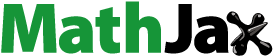
ABSTRACT
Owing to the advancement of bioactive materials, bioactive glasses (BGs) are progressively being recognized as promising choices in fields of bone and tissue engineering with their superior properties such as bioactivity and biocompatibility. However, their applications are limited by the fact that the material itself does not possess antibacterial properties. To address this limitation, antibacterial BGs with silver-zinc co-dopant were proposed and successfully synthesized via the one-step spray pyrolysis technique in this work. The phase composition, chemical composition, surface morphology, and nanoscale structures of the obtained BG specimens were characterized using X-ray diffraction, energy dispersive spectroscopy, scanning electron microscopy, and transmission electron microscopy. Moreover, in-vitro bioactivity was assessed through immersion of the SBF solution, while the in-vitro antibacterial property was examined using Escherichia coli bacteria. Meanwhile, the viability of MC3T3-E1 osteoblast cells was evaluated using the 3-(4,5-dimethylthiazol-2-yl)-2,5-diphenyltetrazolium bromide assay. Finally, the results showed positive effects of zinc addition, and the influence of silver and zinc dopants on the in-vitro bioactivity, antibacterial property, and cell viability were discussed.
1. Introduction
Bioactive glasses (BGs) based on the SiO2, CaO, and P2O5 system have been extensively studied as popular candidates in the biomedical field owing to their excellent biological properties [Citation1], such as osteoinductivity, osteoconductivity, and the ability to induce bone-like mineral phase with the host tissues [Citation2,Citation3]. However, in applications like bone grafts or dental implants, bacterial infection is a frequent post-implantation issue that could be lethal [Citation4,Citation5]. Due to the fact that BGs implants lack sufficient antibacterial characteristics, their practical applicability is highly constrained. Consequently, it is highly required to enhance the antibacterial capabilities of BGs implants [Citation6,Citation7].
For antibacterial properties, studies have demonstrated that incorporating metal ions could provide potential antibacterial properties against common bacteria such as Escherichia coli (E. coli) and Staphylococcus aureus (S. aureus) [Citation8]. For instance, metal ions such as copper (Cu) [Citation9], magnesium (Mg) [Citation10], strontium (Sr) [Citation11], cerium (Ce) [Citation12], zirconium (Zr) [Citation13], silver (Ag) [Citation14], zinc (Zn) [Citation15], and etc. have been added into BG system as therapeutic agents to enhance their biological properties. Among these metal ions, Ag+ and Zn2+ have gained particular interest owing to their several biological functions. For example, dopant of Ag+ was chosen to enhance antibacterial efficiency of BGs [Citation16,Citation17]; dopant of Zn2+ to stimulate the differentiation and proliferation of osteoblasts cells [Citation18]. Recently, Azizabadi et al. [Citation19] have demonstrated that combining both Ag+ and Zn2+ ions into a 58S BG compound is more effective than doping only Ag+ ions in terms of antibacterial, bioactivity, and biocompatibility. In another study, the presence of Ag+ and Zn2+ in BG system showed enhancement of chemical resistance while decreasing ion leaching. The slower dissolution rate results in a reduction in the production of the silica-rich layer, which is followed by a slowdown in the apatite layer formation [Citation20]. Additionally, substitution Ca2+ by Ag+ and Zn2+ ions has been proposed in BG system owing to the similarity in charge and ionic radius (Ag+ = 1.13 Å, Zn2+ = 0.78 Å, Ca2+ = 0.94 Å) [Citation16,Citation19].
Meanwhile, various synthetic methods, such as melt-quenching [Citation21], co-precipitation [Citation22], sol-gel [Citation23], spray drying [Citation24], and spray pyrolysis [Citation25], have been reported to synthesize BG. However, determining the most efficient method of synthesizing the BGs has always been a challenge [Citation26,Citation27]. First, studies have reported that the melting-quenched BGs powders showed low in-vitro bioactivity because of their low porosity [Citation28,Citation29]. In addition, a high calcination temperature (1200 to 1500°C) is required, whereas the boiling point of Zn is 907°C, making the melt-quenching method incompatible with Zn dopant. Next, Hong et al. [Citation30] demonstrated the preparation of BG with co-precipitation method, the result showed that the obtained BG powders were severely agglomerated, which may affect the dopant distribution of metal ions. Finally, for the sol-gel method, Balamurugan et al. [Citation31] used the sol-gel method for the synthesis of SiO2-CaO-P2O5-ZnO BGs and reported reduce of bioactivity. Although the methods mentioned above emphasize good chemical stability, disadvantages of lengthy preparation times and discontinuous processing make them impractical for large production. Additionally, the spray pyrolysis method offers promising options owing to its simplicity, waste reduction, rapid calcination, continuous processing, and scalable production [Citation32]. This method is effective for the preparation of BG, in which the powder is prepared through a liquid phase with stoichiometric and homogeneous compounds by spraying the solutions into a tube furnace [Citation33]. In our previous studies, this technique has been applied to synthesize BG powders [Citation34–36], and has demonstrated promising results with spray pyrolyzed BG powders with submicron size, spherical morphology, and non-aggregation characteristics.
Therefore, in the current work, we aimed to employ the spray pyrolysis technique to prepare specimens of un-doped BG, Ag-doped BG, and Ag/Zn co-doped BG. Moreover, the obtained powders were subjected to analysis using X-ray diffraction (XRD), energy-dispersive X-ray spectroscopy (EDS), scanning electron microscopy (SEM), and transmission electron microscopy (TEM). Further, in-vitro bioactivity was evaluated via simulated body fluid (SBF), in-vitro antibacterial property was performed against E. coli bacteria, and in-vitro cytotoxicity was studied toward mouse osteoblast cells (MTT assays). Finally, the formation mechanisms, antibacterial activity, and cell viability behaviors of all BG specimens were discussed.
