30%CeO2/CoFe2O4 composite. When considering the elemental fractions, the Ce fraction in the composites increased with increasing the CeO2 content, whereas the Co and Fe fraction decreased. The EDXRF was used to determine the oxide composition of composites and the actual wt% of CeO2 in 10%CeO2/CoFe2O4, 20%CeO2/CoFe2O4 and 30%CeO2/CoFe2O4 was 7.73 wt%, 11.17 wt%, and 23.90 wt%, respectively. shows TEM and high-resolution TEM (HRTEM) images of 20%CeO2/CoFe2O4. The composite consisted of a mixed morphology of distorted spherical-shaped particles of CoFe2O4 and quasi-spherical CeO2. The lattice fringes could be seen in the high-resolution image (). The interplanar spacings (d-spacings) of 0.21 nm and 0.25 nm corresponded to the reflection of the (400) and (311) planes of CoFe2O4, while the interplanar spacing of 0.31 nm was attributed to (111) plane of CeO2. The interfacial contact between CoFe2O4 and CeO2 phases observed in the HRTEM image confirmed the formation of heterojunctions of CeO2/CoFe2O4.
The BET surface area of CoFe2O4, CeO2, and CeO2/CoFe2O4 composites are shown in . The specific surface area of CoFe2O4 (163 m2.g−1) was lower than that of CeO2 (228 m2.g−1), which corresponded well with the larger particle size of CoFe2O4 (9 ± 3 nm) than CeO2 (3 ± 1 nm). The surface area of CoFe2O4 and CeO2 obtained in this work are comparable to those previously reported for nanosized CoFe2O4 (160–285 m2.g−1 [Citation53–55]) and CeO2 (200–457 m2.g−1 [Citation56–58]). The composites, 10%CeO2/CoFe2O4 (116 m2.g−1), 20%CeO2/CoFe2O4 (117 m2.g−1) and 30%CeO2/CoFe2O4 (142 m2.g−1), had lower surface area than those of the pure CoFe2O4 and CeO2, which could be attributed to particle agglomeration due to the physical bonding between CeO2 and CoFe2O4 phases [Citation44,Citation45]. The surface area of the composites tended to increase with increasing the amount of higher surface area phase of CeO2. Notably, the surface area of 10%CeO2/CoFe2O4 and 20%CeO2/CoFe2O4 was close at 116 and 117 m2.g−1, and this was probably because, at these ratios, CeO2 was uniformly dispersed throughout the CoFe2O4 phase to create the interfacial contact between both phases.
shows the diffuse reflectance UV-visible spectra inset with Tuac’s plot for determining optical band gap energy (Eg) of CoFe2O4, CeO2, and CeO2/CoFe2O4 composites. The absorption spectra showed that pure CoFe2O4 with a black surface could absorb a broad absorption range of UV light (200–400 nm) and visible light (400–800 nm) regions, while CeO2 mainly absorbed UV light. The absorption intensity of CeO2/CoFe2O4 composites was lower than CoFe2O4 but tended to increase compared to CeO2, especially in the visible light region. The Eg values in show that the Eg of pure CoFe2O4 at 2.15 eV and CeO2 at 3.07 eV
Figure 5. Diffuse reflectance UV-visible spectra inset with Tuac’s plot for Eg determination of CoFe2O4, CeO2 and CeO2/CoFe2O4 composites.

Table 2. Eg and degradation efficiency of CoFe2O4, CeO2, and CeO2/CoFe2O4 photocatalysts.
are comparable to the values reported previously (CoFe2O4 1.1–2.3 eV [Citation1,Citation19,Citation22,Citation23] and CeO2 2.3–3.2 eV [Citation40–43]). For the CeO2/CoFe2O4 composites, coupling CoFe2O4 with a higher Eg phase, CeO2, resulted in an increase of Eg values, in which the Eg values of 10%CeO2/CoFe2O4, 20%CeO2/CoFe2O4, and 30%CeO2/CoFe2O4 were 2.19 eV, 2.37 eV, and 2.58 eV, respectively.
