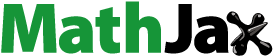
ABSTRACT
Environmentally friendly Pb-free piezoelectric ceramics are essential for electronic industries and devices utilizations owing to their excellent piezoelectric properties which enable powerful sensor, transducer and actuator developments. In the present work, highly dense complex perovskite oxide ceramics with the formula of (1-x)[0.94Bi0.5Na0.5TiO3-0.06BaTiO3]-x(Ba0.7Sr0.3)TiO3 or (1-x)[0.94BNT–0.06BT]-xBST, where x = 0.00, 0.01, 0.02, 0.03, 0.04 and 0.05 mol fraction were fabricated by a solid-state mixed oxide method. After sintering at 1125°C for 2 h, all ceramics were characterized with attention paid on their phases, microstructures, dielectric, ferroelectric, piezoelectric and strain properties as a function of BST content. The unit all size expansion i.e. higher degree of tetragonality was detected as BST content increased. All compositions exhibited single perovskite phase and uniform microstructures containing cubic-like grains with average grain size range of all ceramics was in the order of ~ 0.75–1.57 µm, depending on the amount of BST. It was interestingly found that the addition of BST enhanced the densification, sintering ability, grain size and electrical properties of BNT-BT ceramics, indicating their potential Pb-free piezoelectric candidates for future actual applications.
1. Introduction
Lead-based perovskite materials such as PZT-based systems are the most widely used piezoelectric ceramics industrially manufactured in large scale in the world today. Their low-firing temperature which enables low-cost electrodes and excellent piezoelectric properties make these materials keep up with the trend of electrochromic applications and for energy harvesting applications [Citation1–7]. Moreover, their key fundamental investigation and technological importance have been extensively demonstrated in the literature [Citation1–8]. However, it was reported earlier that the volatilization of the lead oxide (PbO) at high temperature during heat treatment processes causing toxicity environmental pollution and uncertainty in their electrical properties is one of the major obstacles of these Pb-based ceramics [Citation8–12]. Hence, there has been a great deal of interest in the search for novel alternative lead-free piezoelectric materials that can take over the existed Pb-based compounds, in order to continue environmentally sustainable development. The challenges for further evolution of lead-free piezoelectric materials for electronic devices were systematically reviewed by Panda et al [Citation9].
Among Pb-based perovskite piezoelectric ceramics, BaTiO3 or BT, Bi0.5Na0.5TiO3 or BNT, Bi0.5K0.5TiO3 or BKT, Bi0.5(Na0.8K0.2)0.5TiO3 or BNKT and their solid-solutions are widely explored as promising candidates for further technological utilizations due to nontoxic handling and individual properties of these materials [Citation9–15]. As is well documented, assembling various materials together is one of the useful approaches to generate novel multicomponent deriving excellent properties from each component. For example, previous works have shown that the BNKT-based systems modified with a number of oxide compounds, e.g. SrTiO3 [Citation13], BiFeO3 [Citation14] and Ba0.7Sr0.3TiO3 [Citation15], exhibit enhanced piezoelectric properties. On the other hand, our previous work [Citation16] observed that the addition of LiNbO3 significantly decreases the values of d33 but increases the room temperature dielectric constant of BNKT ceramics, depending on the amount of added dopants. In recent years, there are several reports [Citation17–23] on the investigation of BNT-based solid-solutions including BNT-BT system since they are considered to be one of the most promising candidates to replace PZT due to their excellent piezoelectric performance and large remnant polarization located at or near morphotropic phase boundary (MPB).
According to the literature, BNT is one of the relaxor ferroelectric materials and its solid-solution with normal ferroelectric BT could contribute excellent piezoelectric properties. It is known that their electrical properties depend strongly on microstructure as well as chemical compositions. It is believed that the similarity of Bi and Pb ions, in views of their large number of electrons, ionic sizes and lone pair electron, leads to the deformation of unit cell and thus to their piezoelectric properties [Citation24,Citation25]. The BNT can be synthesized by solid state reaction technique. The advantages of technique include lower cost compared with other techniques, short reaction time, high purity of product, collection process of synthesized products is straightforward and it has good reaction efficiency [Citation26]. Furthermore, BNT indicated that nontoxic, eco-friendly piezoelectric material and attractive piezoelectric properties with high curie temperature (TC = 593 K), large remanent polarization (Pr = 38 μC/cm2) and high coercive field (73 kV/cm) at room temperature [Citation27,Citation28]. However, the limitation of BNT ceramics lies in their large leakage current density and high driving electric field [Citation8,Citation9]. Thus, utilizing chemical modification via additive is one of the interesting approaches. Recently, Supriya et al. reported Sr2+ doped BNT system in various forms like single crystals, thin films, multi-layers, and polycrystals for enhanced piezoelectric and ferroelectric activities [Citation29]. Ren et al [Citation30]. reported large electro-strain in Pb-free BNKT ceramics modified with Ba0.95Sr0.05TiO3 and Ta5+ ion, where the intrinsic effect of the crystal and the external electric field could be their major causes. In connection with a solid-solution of barium strontium titanate Ba1-xSrxTiO3, related works [Citation31] the maximum room temperature dielectric constant (εr ~4500) was observed at x = 0.3. Moreover, the energy harvesting performance as a key component of a piezoelectric energy harvester is the piezoelectric materials themselves, which play an important role in determining the output performance of an energy harvester [Citation32–35]. Rout et al. studied the (100-x) Na0.5Bi0.5TiO3-xSrTiO3 system 0≤x≤40 and reported the MPB for this system was found at x = 0.20 and room temperature value presented enhancement from 500 (x = 0) to 3800 (x = 40) at 1 kHz with increasing Sr content [Citation36]. Energy harvesting is the process by which energy is derived from external various sources such as wind energy, thermal energy, mechanical vibrations, or kinetic energy [Citation37–43]. Several Pb-free systems had good energy harvesting performance such as 0.94(Bi0.5Na0.5)TiO3-0.06BaTiO3 [Citation44], (K,Na,Li)(Nb,Ta,Sb)O3 [Citation45], Ba0.85Ca0.15Ti0.9Zr0.1O3 [Citation46], Ba(Zr0.1Ti0.9)O3 [Citation47], (Ba,Ca)(Zr,Ti)O3 [Citation48], Bi0.5(Na0.84K0.16)0.5TiO3/0.01ZnO [Citation49] and (1-y)[0.995Bi0.5(Na0.80K0.20)0.5TiO3-0.005LiNbO3]-y[(Ba0.7Sr0.3)TiO3] [Citation50].