2. Materials and methods
2.1. Preparation
In this work, all BG specimens, including un-doped, Ag-doped, and Ag/Zn co-doped, were fabricated via spray pyrolysis method. Note that the composition of 76S (SiO2: CaO: P2O5 = 80: 15: 5 in mol%) was chosen for its structural homogeneity and phase stability. To start with, 6.70 g of tetraethyl orthosilicate (Si(OC2H5)4, Showa, 99.9%), 0.73 g of triethyl phosphate ((C2H5)3PO4, Alfa Aesar, 99.0%), and 1.40 g of calcium nitrate tetrahydrate (Ca(NO3)2·4 H2O, Showa, 98.5%) were dissolved into 40.00 g ethanol and 1.00 g of HCl for the preparation of precursor solution of the un-doped BG specimen. The solution was stirred for 1 h at 25°C to obtain homogenous in a transparent condition. Then, de-ionized water was added till 400 mL and kept stirring for an additional 24 h. Meanwhile, for the Ag-doped and Ag/Zn co-doped BG specimens, silver nitrate (AgNO3, Echo, 99.0%) and zinc nitrate hexahydrate (Zn(NO3)·6 H2O, Sigma Aldrich, 98.0%) were added into BGs precursor solution. Next, the final precursor solutions were atomized using an ultrasonic nebulizer (King Ultrasonic, KT-100A, Taiwan) with a frequency of 1.65 MHz. The atomized droplets were navigated into a quartz tube furnace (Denying, D110, Taiwan) with three heat-treatment zones: preheated, pyrolysis, and cooling, with temperatures set at 400, 700, and 500°C, respectively. Lastly, the powders were collected with a 16 kV earthed stainless steel tube.
3. Characterization
First, the phase compositions of all specimens were determined using an X-ray diffractometer (D2 Phaser Bruker, Germany) with the 2θ = 20° to 80° in 0.05° steps using Ni-filtered Cu radiation Kα radiation. The surface morphologies were observed using a field-emission scanning electron microscope (SEM, JEOL 6500F, Japan), while the particle size distributions were collected by averaging more than 300 particles for each BG specimen. Additionally, the elemental compositions were acquired via energy-dispersive X-ray spectroscopy (EDS). Next, the nanoscale structures and d-spacing were visualized using a transmission electron microscope (TEM, Tecnai G2 F20, US). Moreover, the specific surface areas of the specimens were evaluated by Brunauer-Emmett-Teller (BET) method. The specimens were degassed at 150°C for 3 h and placed onto an N2 desorption instrument with an operating temperature of −196°C (Quantachrome Instruments, Novatouch LX2, US).
In-vitro antibacterial activities were examined against E. coli (strain ATCC 10,536) and evaluated by the colony-counting method [Citation37]. Initially, a single colony of bacteria was suspended in Lysogeny Broth media and cultured in an incubator (Firstek Scientific, S300R, Taiwan) at 37°C for 24 h. Then, the bacteria suspension was diluted to 1:100 with phosphate-buffered saline (PBS) solution at a concentration of 6 × 106 CFU/mL. Next, 1 mg of specimens were diluted into 0.5 ml of bacteria dilution and carefully spread onto agar plates. Finally, after incubating for 24 h, the grown colonies were counted using a Rocker galaxy 330 colony counter, and the antibacterial rate percentage was calculated following the formula below:
For the assessment of in-vitro bioactivity, Kokubo’s protocol [Citation38] was used. All BG specimens were immersed into the SBF solution, with a solid-to-solution ratio of 0.02 g/mL. The solutions were then placed into an incubator at 37°C for 7 d. It is worth noting that the SBF solution was refreshed once a day. To prevent further reaction, the specimens were rinsed three times with both deionized water and acetone and stored in an oven at 70°C for 1 day to remove any excess solution. Finally, the bioactivity was evaluated by using both SEM and Fourier-transform infrared spectroscope (FTIR, Digilab, FTS-1000, US) with wavenumbers ranging from 400 to 1600 cm−1.
At last, evaluation of in-vitro cytotoxicity was conducted by 3-(4,5-dimethylthiazol-2-yl)-2,5-diphenyltetrazolium bromide (MTT) assay following ISO 10,993–5 protocol [Citation39]. Initially, osteoblast-like cells (MC3T3-E1) were cultured in Modified Broker Eagle medium-α (MEM-α) with 10% fetal bovine serum (FBS) and 100 U/mL streptomycin-penicillin at 37°C under 5% CO2 atmosphere. Then, the cells were seeded into 24-well plates at a density of 2 × 104 cells/cm2. A stock suspension (100 μg/mL) of specimens was prepared in a medium and incubated for 24 h. Next, the suspensions were transferred into 96-well plates and incubated for 24, 48, and 72 h, respectively. The colorimetric viability was determined by adding MTT solutions into each well. After that, the media were carefully removed, and rinsed the formazan residue by adding dimethyl sulfoxide (DMSO, Sigma). Finally, a multi-well microplate reader (Multiskan Go, Thermo Scientific, USA) was used to measure the optical density (OD) at a wavelength of 570 nm. The cell viability was then computed based on the following formula:
4. Results
First, the phase compositions of un-doped BG, Ag-doped BG, and Ag/Zn co-doped BG were characterized using XRD. According to , broad bands across 20 to 40° Could be observed from the XRD patterns of un-doped BG, 2.5 mol% Ag-doped BG, and 2.5 mol% Ag/2.5 mol% Zn co-doped BG specimens, indicating the presence of amorphous structures. In contrast, the 5 mol% Ag-doped BG specimens showed four diffraction peaks at 38.11°, 44.30°, 64.44°, and 77.39°, which corresponded to (111), (200), (220), and (311) crystal planes of Ag, showing that the 5.0 mol% Ag-doped BG has a crystalline structure. In brief, the XRD results revealed that no crystalline impurity peaks, e.g. Ca2SiO4, CaCO3, etc., were identified within all BG specimens.
Figure 1. XRD patterns of un-doped, 2.5 mol% Ag-doped, 5.0 mol% Ag-doped, and 2.5 mol% Ag/2.5 mol% Zn co-doped BG specimens.