3.2. Photocatalytic degradation of dye
The photocatalytic activity of CoFe2O4, CeO2, and CeO2/CoFe2O4 photocatalysts was determined by methylene blue dye (MB) degradation under UV irradiation. The degradation efficiency is shown in and . The blank control of 10 mg.L−1 MB in the absence of the photocatalysts showed a stable concentration of MB under UV irradiation over the studied period at 240 min. In this study, the suspension of the 14 mg photocatalysts in 50 mL of 10 mg.L−1 MB solution at pH 7.3 was stirred with a magnetic bar in the dark for 30 min to reach the adsorption-desorption equilibrium. At 30 min before the UV irradiation, CoFe2O4 had higher adsorption efficiency than CeO2, although it possessed a lower surface area and this could be because the pH of 10 mg.L−1 MB solution at 7.3 was higher than the point of zero charge (pHpzc) of CoFe2O4 = 7.2 [Citation59] but lower than the pHpzc of CeO2 = 8.1 [Citation60], which resulted in a greater electrostatic attraction between the negatively charged CoFe2O4 surface and the cationic MB dye molecules [Citation59,Citation61]. In the case of the composites, the adsorption efficiency of 20%CeO2/CoFe2O4 was the highest due to the good dispersion of the CeO2 throughout the CoFe2O4 matrix. Increasing the CeO2 content excess 20 wt% to form 30%CeO2/CoFe2O4 led to the agglomeration of the CeO2 phase as observed in the SEM-EDS study and thus lowered the adsorption efficiency. After the UV irradiation, the MB photodegradation efficiency of all photocatalysts increased sharply with increasing the reaction times from 30 to 180 min and became steady from 180 to 240 min. According to the photodegradation efficiency shown in , CeO2 is more active for MB degradation than CoFe2O4. The composite photocatalysts, which possessed lower surface area (), showed better photocatalytic performance than pure CoFe2O4 and CeO2, and this suggested that the formation of CeO2/CoFe2O4 heterojunctions played more role in the photocatalytic activity than the surface area. The total MB degradation efficiency at the reaction time of 180 min was shown to follow the order: CoFe2O4 (34.0%) < CeO2 (52.0%) < 30%CeO2/CoFe2O4 (67.7%) < 10%CeO2/CoFe2O4 (71.1%) < 20%CeO2/CoFe2O4 (73.8%). The kinetics of MB photodegradation estimated by the plot between -ln (C/C0) versus UV irradiation time (t) for 120 min time intervals from the time at 30 to 150 min are shown in . The plots of all photocatalysts followed the pseudo-first-order reaction and the apparent rate constant (k) obtained from the slope was summarized in . Notably, the k values were estimated from the longest period data (30–150 min) that produced the slope with R2 values close to 1. The composite photocatalyst possessed a higher photodegradation rate than the pure phases in which 20%CeO2/CoFe2O4 showed the best performance with the k value of 0.0041 min−1. This suggested that the optimum ratio for improving the photocatalytic activity was 20 wt% CeO2 loading to CoFe2O4. Further increasing CeO2 loading to 30 wt% lowered the photocatalytic activity due to agglomeration of the CeO2 phase, which led to less interfacial contact between CeO2 and CoFe2O4 phases.
The photocatalytic degradation of anionic dye, Congo red (CR), under UV irradiation was also studied. The photodegradation of 50 mL of 30 mg.L−1 CR solution (pH 7.1) by using 14 mg of 20%CeO2/CoFe2O4 photocatalysts is shown in . The initial concentration of CR solution was used at 30 mg.L−1 due to the high adsorption capacity of anionic CR dye molecules on the positively charged surface of 20%CeO2/CoFe2O4 (30 mg.L−1 CR solution had pH = 7.1, which was lower than pHpzc of CoFe2O4 = 7.2 [Citation59] and CeO2 = 8.1 [Citation60]). The CR degradation efficiency at the reaction time of 240 min was 51.3%. The apparent rate constant (k) for photodegradation estimated from 210 min time intervals (30 to 240 min) was 0.0025 min−1, which was lower than the photodegradation rate of cationic MB dye at 0.0041 min−1. The reduction of the rate constant was probably related to the high adsorption capacity of anionic CR dye that prevented the photons from reaching the photocatalyst surface to activate the photocatalytic reaction [Citation32].
Figure 8. The photocatalytic degradation and kinetics plots for the pseudo-first-order reaction of 20%CeO2/CoFe2O4 in (a and b) CR, (c and d) MB at different photocatalyst dosage, and (e and f) MB at different initial concentration under UV irradiation.