Thus, Ba0.7Sr0.3TiO3 composition was chosen as the desired dopant for the BNT-BT based system in our study. Interestingly, so far, there is no systematic investigation on Pb-free BNT-BT modified with such chemical formula of Ba0.7Sr0.3TiO3 or BST. Hence, this work is the continuation of our current efforts to develop a novel alternative Pb-free piezoelectric ceramics for Pb-based ceramics replacement and we will discuss on how the different amount of BST fraction could affect the phase, structure, electrical properties and energy harvesting performance of the BNT-BT ceramics.
2. Experimental procedures
The perovskite piezoelectric ceramics of (1-x)[0.94Bi0.5Na0.5TiO3-0.06BaTiO3-x] - x(Ba0.7Sr0.3)TiO3 or (1-x)[0.94BNT–0.06BT]-xBST, where x = 0.00, 0.01, 0.02, 0.03, 0.04 and 0.05 mol fraction, were fabricated by a solid-state mixed oxide method. Commercial powders of Bi2O3 (98%, Fluka, Sigma-Aldrich Cot., St. Louis, MS), Na2CO3 (99.5%, Carlo Erba, Carlo Erba Reagenta, Peypin, France), TiO2 (99%, Riedel-de Haën, Honeywell Specialty Chemicals Seelze GmbH, Seelze, German), BaCO3 (98.5%, Fluka, Sigma-Aldrich Corp.) and SrCO3. (98%, Sigma-Aldrich, Sigma-Aldrich Corp.) were used as starting powders. The methods used for mixing, drying, and grinding of the powders were similar to those used in our earlier work [Citation16]. All starting materials were stoichiometrically weighed, ball milled for 24 h in an ethanol (99.99%) medium and then dried in an oven at 120°C for 24 h. All mixed powders were calcined at 900°C for 2 h. Calcined powders were weighted and pressed into pellets (~15 mm in diameter) under 100 MPa pressure by a uniaxial pressing machine without any binder used. Green samples were sintered in a closed alumina crucible (to minimize the loss of volatile elements) at 1125°C for 2 h with a heating/cooling rate of 5°C/min.
X-ray diffractometer (XRD-Phillip, X-pert) using CuKα radiation was employed to identify crystal structure of prepared ceramics. Lattice parameters of ceramics were determined from the d spacings obtained from diffraction peaks of a tetragonal phase [Citation16]. Tetragonality (c/a) values of all ceramics were extracted using least square analysis. Bulk densities of sintered ceramics were determined using the Archimedes’ method. Theoretical densities of all ceramics were calculated based on the reported densities values of BNT (5.99 g/cm3) [Citation51], BT (6.01 g/cm3) [Citation52] and BST (3.97 g/cm3) [Citation53]. Linear shrinkages of all ceramics were also evaluated. A scanning electron microscope (SEM, JEOL JSM-6335F) was employed to reveal microstructural features of all samples. Their grain sizes were estimated by applying a mean linear interception method (the quantitative measurement of grain size within densely uniform structure [Citation15]). All ceramic surfaces were carefully milled down to 1 mm thickness to achieved parallel scratch-free surfaces. Silver paste electrodes were made onto both parallel polished surfaces of each sample prior to firing at 600°C for 1 h in air. Dielectric constant (εr) and dielectric loss (tanδ) as a function of temperature (50–350°C) of ceramics under frequencies of 1, 10 and 100 kHz were measured by a high precision LCR meter (LCR 821, GW INSTEK). Polarization-electric field (p-E) hysteresis loops at room temperature (25°C) and at a frequency of 1 Hz were measured by a Radiant Precision ferroelectric tester under an applied electric field of 50 kV/cm. Room temperatures strain-electric field (S-E) behavior was measured by an optical displacement sensor (Fotonic Sensor model MTI-2100) under a frequency of 0.1 Hz. Maximum strain (Smax) and negative strain (Sneg) values were obtained from a bipolar curve at the maximum electric field (Emax) of 50 kV/cm. The normalized strain coefficient (d33*) of all samples was also calculated. Finally, all samples were poled in silicone oil bath at 55°C under an applied DC electric field of 40 kV/cm for 15 min. A piezoelectric coefficient (d33) value of each sample was recorded from 1 day-aged samples using a d33 meter (KCF technologies, S5865). Piezoelectric voltage constant (g33) and off-resonance figure of merit (FoM) for energy harvesting of ceramics were also evaluated in this study.
3. Results and discussion
Based on true densities of ceramics, their relative densities were calculated with respect to the theoretical densities of BNT, BT and BST [Citation51–53]. After sintering at 1125°C for 2 h, well-sintered and dense ceramics with relative density values ~ 97–98% can be achieved in all compositions. As demonstrated in , it should be noted that some correlation was found between densification (i.e. the density and the linear shrinkage values) and the amount of BST addition. Generally, with increasing BST content to x = 0.04, better densification was observed. This finding was attributed to the promotion of atomic diffusion via the expense of BST. However, a fall-off in density was observed with further increasing BST content to x = 0.05. It was believed that due to the addition of higher BST content, a grain coarsening process of the ceramic was more promoted than the densification process as will be shown in the microstructure measurement part.
Table 1. Physical properties of (1-x)Bi0.5Na0.5TiO3-0.06BaTiO3-x(Ba0.7Sr0.3)TiO3 ceramics.