The surface morphologies of all BG specimens were investigated using SEM along with their corresponding particle size distributions. As shown in , spherical shapes with diameters ranging from 0.2 to 2.4 μm could be observed from all BG specimens. Moreover, the particle size distributions of un-doped BG, 2.5 mol% Ag-doped BG, 5.0 mol% Ag-doped BG, and 2.5 mol% Ag/2.5 mol% Zn co-doped BG specimens were statistically computed as 0.62 ± 0.28, 0.61 ± 0.29, 0.62 ± 0.28, 0.66 ± 0.30, and 0.61 ± 0.31 μm, respectively. In addition, the EDX spectra were recorded and shown in to confirm the presence of Ag and Zn in all BG specimens. shows the EDX spectrum of un-doped BG, which exhibited peaks of O-Kα, Si-Kα, p-Kα, and Ca-Kα at 0.52, 1.76, 2.02, and 3.70 keV, respectively. Furthermore, for 2.5 mol% Ag-doped BG and 5.0 mol% Ag-doped BG (shown in ), an additional peak of Ag-Lα was observed at 2.98 keV, which confirmed the presence of Ag in the BG system. Further, for the 2.5 mol% Ag/2.5 mol% Zn co-doped BG, two additional peaks of Ag-Lα and Zn-Lα were detected at 2.98 and 1.02 keV. The EDX spectra analysis results demonstrated that all BG specimens are high purity and qualitatively consistent with the nominal composition. Meanwhile, the detailed elemental compositions calculated based on the EDS spectra are shown in . In the case of Ag-doped BG specimens, the results show that the atomic percentage of Ag is proportional to the dopant concentration. In addition, for the Ag/Zn co-doped BG, the presence of both Ag and Zn elements could be detected. These results suggested that the dopant of Ag and Ag/Zn into the BG structure was successful.
Figure 2. SEM images of (a) un-doped, (b) 2.5 mol% Ag-doped, (c) 5.0 mol% Ag-doped, and (d) 2.5 mol% Ag/2.5 mol% Zn co-doped BG specimens.

Figure 3. EDS analysis of (a) un-doped, (b) 2.5 mol% Ag-doped, (c) 5.0 mol% Ag-doped, and (d) 2.5 mol% Ag/2.5 mol% Zn co-doped BG specimens.

Table 1. Atomic compositions of BG, 2.5 mol% Ag-doped BG, 5.0 mol% Ag-doped BG, and 2.5 mol% Ag/2.5 mol% Zn co-doped BG.
For the nanoscale structures, TEM was employed for observation of un-doped BG, Ag-doped BG, and Ag/Zn co-doped BGs, and the resulting images with magnified insets are shown in . To start with, it could be seen from that the un-doped BG specimen exhibited smooth spherical morphologies. Moreover, for the Ag-doped BG and Ag/Zn co-doped BG, similar spherical morphologies were observed with additional nanoparticles found on the surface of BG particles (). These nanoparticles showed darker contrast as compared to the BG particles, indicating that these nanoparticles have a higher atomic number than BG [Citation40]. Furthermore, to examine the nanoparticles, the high-resolution TEM images along with their corresponding magnified lattice images of 2.5 mol% Ag-doped BG and 2.5 mol% Ag/2.5 mol% Zn co-doped BG were recorded and shown in . The results showed interplanar lattice fringes of 0.24 nm, which corresponds to the lattice constant of Ag phase [Citation41]. Additionally, the particle diameter distributions of Ag nanoparticles were measured statistically, suggesting that the average particle sizes are around 8.53 ± 6.20, 9.01 ± 2.13, and 4.49 ± 1.79 nm for the 2.5 mol% Ag-doped BG, 5.0 mol% Ag-doped BG, and 2.5 mol% Ag/2.5 mol% Zn co-doped BG, respectively. Finally, the compositional distribution of Ag and Zn in the BG particles was investigated using STEM-HAADF imaging. shows the STEM-HAADF and EDS mapping results of 2.5 mol% Ag/2.5 mol% Zn co-doped BG specimens. shows the HAADF-STEM image, and the dark field image illustrates both BG particles and metal ions (Ag and Zn) precipitates. While the results of EDS mapping indicate the presence of Si, Ag, and Zn and their homogeneous distribution within the BG particles. These results suggested that Ag and Zn were successfully doped in the BG system.
Figure 4. TEM images of (a) un-doped, (b) 2.5 mol% Ag-doped, (c) 5.0 mol% Ag-doped, and (d) 2.5 mol% Ag/2.5 mol% Zn co-doped BG specimens.

Figure 5. HR-TEM images of (a) 2.5 mol% Ag-doped BG and (b) 2.5 mol% Ag/2.5 mol% Zn co-doped BG specimens, along with (c, d) their corresponding magnified lattice images.

Figure 6. (a) STEM-HAADF image and the corresponding (b) Si-Kα, (c) Ag-Kα, and (d) Zn-Kα EDS maps of 2.5 mol% Ag/2.5 mol% Zn co-doped BG specimen.

Further, the in-vitro antibacterial activities of all BG specimens were assessed and shown in . The photograph of surviving E. coli on agar plates is shown in , whereas the white dots represent the surviving colonies. As shown in , many bacterial colonies on the agar plate could be observed from the un-doped BG specimens, indicating poor antibacterial activity. In addition, for 2.5 mol% Ag-doped BG specimens, the bacterial colonies were reduced as compared to the un-doped specimen, while 5.0 mol% Ag-doped BG and 2.5 mol% Ag/2.5 mol% Zn co-doped BG had almost no bacterial colonies across whole agar plates. Moreover, shows the antibacterial rates which were computed against the control. The graph shows that the antibacterial rate of un-doped BG, 2.5 mol% Ag-doped BG, 5.0 mol% Ag-doped BG, and 2.5 mol% Ag/2.5 mol% Zn co-doped BG were 7.8%, 55.1%, 99.3%, and 100%, respectively. The above results indicated that 5.0 mol% Ag-doped BG and 2.5 mol% Ag/2.5 mol% Zn co-doped BG specimens had the best antibacterial performance against E. coli bacteria compared to BG and 2.5 mol% Ag-doped BG, while the numbers of bacteria-colony representing antibacterial activities followed an order of 5.0 mol% Ag-doped BG ≈2.5 mol% Ag/2.5 mol% Zn co-doped BG >2.5 mol% Ag-doped BG > un-doped BG.