The effects of photocatalyst dosage (7, 14, and 21 mg of 20%CeO2/CoFe2O4) and initial MB dye concentration (5, 10, 15, and 20 mg.L−1) at pH ~ 7.3 on the photodegradation reaction were examined, and the results are shown in . The degradation efficiency increased with increasing the photocatalyst dosage, in which the photodegradation rate constant obtained from using 7, 14, and 21 mg of 20%CeO2/CoFe2O4 photocatalysts in 50 mL of 10 mg.L−1 MB solution were 0.0016, 0.0041, and 0.0065 min−1, respectively. The increase of the rate constant with increasing the photocatalyst dosage was due to the higher number of active sites available for the photocatalytic reaction [Citation32]. However, it should be noted that the excess amount of photocatalyst dosage may decrease the photocatalytic activity due to the light scattering factor [Citation28,Citation34]. show the photocatalytic degradation of 14 mg 20%CeO2/CoFe2O4 in 50 mL of MB solution with different initial concentrations at 5, 10, 15, and 20 mg.L−1. The degradation efficiency of 5, 10, 15, and 20 mg.L−1 MB solution at 180 min was 88.7%, 73.8%, 52.7%, and 42.7%, respectively. The photodegradation rate constant (k) decreased with the increase of initial MB concentration from 5 mg.L−1 (0.0138 min−1), 10 mg.L−1 (0.0041 min−1) to 15 mg.L−1 (0.0036 min−1) and became stable at 20 mg.L−1 (0.0036 min−1). The decrease in photodegradation rate in higher MB concentrations was because the darker color of the dye solution decreased the path length of photons reaching the photocatalyst surface [Citation28,Citation32,Citation37]. Based on these results, the optimum photoreaction condition with 88.7% photodegradation efficiency and k = 0.0138 min−1 was to use 14 mg of 20%CeO2/CoFe2O4 photocatalyst in 50 mL of 5 mg.L−1 MB solution under UV irradiation for 180 min. To further enhance the photocatalytic activity of 20%CeO2/CoFe2O4 in the degradation MB may be done by varying the photoreaction condition by adjusting the pH of the dye solution to pH = 10 [Citation32] to increase the surface adsorption/desorption of cationic dye molecules or adding electron acceptor such as peroxomonosulphate species (SO52−) [Citation28] since these methods were reported to optimize the activity of CoFe2O4-based photocatalysts. summarizes the activity of CoFe2O4-based photocatalysts for dye degradation.
Table 3. Summary of CoFe2O4-based photocatalysts for dye degradation.
The mechanism for photocatalytic degradation of MB over heterojunction CeO2/CoFe2O4 photocatalyst under UV irradiation is shown in . The energy bands were used for deriving the diagram by considering the relative positions of the conduction band (CB), valance band (VB) and oxidation potentials. The band edges of the VB (EVB) and CB (ECB) of CoFe2O4 and CeO2 semiconductors were calculated from EquationEquation 5(5)
(5) and Equation6
(6)
(6) [Citation62]:
where χ is the absolute electronegativity of the semiconductors, 5.81 eV for CoFe2O4 [Citation24] and 5.56 eV for CeO2 [Citation62], Ee is the energy of free electron on the normal hydrogen scale (NHE), ~4.5 eV [Citation62]. Eg is the band gap energy of the semiconductors, 2.15 eV for CoFe2O4 and 3.07 eV for CeO2. Thus, the calculated EVB and ECB relative to the NHE of CoFe2O4 were 2.39 eV and 0.24 eV, while those of CeO2 were 2.60 eV and −0.48 eV, respectively. According to an alignment of EVB and ECB of CoFe2O4 and CeO2, the band edges of CB and VB of CeO2 are higher and lower, respectively, than those of CoFe2O4, so CeO2/CoFe2O4 system is classified into type I heterojunction photocatalyst [Citation1,Citation9]. The photodegradation of MB over the CeO2/CoFe2O4 photocatalyst started when the surface of CoFe2O4 and CeO2 semiconductors were irradiated with UV light and absorbed energy equal to or higher than their Eg to produce photogenerated electron (e−)- holes (h+) pairs from the electrons excited to the CB and holes left in the VB. In the proposed mechanisms, h+ from the CeO2 VB is transferred to the CoFe2O4 VB through the interface, as the CeO2 EVB (2.60 eV) is more positive than the CoFe2O4 EVB (2.39 eV). Meanwhile, the photoexcited e− in the CeO2 CB (−0.48 eV) migrated to the more positive CoFe2O4 CB (0.24 eV). Then, these photogenerated charges reacted with the absorbed species on the semiconductor surface, e.g. O2, H2O, and hydroxide ion (OH−), to generate reactive oxygen species (ROS) for MB degradation. When considering the standard oxidation-reduction potentials, the CoFe2O4 ECB (0.24 eV) is not negative enough to reduce O2 to superoxide radicals (∙O2−) via e− acceptation (O2/∙O2− = −0.046 eV vs. NHE [Citation24]), while the CoFe2O4 EVB (2.40 eV) is more positive to oxidize OH− into hydroxyl radical (∙OH) via h+ acceptation (OH−/∙OH = 1.99 eV vs. NHE [Citation24]). This suggested that in the CeO2/CoFe2O4 photocatalyst system, the photogenerated h+ and ∙OH were the main active species responsible for reacting with MB dye. As the h+ and ∙OH species were required for ring-opening and complete degradation of MB molecules into less harmful products of CO2, H2O, or inorganic ions [Citation63], the formation of heterojunction interfaces between CeO2/CoFe2O4 could improve the photocatalytic activity by facilitating the interfacial charge separation and increasing the availability of reactive species of h+ and ∙OH. It is interesting to note that some of the photogenerated e− in the CeO2 CB might also react with the adsorbed O2 to form reactive ∙O2− as the CeO2 ECB (−0.31 eV) is more negative than the single electron reduction potential of O2 (-0.046 eV vs. NHE [Citation24]). The quenching experiment by using radical scavengers may require confirming the main active species during the photocatalytic reaction [Citation64,Citation65].