In order to identify phase formation of all ceramic compositions, X-ray diffraction technique was employed. XRD patterns of BNT-BT modified with various amount of BST are shown in . In general, all samples mostly exhibited identical XRD patterns ( indicating a single phase of perovskite structure, which was consistent with literature [Citation54–57]. No diffraction peak of any impurity was detected in all patterns, reflecting a complete reaction between the starting oxide powders to form the desired products. This also implied that perovskite BST additive could be fully incorporated into the perovskite BNT-BT lattice, resulting in a formation of solid-solution ceramics. This was similar to the observed results in Refs [Citation15,Citation16,Citation56]. As shown in , the position of (111) peak at 2θ = 38–42° gradually shifted to lower 2θ angles with increasing BST content (x), implying greater tetragonality (c/a) factors of ceramics. The observed result supported mixed rhombohedral-tetragonal phase which the prediction for dominant tetragonal lattice distortion of BNT-BT system modified with tetragonal BST phase which observed in similar previous reported of BNKT-BST [Citation15]. This observation became clearer with the increasing trend in c/a values as listed in . This indicated that all BST addition could induce the lattice anisotropy of the BNT-BT system which was in analogous with those observed in BNKT-BST system [Citation15]. Similar XRD peak shifting by partial substitution of Ba2+ for BNKT ceramics was also found by Chen et al [Citation58]. Enlarged views of (200) peak splitting at 2θ ~45–48 ° were shown in . The peak split into two peaks of a tetragonal structure which were (002) and (200) planes at 2θ ~45.887° and ~ 46.559°, respectively. The change in crystal structure was mainly attributed to the difference in ionic radii of Bi3+ (~1.17 Å), Na2+ (~1.18 Å), Ti4+ (~0.61 Å), Ba2+ (~1.42 Å) and Sr2+ (~1.26 Å) [Citation59] as well as the influence of defects e.g. vacancies and/or interstitials mainly created through charge compensation mechanism. This finding was also in agreement with Jaita et al. [Citation15] and Chen et al [Citation58].
Figure 1. XRD pattern of (1-x)[0.94Bi0.5Na0.5TiO3-0.06BaTiO3]-x(Ba0.7Sr0.3)TiO3 ceramics where (a) 2θ = 20–70 degrees and (b) 2θ = 38–42 degrees, (c) 2θ = 45–48 degrees and (d) plots of FWHM versus BST content (x) and insert shows a relation between crystallite size (blue line) and average grain size (pink line) as a function of BST content (x).
![Figure 1. XRD pattern of (1-x)[0.94Bi0.5Na0.5TiO3-0.06BaTiO3]-x(Ba0.7Sr0.3)TiO3 ceramics where (a) 2θ = 20–70 degrees and (b) 2θ = 38–42 degrees, (c) 2θ = 45–48 degrees and (d) plots of FWHM versus BST content (x) and insert shows a relation between crystallite size (blue line) and average grain size (pink line) as a function of BST content (x).](/cms/asset/7abb8da3-e24b-42a1-86a9-2105d52593d5/tace_a_2314340_f0001_oc.jpg)
The full width at half maximum (FWHM) values were used for absolute XRD peak for all samples [Citation60–62]. In the present work, the FWHM value was calculated from the main peak refection of (110) as listed in . The FWHM value decreased with increasing of BST content (x), suggesting that increase in the degree of crystallinity of the samples. The crystallite size (D) was used to confirm the degree of crystallinity of all samples. The calculation was performed based on the Debye – Scherrer formula as EquationEquation 1(1)
(1) ;
where κ is the fixed number 0.9, λ is X-ray wavelength taken to be 0.15418 nm, B is the full width at half maximum (FWHM) of (hkl) reflection and θ is Bragg angle corresponding to (hkl) reflection [Citation63–70]. The crystallite size decreased when compared with undoped sample and increased from 10.08 nm (x = 0.01) to the maximum value of 14.45 nm (x = 0.05) as presented in . The increase in crystallite size could affect the electrical properties of the ceramic samples [Citation68]. Plots of FWHM versus BST content (x) and insert presenting a relation between crystallite size (blue line) and average grain size (pink line) as a function of BST content (x) in .
The Raman spectroscopy is an effective technique to determine the functional groups in solid solutions, which provides better insight into the structural study [Citation71]. Raman spectra of all compositions after sintering at 1125°C for 2 h are shown in . In previously reported data of BNT-BT-based system, there are four main regions that could be detected in the spectrum at room temperature including the mode located below 200 cm−1 associated with the vibration of perovskite A-site, the ~300 cm−1 modes related to Ti-O vibrations, the modes ~ 450–650 cm−1 related to TiO6-octahedra vibration, and the modes >700 cm−1 related to the A1 (longitudinal optical) and E (longitudinal optical) overlapping bands [Citation36,Citation72–74]. The Raman spectra of all compositions showed broad peaks due to the distorted octahedral [BiO6] and [NaO6] clusters or disorder in A-site of rhombohedral structure and the overlapping of Raman modes due to the lattice anharmonicity which can be observed in (Ba0.5Na0.5)TiO3-xBaTiO3 based system [Citation70–75]. The increase of BST content indicated that the splitting of these peaks which was observed in the regions around 279 cm−1 and 600 cm−1. This was due to the Ba2+ ions in the structure increase the average ionic radius size in the lattice of the ceramics [Citation76,Citation77]. The increase of BST concentration indicated the splitting of these peaks due to the phase transformation the mixed rhombohedral-tetragonal (for BNT-BT sample) to the tetragonal phase (for BST-doped sample) which corresponded XRD result and observed in previous report of BaTiO3-modified Bi0.5(Na0.80K0.20)0.5TiO3 system [Citation78].