Figure 7. Photographs of the antibacterial results of (a) control, (b) un-doped, (c) 2.5 mol% Ag-doped, (d) 5.0 mol% Ag-doped, and (e) 2.5 mol% Ag/2.5 mol% Zn co-doped BG specimens against E. coli bacteria.

Figure 8. Antibacterial rates of un-doped, 2.5 mol% Ag-doped, 5.0 mol% Ag-doped, and 2.5 mol% Ag/2.5 mol% Zn co-doped BG specimens against E. coli bacteria.

The in-vitro bioactivity was assessed by characterizing the formation of the hydroxyapatite (HA) layer on the BG surface using SEM and FTIR. To begin with, shows the SEM images of all BG specimens after 7 d of SBF immersion with insets of magnified images of the marked regions. Compared to the as-prepared specimens, as shown in , precipitations of the HA could be observed on the surface of all BG specimens. In addition, EDS spectra were recorded to examine the elemental composition and the computation of the Ca/P ratio. The results showed that the Ca/P ratios are 1.55 ± 0.16, 1.17 ± 0.06, 1.13 ± 0.20, and 1.12 ± 0.04 for un-doped BG, 2.5 mol% Ag-doped BG, 5.0 mol% Ag-doped BG, and 2.5 mol% Ag/2.5 mol% Zn co-doped BG, respectively. In brief, both SEM and EDS results confirmed the HA formation of all BG specimens. Further, investigation via FTIR was carried out for quantification of the bioactivity. As shown in , a band at 482 cm−1 was observed due to Si-O-Si bending vibration (denoted as I2), corresponding to the BG matrix. Meanwhile, the peaks that occurred in the region between 566 cm−1 are recognized as the PO43− asymmetric bending state (denoted as I1), corresponding to the precipitated HA layer [Citation42]. Note that the baseline was corrected by subtracting the signal from the spectrum to remove background noise and interference. Combining both integrated intensities, the ratio of I1/I2 is computed to quantify the formation of HA layer, which are 0.18 ± 0.01, 0.16 ± 0.01, 0.13 ± 0.01, and 0.13 ± 0.02 for un-doped BG, 2.5 mol% Ag-doped BG, 5.0 mol% Ag-doped BG, and 2.5 mol% Ag/2.5 mol% Zn co-doped BG, respectively. The results showed an order of in-vitro bioactivity as follows: un-doped BG >2.5 mol% Ag-doped BG >5.0 mol% Ag-doped BG ≈2.5 mol% Ag/2.5 mol% Zn co-doped BG specimens. In brief, the growth of the HA layer on the surface was observed after 7 d immersion in SBF, confirming the in-vitro bioactivity of all BG specimens.
Figure 9. SEM images of (a) un-doped, (b) 2.5 mol% Ag-doped, (c) 5.0 mol% Ag-doped, and (d) 2.5 mol% Ag/2.5 mol% Zn co-doped BG specimens after SBF immersion for 7 d, with insets showing the magnified images of the marked regions.

Figure 10. FTIR spectra of un-doped, 2.5 mol% Ag-doped, 5.0 mol% Ag-doped, and 2.5 mol% Ag/2.5 mol% Zn co-doped BG specimens (a) before and (b) after SBF immersion for 7 d.

Finally, the in-vitro cytotoxicity of all BG specimens was evaluated against MC3T3-E1 cells via the MTT assay, and the cell viability after incubation (24 h, 48 h, and 72 h) is shown in , demonstrating the proliferation and adhesion of MC3T3-E1 cells onto the BG specimens. It can be seen from the graph that, after 24 h of incubation, the un-doped BG, 2.5 mol% Ag-doped BG, and 2.5 mol% Ag/2.5 mol% Zn co-doped BG specimens showed no cytotoxic effect toward the cells. In contrast, the 5.0 mol% Ag-doped BG specimens exhibited a significant inhibitory effect on the MC3T3-E1 cells. Furthermore, after longer incubation durations of 48 and 72 h, the cell viability of un-doped BG, 2.5 mol% Ag-doped BG, and 2.5 mol% Ag/2.5 mol% Zn co-doped BG specimens increased, indicating the positive effect on the proliferation of MC3T3-E1 cells. In comparison, the 5.0 mol% Ag-doped BG specimens showed a decrease in cell viability than other BG specimens, indicating a negative impact on cell proliferation. In summary, the in-vitro cytotoxicity results demonstrated that Ag/Zn co-doped BG specimen could improve the cellular responses and increase the cell viability of MC3T3-E1 cells effectively.
Figure 11. Cell viabilities of un-doped, 2.5 mol% Ag-doped, 5.0 mol% Ag-doped, and 2.5 mol% Ag/2.5 mol% Zn co-doped BG specimens on MC3T3-E1 cell incubated for 24, 48, and 72 hours (* presents the statistical differences with respect to control, n = 3 and p < 0.05; # presents the statistical differences with respect to un-doped BG powder).

5. Discussion
First, for the phase information, the XRD patterns () revealed that no impurity peaks could be spotted within the un-doped BG specimen, indicating a successful synthesis of amorphous structure [Citation43,Citation44]. In addition, similar patterns of amorphous structure could also be observed from all other BG specimens, which could probably be attributed to the low concentration that cannot be detected via XRD. Further, with increased dopant concentration, the presence of Ag phase was found within the 5.0 mol% Ag-doped BG specimen.