The magnetic properties of CoFe2O4 and 20%CeO2/CoFe2O4 photocatalysts at room temperature were studied by VSM technique. The magnetic hysteresis loops (M-H loops) of CoFe2O4 and 20%CeO2/CoFe2O4 () showed a superparamagnetic behavior by having nearly no hysteresis loops and low coercivity (Hc) and remanent magnetization (Mr) [Citation7,Citation14]. CoFe2O4 had Hc = 106.4 Oe, Mr = 4.7 emu.g−1, and saturated magnetization (Ms) = 44.1 emu.g−1. In the case of 20%CeO2/CoFe2O4, the Ms value decreased to 33.4 emu.g−1 while Hc and Mr increased to 209.1 Oe and 5.3 emu.g−1 due to the presence of the non-magnetic phase of CeO2 [Citation44]. The inset in shows that the suspended magnetic 20%CeO2/CoFe2O4 photocatalysts could easily be separated from the treated solution by applying an external magnetic field. The 20%CeO2/CoFe2O4 photocatalysts still preserved a good magnetic response and facilitated the separation and recovery of photocatalysts from a dye solution after the photodegradation reaction, which benefits the practical use of photocatalysis technology in wastewater treatment.
Figure 10. The M-H loops of CoFe2O4 and 20%CeO2/CoFe2O4 under a magnetic field. Inset: a photograph showing the magnetic 20%CeO2/CoFe2O4 photocatalysts separated by an external magnetic field.

The reusability of the magnetic 20%CeO2/CoFe2O4 heterojunction photocatalyst for MB dye degradation was examined by magnetically separating the solid photocatalysts from the solution, washing, drying, and reusing in the same experimental procedures by using 14 mg photocatalyst dosage in 10 mg.L−1 MB concentration. The photodegradation efficiency of the reused 20%CeO2/CoFe2O4 in the second cycle was 25.2%, which was 65.9% lower than the efficiency of the fresh photocatalyst (73.8%). The reduction in activity through the recycling test probably resulted from strong adsorption of residual MB on the photocatalyst surface that decreased the surface-active sites. shows FT-IR spectra of the fresh and reused magnetic 20%CeO2/CoFe2O4 photocatalyst and MB. The reused 20%CeO2/CoFe2O4 showed the characteristic absorption bands of CoFe2O4 and CeO2 at 595 cm−1 for spinel-type oxide Fe-O and 409 cm−1 for Co-O and Ce-O [Citation19,Citation66], together with the absorption bands due to the vibrational modes of MB (C16H18N3SCl·3 H2O) at 3390 cm−1 for O-H stretching, 3100, 3025, and 2804 cm−1 for C-H stretching of the aromatic group, 2770 cm−1 for C-H stretching of an alkyl group, 1590–1330 cm−1 for C-C stretching in aromatic rings, 1140 cm−1 for C-N stretching and 1021 cm−1 for C-S-C stretching [Citation67,Citation68]. These results confirmed that adsorbed MB on the photocatalyst surface was responsible for reducing photocatalytic activity in the reusability test. Therefore, removing the adsorbed MB molecules by treating the photocatalysts with a mind acid solution of 0.0001 M hydrochloric acid (HCl) for 20 min prior to reuse is required to regenerate the photocatalysts and maintain the performance [Citation65].
4. Conclusion
This study showed the improvement of photocatalytic activity CoFe2O4 by coupling with CeO2. The magnetically separable CeO2/CoFe2O4 nanocomposites were prepared by a precipitation method with different CeO2 loadings at 10, 20, and 30 wt%. The CeO2/CoFe2O4 nanocomposites had a higher MB dye degradation under UV irradiation than pure CoFe2O4 and CeO2. 20%CeO2/CoFe2O4 photocatalyst showed the highest photocatalytic efficiency and rate constant due to the uniform dispersion of the CeO2 minor phase throughout the CoFe2O4 major phase. The formation of CeO2/CoFe2O4 heterojunctions improved the photocatalytic activity by facilitating the effective charge separation and increasing the availability of reactive species of h+ and ∙OH for MB degradation. The magnetically separable CeO2/CoFe2O4 photocatalysts are suitable for practical application in wastewater treatment as they can easily be recovered after the photodegradation reaction by applying an external magnetic field.
Acknowledgments
This work was supported by the [School of Science, King Mongkut’s Institute of Technology Ladkrabang (KMITL)] under Grant [number 2565-02-05-019]. We thank the Scientific Instruments Center, Faculty of Science, King Mongkut’s Institute of Technology Ladkrabang.
Disclosure statement
No potential conflict of interest was reported by the author(s).