Representative microstructures of all compositions are given in . As expected, typical perovskite BNT-based microstructures with dense granular grain packing derived from a solid-state sintering mechanism are revealed, similar to other reports [Citation15,Citation16,Citation56]. It is also noted that heterogeneous microstructures consisting mainly of two ranges of grains (in respect of size and shape) were observed in all compositions, it is likely that since firing temperatures for achieving highest densification of BT (~1400°C) [Citation79] and BNT (~1100°C) [Citation80] are very different, this could result in grain growth behavior variation between these two phases, hence, heterogeneous microstructures, consistent with Chaisan et al [Citation79]. As data given in , the average grain size range of all ceramics is of the order of ~ 0.75–1.57 µm. As x = 0.01 the average grain size range decreased when compared with unmodified BNT-BT ceramic. This was expected to be caused by the change in crystal structure as well as the solute drag mechanism due to the different diffusivity values of different species of added solutes (i.e. particularly Sr2+ ion). However, when x > 0.01, the grain size gradually increased in the BST-doped sample up to x = 0.05. It was likely that the increase in tetragonality and increasing Sr2+ concentration contributed to a slight increase in grain growth rate with no significant effect on grain shape. This increase in grain size trend was similarly observed in BST-doped BNKT ceramics [Citation15]. The shape of the grains was characterized by many faces (i.e. equiaxed grain morphology). This is probably because the BST phase could act as the grain growth promoter especially for the more retarding one during atomic diffusion mechanism [Citation81]. The observation that the addition of BST results in semi-isotropic grain shape (i.e. cubic-like shape) of the perovskite BNT-BT-based ceramics is also consistent with other similar works [Citation51,Citation82].
Room-temperature dielectric constant (εr) and dielectric loss (tanδ) values of all compositions monitored at frequency of 1 kHz are listed in . In this work, the unmodified BNT-BT ceramics displayed εr of 1663 which was close to the values reported in literature [Citation15,Citation16,Citation55]. When x = 0.01 of BST was added into BNT-BT ceramics, the values of εr and tanδ increased. εr drastically decreased from 1981 to 1095 and tanδ slightly decreased from 0.0820 to 0.0755. This observation could be attributed to the reduction of an internal stress within the larger grains via the formation of 90° domain mechanism [Citation1,Citation9]. However, it can be seen that with further increasing BST concentration to x = 0.04, both values of εr and tanδ slightly increased. Clearly, the increasing εr is due mainly to the increased density and crystal structure change of the samples, in good agreement with other works [Citation15,Citation79]. Another possible explanation is that the increasing grain size resulting in decreasing the volume fraction of grain boundaries (i.e. the sink of defects), thus more fraction of dielectric materials is predominant [Citation79–81]. It should be mentioned that εr and tanδ values decreased considerably with increasing BST greater than x = 0.04 and could be attributed to both grain size and density reduction. It appears that a critical chemical modification level of the BST modified BNT-BT ceramics is x = 0.04, where the maximum value of the room temperature εr of 1981 has been detected. Thus, in this study, it could be stated that densification and microstructure (i.e., grain size) of the ceramics, which are influenced by the addition of BST, are also responsible for the room temperature dielectric behavior of the ceramics. Although the correlation between grain size and electrical properties of perovskite ferroelectric ceramics have been widely explored for several decades. There are still controversies regarding the dependence of the piezoelectric and ferroelectric properties on the grain size. The temperature dependence (30–400°C) of dielectric constant (εr) and dielectric loss (tanδ) of all compositions at various frequencies (1 kHz, 10 kHz, 100 kHz and 500 kHz) are shown in . The dielectric constant value of all composition presented highest value at frequency of 1 kHz. This is due to active presence of polarizations types (i.e. space charge, electronic, ionic). The orientational polarizations cannot follow higher applied frequencies [Citation83]. Two dielectric peaks have been observed in all compositions. The peak at lower temperature is the depolarization temperature (Td) and that as higher temperature is dielectric maximum temperature (Tm) the BNT-based ceramics [Citation53,Citation84]. The maximum dielectric constant (εmax) increased with increasing of BST composition and indicated the optimum value of 5850 at x = 0.04 while the value dropped at x = 0.05 with the dielectric loss range 0.0652–0.0877. The increase of BST content indicated that broadness of dielectric peak around Td near 120°C observed in x = 0.03 and 0.04 suggesting a diffusive phase transition to relaxor-like behavior of the ceramic. This was due to compositional fluctuations occurring at A- and B-site of the relevant perovskite unit cell [Citation85]. The Td showed a slight decreasing trend with increasing of BST composition. However, Tm tended to increase with increasing BST composition as presented in . This may also be associated with the possible presence of nonpolar region in the BST doped samples [Citation84].
Figure 4. The temperature dependence of dielectric constant (εr) and dielectric loss (tanδ) of all compositions at various frequencies of (1-x)[0.94Bi0.5Na0.5TiO3-0.06BaTiO3]-x(Ba0.7Sr0.3)TiO3 ceramics.
![Figure 4. The temperature dependence of dielectric constant (εr) and dielectric loss (tanδ) of all compositions at various frequencies of (1-x)[0.94Bi0.5Na0.5TiO3-0.06BaTiO3]-x(Ba0.7Sr0.3)TiO3 ceramics.](/cms/asset/f4b367a5-fbb1-4e7a-a880-960393c28fb3/tace_a_2314340_f0004_oc.jpg)
Figure 5. (a) Plots of Tm and Td as a function of BST composition, (b) plot of Smax and d33* as a function of BST composition and (c) plot of g33 and FoM as a function of BST composition of (1-x)[0.94Bi0.5Na0.5TiO3-0.06BaTiO3]-x(Ba0.7Sr0.3)TiO3 ceramics.
![Figure 5. (a) Plots of Tm and Td as a function of BST composition, (b) plot of Smax and d33* as a function of BST composition and (c) plot of g33 and FoM as a function of BST composition of (1-x)[0.94Bi0.5Na0.5TiO3-0.06BaTiO3]-x(Ba0.7Sr0.3)TiO3 ceramics.](/cms/asset/b3870508-0073-411b-9cff-03c515f89abc/tace_a_2314340_f0005_oc.jpg)
Table 2. Electrical properties of (1-x)[0.94Bi0.5Na0.5TiO3-0.06BaTiO3]-x(Ba0.7Sr0.3)TiO3 ceramics.