Next, the morphology and nanoscale structures of spray pyrolyzed BG specimens were discussed. During spray pyrolysis, particle formation could be divided into two mechanisms: gas-to-particle and one-particle-per-droplet [Citation45]. As depicted in , the SEM and TEM images revealed that all BG specimens showed the morphology of spheres with diameters measured at approximately 600 nm. This suggested that the morphology obtained in this study is dominated by the one-particle-per-droplet mechanism. Moreover, the TEM and HRTEM analyses confirmed that nanoparticles with a d-spacing of 0.24 nm, corresponding to the (111) crystal plane of Ag, within both Ag- and Ag/Zn co-doped BG particles. Additionally, the images showed that both BG specimens had Ag nanoparticles with diameters smaller than 100 nm. This suggested that the formation of Ag nanoparticles is based on the gas-to-particle conversion, facilitated by the high calcination temperature (700°C), which is higher than the melting point of the silver nitrate precursor (444°C) [Citation46]. It is worth noting that the average diameter of Ag nanoparticles for Ag-doped BG specimens is about 10 nm, whereas for the Ag/Zn co-doped BG specimens is around 5 nm. Based on our previous study [Citation47], we have demonstrated that the addition of Zn could result in a reduction of the size of Ag nanoparticles, which is attributed to the exothermic property of zinc nitrate precursor. Therefore in this study, the Ag nanoparticles of Ag/Zn co-doped BG specimens have a smaller particle diameter and are well distributed in the BG particles, showing a consistent agreement with our previous study.
Further, we discussed the in-vitro bioactivity of un-doped BG, Ag-doped BG, and Ag/Zn co-doped BG specimens. Both SEM images () and FTIR spectra () showed that all BG specimens were bioactive due to the observed HA layer formation. Moreover, various studies have suggested the influence of Ag ions substitution on in-vitro properties of BG [Citation48–50]. For instance, El-Kady et al. [Citation49] demonstrated that substituting Ca with Ag in the BG system will decrease the amount of non-bridging oxygen groups for the Si dissolution, thus delaying the BG disintegration. Furthermore, Rahmani et al. [Citation51] proved that adding Ag ions into the BG system will retard the formation of HA. In addition, Shahrbabak et al. [Citation52] showed that doping Ag and Zn into BG via the sol-gel method will decrease the bioactivity properties. All the above studies indicated that the dopant of Ag or Zn might decrease the bioactivity properties, which is consistent with the in-vitro bioactivity results in this study, resulting in an order of un-doped BG >2.5 mol% Ag-doped BG >2.5 mol% Ag/2.5 mol% Zn co-doped BG ≈5.0 mol% Ag-doped BG specimens.
For the antibacterial activity, showed that the un-doped BG specimen has an antibacterial rate of around 7.8% against E. coli bacteria. This could be explained by an increase in the pH of the medium caused by BG dissolution [Citation53]. Furthermore, the antibacterial rate of the 2.5 mol% Ag-doped BG and 5.0 mol% Ag-doped BG specimens were measured as 55.1% and 99.3%. Based on previous studies, the leaching of Ag ions could trigger the antibacterial activity and thus have been used as an antibacterial agent for a long period of time [Citation54,Citation55]. In addition, mechanisms have been discussed to validate the antibacterial efficacy of Ag nanoparticles against bacteria [Citation48,Citation54,Citation56]. These mechanisms include the release of Ag ions can easily penetrate through the cell membrane, followed by the formation of reactive oxygen species and blocked ATP and DNA transcription, all of which result in the death of the bacteria [Citation57]. Similar to Ag ions, Zn ions share the same antibacterial mechanism, which includes interacting with the cell membrane, binding to proteins, and deactivating them to produce an anti-bacterial effect [Citation58,Citation59]. However, Ag ions have a greater antibacterial value than Zn ions [Citation60]. Moreover, the 2.5 mol% Ag/2.5 mol% Zn co-doped BG demonstrated an antibacterial activity of 100%. This antibacterial efficacy could be related to the size of the Ag nanoparticles. Based on the TEM observation, the average Ag nanoparticles size of 2.5 mol% Ag/2.5 mol% Zn co-doped BG observed is around 5 nm, which is smaller than the Ag nanoparticle size of 2.5 mol% Ag-doped BG and 5.0 mol% Ag-doped BG of around 10 nm, indicating an increase in the specific surface area about 4 times. Thus, it could be associated with a higher inhibitory effect [Citation61,Citation62]. This result is in agreement with Sotiriou et al. [Citation63] who reported that Ag ions with a high specific surface area enhanced antibacterial activity against E. coli. In our previous study [Citation47], the positive correlation between specific surface area and antibacterial efficiency of Ag nanoparticles was also demonstrated.
Finally, the evaluation of in-vitro cytotoxicity was examined after 24 h, 48 h, and 72 h. The results demonstrated that the cell viability of un-doped BG, 2.5 mol% Ag-doped BG, and 2.5 mol% Ag/2.5 mol% Zn co-doped BG specimens had almost similar percentage after 24 h incubation, whereas the cell viability of Ag/Zn co-doped BG was noticeably improved after 48 h and 72 h incubation. In contrast, the cell viability of 5.0 mol% Ag-doped BG specimens was decreased significantly with longer incubation time. According to previous studies, high Ag ion concentration will induce cytotoxicity in cells [Citation64,Citation65], while Luo et al. [Citation50] showed that the cell viability will decrease with the increase of Ag concentration up to 2 wt% in the borate BG. While the cell viability of Ag-doped BG is influenced by the concentration of Ag within the BG, the addition of Zn may lead to improvements due to their positive impact on bone formation. For instance, Zn has the ability to stimulate bone regeneration, enhance osteogenic functions in osteoblasts, and promote cell proliferation. Therefore, in another work, Azizabadi et al. [Citation19] reported that cell viability could be improved by the of Ag and Zn in the BG system, which is consistent with our results in this study, suggesting that the co-dopant of Ag and Zn could improve the cell growth of MC3T3E1 cells.
6. Conclusion
In this study, both Ag- and Ag/Zn co-doped BG specimens were fabricated successfully via spray pyrolysis method. The surface morphologies and nanostructures of all BG specimens were characterized by SEM and TEM, and the corresponding formation mechanisms of particle formation and Ag distribution were discussed. Next, the in-vitro bioactivity was confirmed by the formation of the crystalline HA layer via FTIR analysis. Moreover, the resulting antibacterial activity revealed that both 5.0 mol% Ag-doped BG and 2.5 mol% Ag/2.5 mol% Zn co-doped BG have similar antibacterial efficacy of nearly 100%. In addition, the in-vitro cell viability demonstrated that the Ag/Zn co-doped BG has the highest MT3C3-E1 cell viability. Considering the bioactivity, antibacterial property, and cell viability, Ag/Zn co-doped BG specimen is a promising candidate and could be applied as a suitable bioceramic material for orthopedic and dental applications.