Additional information
Funding
References
- Sonu DV, Sharma S Review on augmentation in photocatalytic activity of CoFe2O4 via heterojunction formation for photocatalysis of organic pollutants in water. J Saudi Chem Soc. 2019;23(8):1119–1136. 2019/12/01/. doi: 10.1016/j.jscs.2019.07.003
- Hosny NM, Gomaa I, Elmahgary MG. Adsorption of polluted dyes from water by transition metal oxides: a review. Appl Surf Sci. 2023;15:100395. 2023/06/01/. doi: 10.1016/j.apsadv.2023.100395.
- Islam T, Repon MR, Islam T, et al. Impact of textile dyes on health and ecosystem: a review of structure, causes, and potential solutions. Environ Sci Pollut Res. 2023;30(4):9207–9242. 2023/01/01. doi: 10.1007/s11356-022-24398-3
- Rafiq A, Ikram M, Ali S, et al. Photocatalytic degradation of dyes using semiconductor photocatalysts to clean industrial water pollution. J Ind Eng Chem. 2021;97:111–128. 2021/05/25/ doi: 10.1016/j.jiec.2021.02.017
- Nguyen V-H, Nguyen B-S, Jin Z, et al. Towards artificial photosynthesis: sustainable hydrogen utilization for photocatalytic reduction of CO2 to high-value renewable fuels. Chem Eng J. 2020;402:126184. 2020/12/15/ doi: 10.1016/j.cej.2020.126184
- Foteinis S, Chatzisymeon E. 4 - Heterogeneous photocatalysis for water purification. In: Boukherroub R, Ogale S, and Robertson N, editors. Nanostructured photocatalysts. Amsterdam, Netherlands: Elsevier; 2020. pp. 75–97. doi:10.1016/B978-0-12-817836-2.00004-1.
- Hasija V, Nguyen V-H, Kumar A, et al. Advanced activation of persulfate by polymeric g-C3N4 based photocatalysts for environmental remediation: a review. J Hazard Mater. 2021;413:125324. 2021/07/05/ doi: 10.1016/j.jhazmat.2021.125324
- Rajeshwar K, Osugi ME, Chanmanee W, et al. Heterogeneous photocatalytic treatment of organic dyes in air and aqueous media. J Photochem Photobiol C Photochem Rev. 2008;9(4):171–192. 2008/12/01/. doi: 10.1016/j.jphotochemrev.2008.09.001
- Kumari P, Bahadur N, Kong L, et al. Engineering schottky-like and heterojunction materials for enhanced photocatalysis performance – a review [10.1039/D1MA01062J]. Mater Adv. 2022;3(5):2309–2323. :
- Venci X, George A, Raj AD, et al. Photocatalytic degradation effect of CdSe nanoparticles for textile wastewater effluents at low cost and proves to be efficient method. Environ Res. 2022;213:113595. 2022/10/01/ doi: 10.1016/j.envres.2022.113595
- Krishnan A, Swarnalal A, Das D, et al. A review on transition metal oxides based photocatalysts for degradation of synthetic organic pollutants. J Environ Sci. 2024;139:389–417. doi:10.1016/j.jes.2023.02.051.
- Thasirisap E, Vittayakorn N, Seeharaj P. Surface modification of TiO2 particles with the sono-assisted exfoliation method. Ultrason Sonochem. 2017;39:733–740. 2017/11/01/. doi: 10.1016/j.ultsonch.2017.06.002.
- Murillo-Sierra JC, Hernández-Ramírez A, Hinojosa-Reyes L, et al. A review on the development of visible light-responsive WO3-based photocatalysts for environmental applications. Chem Eng J Adv. 2021;5:100070. 2021/03/15/ doi: 10.1016/j.ceja.2020.100070
- Koppala S, Xia Y, Zhang L, et al. Hierarchical ZnO/Ag nanocomposites for plasmon-enhanced visible-light photocatalytic performance. Ceram Int. 2019;45(12):15116–15121. 2019/08/15/. doi: 10.1016/j.ceramint.2019.04.252
- Jiang X, Kong D, Luo B, et al. Preparation of magnetically retrievable flower-like AgBr/BiOBr/NiFe2O4 direct Z-scheme heterojunction photocatalyst with enhanced visible-light photoactivity. Colloids Surf A Physicochem Eng Asp. 2022;633:127880. 2022/01/20/ doi: 10.1016/j.colsurfa.2021.127880
- Jiang X, Wang M, Luo B, et al. Magnetically recoverable flower-like Sn3O4/SnFe2O4 as a type-II heterojunction photocatalyst for efficient degradation of ciprofloxacin. J Alloys Compd. 2022;926:166878. 2022/12/10/ doi: 10.1016/j.jallcom.2022.166878
- Duan K, Que T, Koppala S, et al. A facile route to synthesize n-SnO 2 /p-CuFe 2 O 4 to rapidly degrade toxic methylene blue dye under natural sunlight. RSC Adv. 2022;12(26):16544–16553. :
- Sonu SS, Dutta V An overview of heterojunctioned ZnFe2O4 photocatalyst for enhanced oxidative water purification. J Environ Chem Eng. 2021;9(5):105812. 2021/10/01/. doi: 10.1016/j.jece.2021.105812