The room temperature polarization electric field (p-E) hysteresis loops investigation of all compositions, measured as a function of BST addition under electric field of 50 kV/cm and frequency of 1 Hz, are shown in . The unmodified BNT-BT ceramics exhibited a typical ferroelectric characteristic of well-saturated hysteresis with square shape, indicating strong ferroelectric order in agreement with related works [Citation15,Citation79,Citation80]. As listed in , it had maximum values of Pr = 25.11 µC/cm2, Ec = 26.61 kV/cm. By comparison with the unmodified composition, the addition of BST (1–5 mol/%) displayed a significant effect on p-E hysteresis loops by causing narrower loops, indicating softer ferroelectric response, matched with the reduction of εr, Pr and Ec values. This suggested the weakening of ferroelectric order. X-ray diffraction result indicated that the lattice distortion due to tetragonality change and lattice expansion from peak shift in BST-doped samples. The ferroelectric domains were ordered while applying the electric field, and nano-domains were uniformly aligned due to lattice expansion. The resulting smaller ferroelectric loops in x = 0.01 and 0.02 samples indicated the softer ferroelectric behavior and became more relaxor-like [Citation86]. This can be explained by the chemical fluctuation within the BNT-BT system induced via the addition of BST leading to the more randomized ferroelectric domain distribution and then, resulting in the formation of defect dipoles and possibly the polar nanoregions as suggested earlier by Jaita et al. [Citation15] and Hussain et al [Citation85]. It should be that further increasing BST content, Pmax values slightly increased continuously but the p-E loops still maintain their constricted shapes (pinching), indicating dielectric softening associated with micro-polar behavior via the disordering ferroelectric mechanism [Citation1,Citation15]. The pinched loops observed for x = 0.03–0.05 samples could be due to the pinning of domain walls interacting with defects or as a result of the presence of intermediate modulated phases with inhomogeneous dipolar regions leading to the coexistence of both ferroelectric and antiferroelectric orders [Citation87]. Hence, improved understanding of their defect formation and order-disorder ferroelectric mechanism will help to guide both the development of novel Pb-free piezoceramics and the improvement of device performance for future utilization.
Figure 6. Polarization-electric field (p-E) hysteresis data for (1-x)[0.94Bi0.5Na0.5TiO3-0.06BaTiO3]-x(Ba0.7Sr0.3)TiO3 ceramics.
![Figure 6. Polarization-electric field (p-E) hysteresis data for (1-x)[0.94Bi0.5Na0.5TiO3-0.06BaTiO3]-x(Ba0.7Sr0.3)TiO3 ceramics.](/cms/asset/3636589f-e6cf-46b9-b4ce-b43243985799/tace_a_2314340_f0006_oc.jpg)
The corresponding strain “butterfly” or S-E loops is shown in . All compositions showed the butterfly-shaped strain hysteresis loop [Citation88–90]. The maximum strain (Smax), the negative strain (Sneg) and the normalized strain coefficient (d33*) were also calculated using EquationEquation 2(2)
(2) as listed in .
Figure 7. Plot of strain% as function of electric field of (1-x)[0.94Bi0.5Na0.5TiO3-0.06BaTiO3]-x(Ba0.7Sr0.3)TiO3 ceramics.
![Figure 7. Plot of strain% as function of electric field of (1-x)[0.94Bi0.5Na0.5TiO3-0.06BaTiO3]-x(Ba0.7Sr0.3)TiO3 ceramics.](/cms/asset/b64e5589-ff9b-4240-a632-185e0edbc55e/tace_a_2314340_f0007_oc.jpg)
The undoped sample has the Smax of 0.10% and d33* of 196 pm/V. With increasing BST concentration, the d33* and Smax tended to increase with the increasing of BST composition and promoted the maximum value at x = 0.04 with Smax = 0.26% and d33* = 519 pm/V. After that the value decreased at x = 0.05. Furthermore, when the added BST concentration increased, the strain curves showed drastic deviation from typical ferroelectric behavior, which was evidenced by the absence of the negative strain. This behavior was observed in other BNT-based systems [Citation70,Citation85,Citation88]. Plot of Smax and d33* as a function of BST composition are shown in .
The piezoelectric coefficient (d33) of all ceramics is also summarized in . The values of all ceramics indicated a range of 160–277 pC/N. The unmodified BNT-BT ceramic had d33 of 178 pC/N which was similar to the value reported by Jaita et al. [Citation15]. It is interesting to note that the addition of BST more than x = 0.03 can effectively enhance the d33 values of BNT-BT ceramics. The optimum of d33 was found that at the composition of x = 0.04 mol fraction with the value of 277 pC/N and dropped to 203 pC/N at composition of x = 0.05 mol fraction. Apart from their highest density observed, as already mentioned, the tetragonality enhancement of the BNT-BT ceramics modified with BST can explain better dielectric and piezoelectric properties of these ceramics. The energy harvesting properties of all ceramics were investigated by calculation. The piezoelectric voltage constant (g33) is one of the important parameters for energy harvesting applications. The piezoelectric voltage constant (g33) was calculated using EquationEquation 3(3)
(3)
where εr is the dielectric constant of the piezoelectric material, is the dielectric constant in a vacuum = 8.854 × 10−12 Fm−1 [Citation91,Citation92] and d33 is the piezoelectric charge constant. The addition of BST of x = 0.01 caused the g33 to slightly drop and then increased with increasing BST composition to the optimum value of 20.93 Χ 10−3 V/mN at x = 0.05. The energy harvesting performance figure of merit can be calculated from the off-resonance figure of merit (FoM) as EquationEquation (4)
(4)
(4)
where d33 is the piezoelectric charge constant, and g33 is the piezoelectric voltage constant, respectively [Citation93–96]. As a result, the FoM value of undoped sample was 2.29 pm2/N. The addition of BST into BNT-BT increased the FOM value from 1.70 (x = 0.01) − 4.37 pm2/N (x = 0.04) with increasing of BST composition and dropped at x = 0.04 sample. Therefore, this showed that the figure of merit of the BNT-BT ceramics was significantly improved by the addition of BST. The best composition of x = 0.04 with the highest d33 of 277 pC/N and high g33 value of 15.79 × 10−3 Vm/N. The relation between g33 and FoM value is shown in . The comparison of the FoM value in this work with other previous reported [Citation44,Citation89,Citation94], [Citation97]; [Citation98] indicates that higher value.