Acknowledgments
The authors acknowledge the financial support from the National Science and Technology Council of Taiwan (Grant number NSTC 112-2221-E-011-049) and the Taipei Medical University/National Taiwan University of Science and Technology cross-university collaboration project (Grant number TMU-NTUST-112-05).
Disclosure statement
No potential conflict of interest was reported by the author(s).
Additional information
Funding
References
- Arcos D, Vallet-Regí M. Sol–gel silica-based biomaterials and bone tissue regeneration. Acta Biomaterialia. 2010;6(8):2874–2888. doi: 10.1016/j.actbio.2010.02.012
- Baino F, Fiorilli S, Vitale-Brovarone C. Bioactive glass-based materials with hierarchical porosity for medical applications: review of recent advances. Acta Biomaterialia. 2016;42:18–32. doi: 10.1016/j.actbio.2016.06.033
- Izquierdo-Barba I, Vallet-Regí M. Mesoporous bioactive glasses: relevance of their porous structure compared to that of classical bioglasses. Biomedical Glasses. 2015;1(1). doi: 10.1515/bglass-2015-0014
- Moghanian A, Firoozi S, Tahriri M, et al. RETRACTED: a comparative study on the in vitro formation of hydroxyapatite, cytotoxicity and antibacterial activity of 58S bioactive glass substituted by Li and Sr. Mater Sci Eng C. 2018;91:349–360. doi: 10.1016/j.msec.2018.05.058
- Capela M, Tobaldi D, Oliveira C, et al. Bioactivity and antibacterial activity against E-coli of calcium-phosphate-based glasses: effect of silver content and crystallinity. Ceram Int. 2017;43(16):13800–13809. doi: 10.1016/j.ceramint.2017.07.100
- Gupta N, Santhiya D, Murugavel S, et al. Effects of transition metal ion dopants (ag, Cu and Fe) on the structural, mechanical and antibacterial properties of bioactive glass. Colloids Surf A Physicochem Eng Asp. 2018;538:393–403. doi: 10.1016/j.colsurfa.2017.11.023
- Hu S, Chang J, Liu M, et al. Study on antibacterial effect of 45S5 bioglass®. J Mater Sci. 2009;20(1):281–286. doi: 10.1007/s10856-008-3564-5
- Stankic S, Suman S, Haque F, et al. Pure and multi metal oxide nanoparticles: synthesis, antibacterial and cytotoxic properties. J Nanobiotechnol. 2016;14(1):1–20. doi: 10.1186/s12951-016-0225-6
- Kargozar S, Mozafari M, Ghodrat S, et al. Copper-containing bioactive glasses and glass-ceramics: from tissue regeneration to cancer therapeutic strategies. Mater Sci Eng: C. 2020;121:111741. doi: 10.1016/j.msec.2020.111741
- Moghanian A, Sedghi A, Ghorbanoghli A, et al. The effect of magnesium content on in vitro bioactivity, biological behavior and antibacterial activity of sol–gel derived 58S bioactive glass. Ceram Int. 2018;44(8):9422–9432. doi: 10.1016/j.ceramint.2018.02.159
- Fei Y-C, Chen L-G, Kuo C-K, et al. Influence of strontium dopant on bioactivity and osteoblast activity of spray pyrolyzed strontium-doped mesoporous bioactive glasses. J Asian Ceram Soc. 2021;9(1):221–228. doi: 10.1080/21870764.2020.1860437
- Saatchi A, Arani AR, Moghanian A, et al. Synthesis and characterization of electrospun cerium-doped bioactive glass/chitosan/polyethylene oxide composite scaffolds for tissue engineering applications. Ceram Int. 2021;47(1):260–271. doi: 10.1016/j.ceramint.2020.08.130
- Moghanian A, Zohourfazeli M, Tajer MHM. The effect of zirconium content on in vitro bioactivity, biological behavior and antibacterial activity of sol-gel derived 58S bioactive glass. J Non-Crystalline Solids. 2020;546:120262. doi: 10.1016/j.jnoncrysol.2020.120262
- Sharifianjazi F, Parvin N, Tahriri M. Formation of apatite nano-needles on novel gel derived SiO2-P2O5-CaO-SrO-Ag2O bioactive glasses. Ceram Int. 2017;43(17):15214–15220. doi: 10.1016/j.ceramint.2017.08.056
- Bai X, Liu W, Xu L, et al. Sequential macrophage transition facilitates endogenous bone regeneration induced by Zn-doped porous microcrystalline bioactive glass. J Mat Chem B. 2021;9(12):2885–2898. doi: 10.1039/D0TB02884C
- El-Rashidy AA, Waly G, Gad A, et al. Preparation and in vitro characterization of silver-doped bioactive glass nanoparticles fabricated using a sol-gel process and modified stöber method. J Non-Crystalline Solids. 2018;483:26–36. doi: 10.1016/j.jnoncrysol.2017.12.044
- Sharifianjazi F, Parvin N, Tahriri M. Synthesis and characteristics of sol-gel bioactive SiO2-P2O5-CaO-Ag2O glasses. J Non-Crystalline Solids. 2017;476:108–113. doi: 10.1016/j.jnoncrysol.2017.09.035
- Neščáková Z, Zheng K, Liverani L, et al. Multifunctional zinc ion doped sol–gel derived mesoporous bioactive glass nanoparticles for biomedical applications. Bioact Mater. 2019;4:312–321. doi: 10.1016/j.bioactmat.2019.10.002
- Azizabadi N, Azar PA, Tehrani MS, et al. Synthesis and characteristics of gel-derived SiO2-CaO-P2O5-SrO-Ag2O-ZnO bioactive glass: bioactivity, biocompatibility, and antibacterial properties. J Non-Crystalline Solids. 2021;556:120568. doi: 10.1016/j.jnoncrysol.2020.120568