- Abroushan E, Farhadi S, Zabardasti A. Ag 3 PO 4 /CoFe 2 O 4 magnetic nanocomposite: synthesis, characterization and applications in catalytic reduction of nitrophenols and sunlight-assisted photocatalytic degradation of organic dye pollutants. RSC Adv. 2017;7(30):18293–18304. doi: 10.1039/C7RA01728F
- El-Shobaky GA, Turky AM, Mostafa NY, et al. Effect of preparation conditions on physicochemical, surface and catalytic properties of cobalt ferrite prepared by coprecipitation. J Alloys Compd. 2010;493(1):415–422. 2010/03/18/. doi: 10.1016/j.jallcom.2009.12.115
- Pasupong P, Choojun K, Vittayakorn N, et al. Synthesis of nanocrystalline cobalt ferrite by the sonochemical method in highly basic aqueous solution. Key Eng Mater. 2017;751:368–373. doi: 10.4028/www.scientific.net/KEM.751.368
- Cannas C, Falqui A, Musinu A, et al. CoFe2O4 nanocrystalline powders prepared by citrate-gel methods: synthesis, structure and magnetic properties. J Nanopart Res. 2006;8(2):255–267. 2006/04/01. doi: 10.1007/s11051-005-9028-7
- Hafeez HY, Lakhera SK, Narayanan N, et al. Environmentally sustainable synthesis of a CoFe2O4–TiO2/rGO Ternary photocatalyst: a highly efficient and stable photocatalyst for high production of hydrogen (solar fuel). ACS Omega. 2019;4(1):880–891. 2019/01/31. doi: 10.1021/acsomega.8b03221
- Zhang L, Zhang A, Lu H, et al. Magnetically separable AgI–BiOI/CoFe 2 O 4 hybrid composites for Hg 0 removal: characterization, activity and mechanism. RSC Adv. 2017;7(50):31448–31456. :
- Yang H. A short review on heterojunction photocatalysts: carrier transfer behavior and photocatalytic mechanisms. Mater Res Bull. 2021;142:111406. 2021/10/01/. doi: 10.1016/j.materresbull.2021.111406.
- Kumar R, Sudhaik A, Sonu, et al. Graphene oxide modified K, P co-doped g-C3N4 and CoFe2O4 composite for photocatalytic degradation of antibiotics. J Taiwan Inst Chem Eng. 2023;150:105077. 2023/09/01/ doi: 10.1016/j.jtice.2023.105077
- Sharma K, Hasija V, Patial S, et al. Recent progress on MXenes and MOFs hybrids: structure, synthetic strategies and catalytic water splitting. Int J Hydrogen Energy. 2023;48(17):6560–6574. 2023/02/26/. doi: 10.1016/j.ijhydene.2022.01.004
- Sathishkumar P, Mangalaraja RV, Anandan S, et al. CoFe2O4/TiO2 nanocatalysts for the photocatalytic degradation of reactive red 120 in aqueous solutions in the presence and absence of electron acceptors. Chem Eng J. 2013;220:302–310. 2013/03/15/ doi: 10.1016/j.cej.2013.01.036
- Ibrahim I, Belessiotis GV, Elseman AM, et al. Magnetic TiO2/CoFe2O4 photocatalysts for degradation of organic dyes and pharmaceuticals without oxidants. Nanomaterials. 2022;12(19):3290. :
- Fu W, Yang H, Li M, et al. Anatase TiO2 nanolayer coating on cobalt ferrite nanoparticles for magnetic photocatalyst. Mater Lett. 2005;59(27):3530–3534. 2005/11/01/. doi: 10.1016/j.matlet.2005.06.071
- Wilson A, Mishra SR, Gupta R, et al. Preparation and photocatalytic properties of hybrid core–shell reusable CoFe2O4–ZnO nanospheres. J Magn Magn Mater. 2012;324(17):2597–2601. 2012/08/01/. doi: 10.1016/j.jmmm.2012.02.009
- Li Z, Ai J, Ge M. A facile approach assembled magnetic CoFe2O4/AgBr composite for dye degradation under visible light. J Environ Chem Eng. 2017;5(2):1394–1403. 2017/04/01/. doi: 10.1016/j.jece.2017.02.024
- Duangjam S, Wetchakun K, Phanichphant S, et al. Hydrothermal synthesis of novel CoFe2O4/BiVO4 nanocomposites with enhanced visible-light-driven photocatalytic activities. Mater Lett. 2016;181:86–91. 2016/10/15/ doi: 10.1016/j.matlet.2016.06.024
- Mahdikhah V, Ataie A, Akbari Moayyer H, et al. Magnetic and photocatalytic properties of CoFe2O4/Ni nanocomposites. J Electroceram. 2022;48(1):51–66. 2022/02/01. doi: 10.1007/s10832-021-00271-6
- Fu Y, Chen H, Sun X, et al. Combination of cobalt ferrite and graphene: high-performance and recyclable visible-light photocatalysis. Appl Catal B Environ. 2012;111-112:280–287. 2012/01/12/ doi: 10.1016/j.apcatb.2011.10.009
- Mahdikhah V, Saadatkia S, Sheibani S, et al. Outstanding photocatalytic activity of CoFe2O4/rGO nanocomposite in degradation of organic dyes. Opt Mater. 2020;108:110193. 2020/10/01/ doi: 10.1016/j.optmat.2020.110193
- Gan L, Xu L, Qian K. Preparation of core-shell structured CoFe2O4 incorporated Ag3PO4 nanocomposites for photocatalytic degradation of organic dyes. Mater Design. 2016;109:354–360. 2016/11/05/. doi: 10.1016/j.matdes.2016.07.043.