4. Conclusions
Lead-free piezoelectric ceramics of (1-x)Bi0.5Na0.5TiO3-0.06BaTiO3-x(Ba0.7Sr0.3)TiO3 with x = 0.00, 0.01, 0.02, 0.03, 0.04 and 0.05 mol fraction were successfully prepared by using a conventional mixed-oxide technique. All compositions displayed a single phase of perovskite structure with larger tetragonality obtained at higher BST content. A slight grain size increase with more densification and heterogeneous microstructures were noticeable for the ceramics modified with BST additives. The highest piezoelectric coefficient (d33 = 277 pC/N) with good ferroelectric properties and energy harvesting properties were found for the ceramics with the addition of x = 0.04. This composition could be interesting for transducer, actuator or energy harvesting applications.
Acknowledgments
This research was financially supported by Center of Excellence in Materials Science and Materials Technology, the National Research University Project under Thailand’s Office of the Higher Education. Department of Physics and Materials Science, Faculty of Science, and Office of Research Administration, Chiang Mai University are also acknowledged. P. Wannasut would like to thank CMU Proactive Researcher, Chiang Mai University [grant number 819/2566].
Disclosure statement
No potential conflict of interest was reported by the author(s).
Additional information
Funding
References
- Moulson AJ, Herbert JM. Electroceramics: Materials Properties Applications. New York: Chapman and Hall Press; 1990. doi: 10.1002/0470867965
- Saito Y, Takao H, Tani T, et al. Lead free piezoelectric. Nature. 2004;432(7013):84–87. doi: 10.1038/nature03028
- Goel M. Recent developments in electroceramics: MEMS applications for energy and environment. Ceram Int. 2004;30(7):147–1154. doi: 10.1016/j.ceramint.2003.12.012
- Camargo J, Osinaga S, Febbo M, et al. Piezoelectric and structural properties of bismuth sodium potassium titanate lead-free ceramics for energy harvesting. J Mater Sci Mater Electron. 2021;32(14):19117–19125. doi: 10.1007/s10854-021-06430-3
- Wei HG, Wang H, Xia YJ, et al. An overview of lead-free piezoelectric materials and devices. J Mater Chem C. 2018;6(46):12446–67. doi: 10.1039/C8TC04515A
- Gao JH, Xue DZ, Liu WF, et al. Recent progress on BaTiO3-based piezoelectric ceramics for actuator applications. Actuators. 2017;6(3):24. doi: 10.3390/act6030024
- Zheng T, Zhang Y, Ke Q, et al. High-performance potassium sodium niobate piezoceramics for ultrasonic transducer. Nano Energy. 2020;70:104559. doi: 10.1016/j.nanoen.2020.104559
- Zang S, Xia R, Shrout TR. Leed free piezoelectric ceramics vs PZT. J Electroceram. 2007;19(4):251–257. doi: 10.1007/s10832-007-9056-z
- Panda PK. Review: environmental friendly lead-free piezoelectric materials. J Mater Sci. 2009;44(19):5049–5062. doi: 10.1007/s10853-009-3643-0
- Rodel J, Webber KG, Dittmer R, et al. Transferring lead-free-piezoelectric ceramics into application. J Eur Ceram Soc. 2015;35(6):1659–1681. doi: 10.1016/j.jeurceramsoc.2014.12.013
- Li W, Xu ZJ, Chu RQ, et al. Piezoelectric and Dielectric Properties of (Ba 1− x Ca x)(Ti 0.95 Zr 0.05)O 3 Lead-Free Ceramics. J Am Ceram Soc. 2010;93(10):2942–2944. doi: 10.1111/j.1551-2916.2010.03907.x
- Yoon MS, Khansur NH, Choi BK, et al. The effect of nano-sized BNBT on microstructure and dielectric/piezoelectric properties. Ceram Int. 2009;35(8):3027–3036. doi: 10.1016/j.ceramint.2009.04.016
- Wang K, Hussain A, Jo W, et al. Temperature-dependent properties of (Bi 1/2 Na 1/2) TiO 3 –(Bi 1/2 K 1/2) TiO 3 – SrTiO 3 Lead-Free Piezoceramics. J Am Ceram Soc. 2012;95(7):2241–2247. doi: 10.1111/j.1551-2916.2012.05162.x
- Lee KT, Park JS, Cho HJ, et al. Phase transition and electrical characteristics of Bi0.5(Na0.78K0.22)0.5TiO3–BiFeO3 lead-free piezoelectric ceramics. Ceram Int. 2015;41(8):10298–10303. doi: 10.1016/j.ceramint.2015.04.063
- Jaita P, Watcharapasorn A, Kumar N, et al. Lead- free (Ba0.70Sr0.30)TiO3-modified Bi0.5(Na0.80K0.20)0.5TiO3 ceramics with large electric fied-induced strain. J Am Ceram Soc. 2016;99:1615–1624. doi: 10.1111/jace.14136
- Wannasut P, Jaita P, Watcharapasorn A, et al. Microstructure and electrical properties of (1-x)Bi0.5(Na0.80K0.20)0.5TiO3-xLiNbO3 lead-free piezoelectric ceramics. IEEE Conference Proceedings and Special Issue in IEEE Transactions on Ultrasonics, Ferroelectrics, and Frequency Control; Singapore. 2015. p. 167–170.