- Baghbani F, Moztarzadeh F, Mozafari M, et al. Production and characterization of a Ag-and Zn-doped glass-ceramic material and in vitro evaluation of its biological effects. J Materi Eng Perform. 2016;25(8):3398–3408. doi: 10.1007/s11665-016-2156-7
- Ben-Arfa BA, Pullar RC. A comparison of bioactive glass scaffolds fabricated by Robocasting from powders made by sol–gel and melt-quenching methods. Processes. 2020;8(5):615. doi: 10.3390/pr8050615
- Ji L, Qiao W, Huang K, et al. Synthesis of nanosized 58S bioactive glass particles by a three-dimensional ordered macroporous carbon template. Mater Sci Eng C. 2017;75:590–595. doi: 10.1016/j.msec.2017.02.107
- Zheng K, Boccaccini AR. Sol-gel processing of bioactive glass nanoparticles: a review. Adv Colloid Interfac. 2017;249:363–373. doi: 10.1016/j.cis.2017.03.008
- Ningsih HS, Liu Y-C, Chen J-W, et al. Effects of strontium dopants on the in vitro bioactivity and cytotoxicity of strontium-doped spray-dried bioactive glass microspheres. J Non-Crystalline Solids. 2022;576:121284. doi: 10.1016/j.jnoncrysol.2021.121284
- Kuo C-K, Chen L-G, Tseng C-F, et al. Influences of acid catalysts on the microstructure, bioactivity and cytotoxicity of bioactive glass nanoparticles prepared by spray pyrolysis. J Non-Crystalline Solids. 2021;560:120710. doi: 10.1016/j.jnoncrysol.2021.120710
- Lucas-Girot A, Mezahi FZ, Mami M, et al. Sol–gel synthesis of a new composition of bioactive glass in the quaternary system SiO2–CaO–Na2O–P2O5: comparison with melting method. J Non-Crystalline Solids. 2011;357(18):3322–3327. doi: 10.1016/j.jnoncrysol.2011.06.002
- Sepulveda P, Jones J, Hench L. In vitro dissolution of melt‐derived 45S5 and sol‐gel derived 58S bioactive glasses. J Biomed Mater Res. 2002;61(2):301–311. doi: 10.1002/jbm.10207
- Begum AN, Rajendran V, Ylänen H. Effect of thermal treatment on physical properties of bioactive glass. Materials Chemistry & Physics. 2006;96(2–3):409–417. doi: 10.1016/j.matchemphys.2005.07.031
- Jones JR. Review of bioactive glass: from Hench to hybrids. Acta Biomaterialia. 2013;9(1):4457–4486. doi: 10.1016/j.actbio.2012.08.023
- Hong Z, Liu A, Chen L, et al. Preparation of bioactive glass ceramic nanoparticles by combination of sol–gel and coprecipitation method. J Non-Crystalline Solids. 2009;355(6):368–372. doi: 10.1016/j.jnoncrysol.2008.12.003
- Balamurugan A, Balossier G, Kannan S, et al. Development and in vitro characterization of sol–gel derived CaO–P2O5–SiO2–ZnO bioglass. Acta Biomaterialia. 2007;3(2):255–262. doi: 10.1016/j.actbio.2006.09.005
- Chen C-Y, Lyu Y-R, Su C-Y, et al. Characterization of spray pyrolyzed manganese oxide powders deposited by electrophoretic deposition technique. Surf Coat Technol. 2007;202(4–7):1277–1281. doi: 10.1016/j.surfcoat.2007.07.083
- Messing G, Zhang S, Jayanthi G. Modeling of particle morphology and size. J Am Ceram Soc. 1993;76(11):2707. doi: 10.1111/j.1151-2916.1993.tb04007.x
- Shih S-J, Chou Y-J, Chien I-C. One-step synthesis of bioactive glass by spray pyrolysis. J Nanopart Res. 2012;14(12):1–8. doi: 10.1007/s11051-012-1299-1
- Tseng C-F, Fei Y-C, Chou Y-J. Investigation of in vitro bioactivity and antibacterial activity of manganese-doped spray pyrolyzed bioactive glasses. J Non-Crystalline Solids. 2020;549:120336. doi: 10.1016/j.jnoncrysol.2020.120336
- Shih S-J, Tzeng W-L, Jatnika R, et al. Control of Ag nanoparticle distribution influencing bioactive and antibacterial properties of Ag-doped mesoporous bioactive glass particles prepared by spray pyrolysis. J Biomed Mater Res, Part B. 2014;103(4):899–907. doi: 10.1002/jbm.b.33273
- Brugger SD, Baumberger C, Jost M, et al. Automated counting of bacterial colony forming units on agar plates. PloS One. 2012;7(3):e33695. doi: 10.1371/journal.pone.0033695
- Kokubo T, Kushitani H, Sakka S, et al. Solutions able to reproduce in vivo surface-structure changes in bioactive glass-ceramic A-W 3. J Biomed Mater Res. 1990;24(6):721–734. doi: 10.1002/jbm.820240607
- Mosmann T. Rapid colorimetric assay for cellular growth and survival: application to proliferation and cytotoxicity assays. J Immunol Methods. 1983;65(1–2):55–63. doi: 10.1016/0022-1759(83)90303-4
- Zhou Y, Zan Y, Zheng S, et al. Distribution of the microalloying element Cu in B4C-reinforced 6061Al composites. J Alloys Compd. 2017;728:112–117. doi: 10.1016/j.jallcom.2017.08.273
- Tseng H-C, Chen Y-W. Facile synthesis of Ag/TiO2 by photoreduction method and its degradation activity of methylene blue under UV and visible light irradiation. Mod Res Catal. 2019;9(1):1. doi: 10.4236/mrc.2020.91001
- Akhtach S, Tabia Z, Bricha M, et al. Structural characterization, in vitro bioactivity, and antibacterial evaluation of low silver-doped bioactive glasses. Ceram Int. 2021;47(20):29036–29046. doi: 10.1016/j.ceramint.2021.07.066