- Huang S, Xu Y, Xie M, et al. Synthesis of magnetic CoFe2O4/g-C3N4 composite and its enhancement of photocatalytic ability under visible-light. Colloids Surf A Physicochem Eng Asp. 2015;478:71–80. 2015/08/05/ doi: 10.1016/j.colsurfa.2015.03.035
- Xiong P, Chen Q, He M, et al. Cobalt ferrite–polyaniline heteroarchitecture: a magnetically recyclable photocatalyst with highly enhanced performances [10.1039/C2JM31522J]. J Mater Chem. 2012;22(34):17485–17493. :
- Magesh G, Viswanathan B, Viswanath B, et al. Photocatalytic behavior of CeO2-TiO2 system for the degradation of methylene blue. Indian J Chem. 2009;48A:480–488.
- Abdullah H, Khan MR, Pudukudy M, et al. CeO2-TiO2 as a visible light active catalyst for the photoreduction of CO2 to methanol. J Rare Earths. 2015;33(11):1155–1161. 2015/11/01/. doi: 10.1016/S1002-0721(14)60540-8
- Seeharaj P, Vittayakorn N, Morris J, et al. CeO2/CuO/TiO2 heterojunction photocatalysts for conversion of CO2 to ethanol. Nanotechnology. 2021;32(37):375707. 2021/06/24. doi: 10.1088/1361-6528/ac08be
- Seeharaj P, Kongmun P, Paiplod P, et al. Ultrasonically-assisted surface modified TiO(2)/rGO/CeO2 heterojunction photocatalysts for conversion of CO2 to methanol and ethanol. Ultrason Sonochem. 2019 Nov;58:104657
- Wetchakun N, Chaiwichain S, Wetchakun K, et al. Synthesis and characterization of novel magnetically separable CoFe2O4/CeO2 nanocomposite photocatalysts. Mater Lett. 2013;113:76–79. 2013/12/15/ doi: 10.1016/j.matlet.2013.09.008
- Zhu F, Ji Q, Lei Y, et al. Efficient degradation of orange II by core shell CoFe2O4–CeO2 nanocomposite with the synergistic effect from sodium persulfate. Chemosphere. 2022;291:132765. 2022/03/01/ doi: 10.1016/j.chemosphere.2021.132765
- Ghasemi S, Setayesh SR, Habibi-Yangjeh A, et al. Assembly of CeO2–TiO2 nanoparticles prepared in room temperature ionic liquid on graphene nanosheets for photocatalytic degradation of pollutants. J Hazard Mater. 2012;199-200:170–178. 2012/01/15/ doi: 10.1016/j.jhazmat.2011.10.080
- Hezam A, Namratha K, Drmosh QA, et al. CeO2 nanostructures enriched with oxygen vacancies for photocatalytic CO2 reduction. ACS Appl Nano Mater. 2020;3(1):138–148. 2020/01/24. doi: 10.1021/acsanm.9b01833
- Prabhakaran T, Mangalaraja RV, Denardin JC. Controlling the size and magnetic properties of nano CoFe2O4 by microwave assisted co-precipitation method. Mater Res Express. 2018;5(2):026102. 2018/02/01. doi: 10.1088/2053-1591/aaa73f
- Seeharaj P, Charoonsuk T, Pasupong P, et al. Phase formation, microstructure, and densification of yttrium-doped barium zirconate prepared by the sonochemical method. Int J Applied Ceramic Tech. 2016;13(1):200–208. :
- Channei D, Inceesungvorn B, Wetchakun N, et al. Photocatalytic degradation of methyl orange by CeO2 and Fe–doped CeO2 films under visible light irradiation. Sci Rep. 2014;4(1):5757. 2014/08/29. doi: 10.1038/srep05757
- Duru IP. Electronic and magnetic properties of CoFe2O4 nanostructures: an ab-initio and Monte Carlo study. Phys B Condens Matter. 2022;627:413548. 2022/02/15/. doi: 10.1016/j.physb.2021.413548.