- Bai W, Lui F, Li P, et al. Structure and electromechanical properties in Bi0.5Na0.5TiO3-based lead-free piezoceramics with calculated end-member Bi(Ni0.5Ti0.5)O3. J Eur Ceram Soc. 2015;35(13):3457–3466. doi: 10.1016/j.jeurceramsoc.2015.05.001
- Liu F, Wahyudi O, Li Y, et al. A new Bi0.5Na0.5TiO3 based lead-free piezoelectric system with calculated end-member Bi(Zn0.5Hf0.5)O3. Appl Phys. 2014;115(11):114101. doi: 10.1063/1.4868583
- Wang Q, Chen J, Fan L, et al. Preparation and electric properties of Bi0.5Na0.5TiO3-Bi(Mg0.5Ti0.5)O3 lead-free piezoceramics. J Am Ceram Soc. 2013;96:1171–1175. doi: 10.1111/jace.12147
- Zhao W, Ya J, Xin Y, et al. Fabrication of Na 0.5 Bi 0.5 TiO 3 –BaTiO 3 -textured ceramics templated by plate-like Na 0.5 Bi 0.5 TiO 3 particles. J Am Ceram Soc. 2009;92(7):1607. doi: 10.1111/j.1551-2916.2009.03043.x
- Yan YK, Zhou HP, Zhao W, et al. Fabrication and electrical properties of textured Na1/2Bi1/2TiO3-BaTiO3 ceramics by reactive-templated grain growth. J Electroceram. 2008;21(1–4):246. doi: 10.1007/s10832-007-9140-4
- Zhang QH, Zhang YY, Wang FF, et al. Growth and electric properties of 0.96Na0.5Bi0.5TiO3–0.04BaTiO3 single crystal. J Cryst Growth. 2010;312(3):457. doi: 10.1016/j.jcrysgro.2009.11.006
- Zhang QH, Zhang YY, Wang FF, et al. Enhanced piezoelectric and ferroelectric properties in Mn-doped Na0.5Bi0.5TiO3–BaTiO3 single crystals. Appl Phys Lett. 2009;95(10):102904. doi: 10.1063/1.3222942
- Ben WVE, Damjanovic D, Klein N, et al. Structural complexity of (Na0.5Bi0.5)TiO3-BaTiO3 as revealed by Raman spectroscopy. Phys Rev B. 2010;82(10):104112–104117. doi: 10.1103/PhysRevB.82.104112
- Machado R, Ochoa DA, Santos VBD, et al. High stability of properties in morphotropic phase boundary Bi0.5Na0.5TiO3-BaTiO3 piezoceramics. Mater Lett. 2016;183:73–76. doi: 10.1016/j.matlet.2016.07.045
- Supriya S. Synthesis mechanisms and effects of BaTiO3 doping on the optical. J Solid State Chem. 2022;308:122940. doi:10.1016/j.jssc.2022.122940
- Supriya S. A review on lead-free-Bi0.5Na0.5TiO3 based ceramics and films: dielectric, piezoelectric, ferroelectric and energy storage performance. J Inorg Organomet Polym. 2022;32(10):3659–3676. doi: 10.1007/s10904-022-02418-6
- Supriya S. Highly tunable multifunctional rare earth based Bi0.5-xCexNa0.5TiO3 perovskites via site selective doping engineering. Mater Chem Phys. 2022;287:126233. doi: 10.1016/j.matchemphys.2022.126233
- Supriya S. A critical review on crystal structure mechanisms, microstructural and electrical performances of Bi0.5Na0.5TiO3—SrTiO3 perovskites. J Electroceramics. 2022;49(2):94–108. doi: 10.1007/s10832-022-00295-6
- Ren X, Yin H, Tang Y, et al. The large electro-strain in BNKT-BST-100xTa lead-free ceramics. Ceram Int. 2020;46(2):1876–1882. doi: 10.1016/j.ceramint.2019.09.164
- Cheng BL, Su B, Holmes JE, et al. Dielectric and mechanical losses in (Ba,Sr)TiO3 systems. J Electroceram. 2002;9(1):17–23. doi: 10.1023/A:1021633917071
- Ren X, Fan H, Zhao Y, et al. Flexible lead-free BiFeO3/PDMS-based nanogenerator as piezoelectric energy harvester. ACS Appl Mater Interfaces. 2016;8(39):26190–26197. doi: 10.1021/acsami.6b04497
- Deol RS, Batra N, Rai P, et al. A lead-free flexible energy harvesting device microsyst. Microsyst Technol. 2022;28(9):2061–2070. doi: 10.1007/s00542-022-05345-1
- Alam MM, SK G, Sultana A, et al. Lead-free ZnSnO 3 /MWCNTs-based self-poled flexible hybrid nanogenerator for piezoelectric power generation. Nanotechnology. 2015;26(16):165403. doi: 10.1088/0957-4484/26/16/165403
- Yan M, Liu S, Xu Q, et al. Enhanced energy harvesting performance in lead-free multi-layer piezoelectric composites with a highly aligned pore structure. Nano Energy. 2023;106:108096. doi: 10.1016/j.nanoen.2022.108096
- Rout D, Moon KS, Kang SJL, et al. Dielectric and Raman scattering studies of phase transitions in the (100−x)Na0.5Bi0.5TiO3–xSrtio3 system. J Appl Phys. 2010;108(8):084102. doi: 10.1063/1.3490781
- Rai R, Coondoo I, Lopes RP, et al. Development of lead-free materials for piezoelectric energy harvesting. Mater Res Soc Symp Proc. 2011;1325:105–110. doi: 10.1557/opl.2011.847
- Ye J, Ding G, Wu X, et al. Development and performance research of PSN-PZT piezoelectric ceramics based on road vibration energy harvesting technology. Mater Today Commun. 2023;34:105135. doi: 10.1016/j.mtcomm.2022.105135
- Song J, Qi L, Wang Y. A dual-function system integrating kinetic energy harvesting and passenger sensing for urban subway. Int J Hydrogen Energy. 2023;48(100):40053–40070. In Press. doi: 10.1016/j.ijhydene.2023.09.172