- Bogle K, Dhole S, Bhoraskar V. Silver nanoparticles: synthesis and size control by electron irradiation. Nanotechnology. 2006;17(13):3204. doi: 10.1088/0957-4484/17/13/021
- Zamiri R, Zakaria A, Husin MS, et al. Formation of silver microbelt structures by laser irradiation of silver nanoparticles in ethanol. Int J Nanomed. 2011;6:2221. doi: 10.2147/IJN.S23830
- Shih S-J, Chien I-C. Preparation and characterization of nanostructured silver particles by one-step spray pyrolysis. Powder Technol. 2013;237:436–441. doi: 10.1016/j.powtec.2012.12.032
- Zimmermann R, Pfuch A, Horn K, et al. An approach to create silver containing antibacterial coatings by use of atmospheric pressure plasma chemical vapour deposition (APCVD) and combustion chemical vapour deposition (CCVD) in an economic way. Plasma Process Polym. 2011;8(4):295–304. doi: 10.1002/ppap.201000113
- Chou Y-J, Ningsih HS, Shih S-J. Preparation, characterization and investigation of antibacterial silver-zinc co-doped β-tricalcium phosphate by spray pyrolysis. Ceram Int. 2020;46(10):16708–16715. doi: 10.1016/j.ceramint.2020.03.245
- Qian G, Zhang L, Liu X, et al. Silver-doped bioglass modified scaffolds: a sustained antibacterial efficacy. Mater Sci Eng C. 2021;129:112425. doi: 10.1016/j.msec.2021.112425
- El-Kady AM, Ali AF, Rizk RA, et al. Synthesis, characterization and microbiological response of silver doped bioactive glass nanoparticles. Ceram Int. 2012;38(1):177–188. doi: 10.1016/j.ceramint.2011.05.158
- Luo SH, Xiao W, Wei XJ, et al. In vitro evaluation of cytotoxicity of silver‐containing borate bioactive glass. J Biomed Mater Res, Part B. 2010;95(2):441–448. doi: 10.1002/jbm.b.31735
- Rahmani M, Moghanian A, Yazdi MS. The effect of ag substitution on physicochemical and biological properties of sol-gel derived 60% SiO2–31% CaO–4% P2O5–5% Li2O (mol%) quaternary bioactive glass. Ceram Int. 2021;47(11):15985–15994. doi: 10.1016/j.ceramint.2021.02.173
- Shahrbabak MSN, Sharifianjazi F, Rahban D, et al. A comparative investigation on bioactivity and antibacterial properties of sol-gel derived 58S bioactive glass substituted by Ag and Zn. Silicon. 2019;11(6):2741–2751. doi: 10.1007/s12633-018-0063-2
- Zhang D, Leppäranta O, Munukka E, et al. Antibacterial effects and dissolution behavior of six bioactive glasses. J Biomed Mater Res. 2010;93(2):475–483. doi: 10.1002/jbm.a.32564
- Bellantone M, Williams HD, Hench LL. Broad-spectrum bactericidal activity of Ag2O-doped bioactive glass. Antimicrob Agents Chemother. 2002;46(6):1940–1945. doi: 10.1128/AAC.46.6.1940-1945.2002
- Silver S, Phung LT. Bacterial heavy metal resistance: new surprises. Annu Rev Microbiol. 1996;50(1):753–789. doi: 10.1146/annurev.micro.50.1.753
- Feng QL, Wu J, Chen GQ, et al. A mechanistic study of the antibacterial effect of silver ions on Escherichia coli and staphylococcus aureus. J Biomed Mater Res. 2000;52(4):662–668. doi: 10.1002/1097-4636(20001215)52:4<662:AID-JBM10>3.0.CO;2-3
- Khodashenas B. The influential factors on antibacterial behaviour of copper and silver nanoparticles. Indian Chem Eng. 2016;58(3):224–239. doi: 10.1080/00194506.2015.1026950
- Devanand Venkatasubbu G, Ramasamy S, Ramakrishnan V, et al. Nanocrystalline hydroxyapatite and zinc-doped hydroxyapatite as carrier material for controlled delivery of ciprofloxacin, 3 biotech. 3 Biotech. 2011;1(3):173–186. doi: 10.1007/s13205-011-0021-9
- Nath S, Kalmodia S, Basu B. Densification, phase stability and in vitro biocompatibility property of hydroxyapatite-10 wt% silver composites. J Mater Sci Mater Med. 2009;21(4):1273–1287. doi: 10.1007/s10856-009-3939-2
- Wu J, Wang L, He J, et al. In vitro cytotoxicity of Cu2+, Zn2+, Ag+ and their mixtures on primary human endometrial epithelial cells. Contraception. 2012;85(5):509–518. doi: 10.1016/j.contraception.2011.09.016
- Lok C-N, Ho C-M, Chen R, et al. Silver nanoparticles: partial oxidation and antibacterial activities. J Biol Inorg Chem. 2007;12(4):527–534. doi: 10.1007/s00775-007-0208-z
- Sotiriou GA, Pratsinis SE. Antibacterial activity of nanosilver ions and particles. Environ Sci Technol. 2010;44(14):5649–5654. doi: 10.1021/es101072s
- Sotiriou GA, Teleki A, Camenzind A, et al. Nanosilver on nanostructured silica: Antibacterial activity and Ag surface area. Chem Eng J. 2011;170(2–3):547–554. doi: 10.1016/j.cej.2011.01.099
- Phetnin R, Rattanachan ST. Preparation and antibacterial property on silver incorporated mesoporous bioactive glass microspheres. J Sol Gel Sci Techn. 2015;75(2):279–290. doi: 10.1007/s10971-015-3697-1
- Ahamed M, Karns M, Goodson M, et al. DNA damage response to different surface chemistry of silver nanoparticles in mammalian cells. Toxicol Appl Pharmacol. 2008;233(3):404–410. doi: 10.1016/j.taap.2008.09.015