- Nadjia L, Abdelkader E, Naceur B, et al. CeO2 nanoscale particles: synthesis, characterization and photocatalytic activity under UVA light irradiation. J Rare Earths. 2018;36(6):575–587. 2018/06/01/. doi: 10.1016/j.jre.2018.01.004
- Charan C, Shahi VK. Cobalt ferrite (CoFe 2 O 4) nanoparticles (size: ∼10 nm) with high surface area for selective non-enzymatic detection of uric acid with excellent sensitivity and stability. RSC Adv. 2016;6(64):59457–59467. doi: 10.1039/C6RA08746A
- Cannas C, Ardu A, Peddis D, et al. Surfactant-assisted route to fabricate CoFe2O4 individual nanoparticles and spherical assemblies. J Colloid Interface Sci. 2010;343(2):415–422. 2010/03/15/. doi: 10.1016/j.jcis.2009.12.007
- Pourgolmohammad B, Masoudpanah SM, Aboutalebi MR. Synthesis of CoFe2O4 powders with high surface area by solution combustion method: effect of fuel content and cobalt precursor. Ceram Int. 2017;43(4):3797–3803. 2017/03/01/. doi: 10.1016/j.ceramint.2016.12.027
- Yuejuan W, Jingmeng M, Mengfei L, et al. Preparation of high-surface area Nano-CeO2 by template-assisted precipitation method. J Rare Earths. 2007;25(1):58–62. 2007/02/01/. doi: 10.1016/S1002-0721(07)60045-3
- Saikia H, Hazarika KK, Chutia B, et al. A simple chemical route toward high surface area CeO2 nanoparticles displaying remarkable radical scavenging activity. ChemistrySelect. 2017;2(11):3369–3375. :
- Guo M-N, Guo C-X, Jin L-Y, et al. Nano-sized CeO2 with extra-high surface area and its activity for CO oxidation. Mater Lett. 2010;64(14):1638–1640. 2010/07/31/. doi: 10.1016/j.matlet.2010.04.018
- Nassar MY, Khatab M. Cobalt ferrite nanoparticles via a template-free hydrothermal route as an efficient nano-adsorbent for potential textile dye removal [10.1039/C6RA12852A]. RSC Adv. 2016;6(83):79688–79705. doi: 10.1039/C6RA12852A
- De Faria LA, Trasatti S. The point of zero charge of CeO2. J Colloid Interface Sci. 1994;167(2):352–357. 1994/10/15/. doi: 10.1006/jcis.1994.1370
- Azeez F, Al-Hetlani E, Arafa M, et al. The effect of surface charge on photocatalytic degradation of methylene blue dye using chargeable titania nanoparticles. Sci Rep. 2018;8(1):7104. 2018/05/08. doi: 10.1038/s41598-018-25673-5
- Fan Z, Meng F, Gong J, et al. Enhanced photocatalytic activity of hierarchical flower-like CeO2/TiO2 heterostructures. Mater Lett. 2016;175:36–39. 2016/07/15/ doi: 10.1016/j.matlet.2016.03.136
- Khan I, Saeed K, Zekker I, et al. Review on methylene blue: its properties, uses, toxicity and photodegradation. Water. 2022;14(2):242. :
- Ye H, Xia L, Wang Y, et al. Magnetic Ag3PO4/CoFe2O4 Z-scheme heterojunction material for photocatalytic decomposition of ofloxacin. J Mater Sci Mater Electron. 2023;34(31):2090. 2023/11/05. doi: 10.1007/s10854-023-11567-4
- Ranjith KS, Rajendra Kumar RT. Regeneration of an efficient, solar active hierarchical ZnO flower photocatalyst for repeatable usage: controlled desorption of poisoned species from active catalytic sites [10.1039/C6RA27380G]. RSC Adv. 2017;7(9):4983–4992. doi: 10.1039/C6RA27380G
- Kulthananat T, Kim-Lohsoontorn P, Seeharaj P. Ultrasonically assisted surface modified CeO2 nanospindle catalysts for conversion of CO2 and methanol to DMC. Ultrason Sonochem. 2022;90:106164. 2022/11/01/. doi: 10.1016/j.ultsonch.2022.106164.
- Pradhan AC, Paul A, Rao GR. Sol-gel-cum-hydrothermal synthesis of mesoporous Co-Fe@Al2O3−MCM-41 for methylene blue remediation. J Chem Sci. 2017;129(3):381–395. 2017/03/01. doi: 10.1007/s12039-017-1230-5
- Ovchinnikov OV, Evtukhova AV, Kondratenko TS, et al. Manifestation of intermolecular interactions in FTIR spectra of methylene blue molecules. Vib Spectrosc. 2016;86:181–189. 2016/09/01/ doi: 10.1016/j.vibspec.2016.06.016