- Wang X. Piezoelectric nanogenerators—Harvesting ambient mechanical energy at the nanometer scale. Nano Energy. 2012;1(1):13–24. doi: 10.1016/j.nanoen.2011.09.001
- Rani GM, Wu CM, Motora KG. Acoustic-electric conversion and triboelectric properties of nature-driven CF-CNT based triboelectric nanogenerator for mechanical and sound energy harvesting. Nano Energy. 2023;108:108211. doi: 10.1016/j.nanoen.2023.108211
- Rani GM, Wu CM, Motora KG. Waste-to-energy: utilization of recycled waste materials to fabricate triboelectric nanogenerator for mechanical energy harvesting. J Clean Prod. 2022;363:132532. doi: 10.1016/j.jclepro.2022.132532
- Wang S, Wang C, Yu G, et al. Development and performance of a piezoelectric energy conversion structure applied in pavement. Energy Convers Manag. 2020;207:112571. doi: 10.1016/j.enconman.2020.112571
- Jaita P, Manotham S, Rujijanagul G. Influence of Al2O3 nanoparticle doping on depolarization temperature, and electrical and energy harvesting properties of lead-free 0.94(Bi0.5Na0.5)TiO3-0.06BaTiO3 ceramics. RSC Adv. 2020;10(53):32078–32087. doi: 10.1039/D0RA04866F
- Yao FZ, Yu Q, Wang K, et al. Ferroelectric domain morphology and temperature-dependent piezoelectricity of (K,Na,Li)(Nb,Ta,Sb)O3 lead-free piezoceramics. RSC Adv. 2014;4(39):20062–20068. doi: 10.1039/C4RA01697A
- Coondoo I, Panwar N, Amorın H, et al. Enhanced piezoelectric properties of praseodymium-modified lead-free (Ba 0.85 Ca 0.15)(ti 0.90 Zr 0.10)O 3 ceramics. J Am Ceram Soc. 2015;98(10):3127–3135. doi: 10.1111/jace.13713
- Ngo NCT, Sugiyama H, Sodige BAK, et al. Enhancing low-temperature energy harvesting by lead-free ferroelectric Ba(Zr0.1Ti0.9)O3. J Asian Ceram Soc. 2023;106(1):201–212. doi: 10.1111/jace.18663
- Bijalwan V, Erhart J, Spotz Z, et al. Composition driven (Ba,Ca)(Zr,Ti)O3 lead-free ceramics with large quality factor and energy harvesting characteristics. J Am Ceram Soc. 2021;104(2):1088–1101. doi: 10.1111/jace.17497
- Jaita P, Saenkamad K, Rujijanagul G. Improvements in piezoelectric and energy harvesting properties with a slight change in depolarization temperature in modified BNKT ceramics by a simple technique. RSC Adv. 2023;13(6):3743. doi: 10.1039/D2RA07587C
- Wannasut P, Jaiban P, Jaita P, et al. Improvement of electrical and energy harvesting properties of new lead-free BST modified 0.995BNKT-0.005LN ceramics. J Asian Ceram Soc. 2023;11(1):88–97. doi: 10.1080/21870764.2022.2156668
- Powder Diffraction File No. 00-036-0340. Newton Square, PA: International Centre for Diffraction Data; 2000.
- Powder Diffraction File No. 00-005-0626. Newton Square, PA: International Centre for Diffraction Data; 2000.
- Powder Diffraction File No. 01-089-0274. Newton Square, PA: International Centre for Diffraction Data;, 2000.
- Takenaka T, Maruyama K, Sakata K. (Bi1/2Na1/2)TiO3-BaTiO3 system for lead-free piezoelectric ceramic. Jpn J Appl Phys. 1991;30:2236–2239. doi: 10.1143/JJAP.30.2236
- Chandrasekhar M, Kumar P. Synthesis and characterizations of BNT–BT and BNT–BT–KNN ceramics for actuator and energy storage applications. Ceram Int. 2015;4(4):5574–5580. doi: 10.1016/j.ceramint.2014.12.136
- Jaita P, Watcharapasorn A, Cann DP. Dielectric, ferroelectric and electric field-induced strain behavior of Ba(Ti0.90Sn0.10)O3-modified Bi0.5(Na0.80K0.20)0.5TiO3 lead-free piezoelectrics. J Alloys Compd. 2014;596:98–106. doi: 10.1016/j.jallcom.2014.01.183
- Supriya S. Crystal structure engineered non-toxic Bi0.5Na0.5TiO3 based thin films- fabrication process, enhanced electrical performance, challenges and recent reports. J Inorg Organomet Polym. 2023;33(10):3013–3026. doi: 10.1007/s10904-023-02765-y
- Chen ZW, Hu JQ. Piezoelectric and dielectric properties of Bi 0 ˙ 5 (Na 0 ˙ 84 K 0 ˙ 16) 0 ˙ 5 TiO 3 –Ba(Zr 0 ˙ 04 Ti 0 ˙ 96)O 3 lead free piezoelectric ceramics. Adv Appl Ceram. 2008;107(4):222–226. doi: 10.1179/174367608X263403
- Shannon RD. Revised effective ionic radii and systematic studies of interatomic distances in halides and chalcogenides. Acta Cryst A. 1976;32(5):751–767. doi: 10.1107/S0567739476001551
- Rafique R, Tonny KN, Sharmin A, et al. Study on the effect of varying film thickness on the transparent conductive nature of aluminum doped zinc oxide deposited by dip coating. Mater Focus. 2018;7(5):1–7. doi: 10.1166/mat.2018.1572
- Markevich N, Gertner I. Comparison among methods for calculating FWHM. Nucl Instrum Methods Phys Res A. 1989;283(1):72–77. doi: 10.1016/0168-9002(89)91258-8
- Cullity BD. Elements of X-ray diffraction. Philippines: Addison-Wesley Publishing; 1978.
- Hosseinmardi A, Shojaee N, Rad MK, et al. A study on the photoluminescence properties of electrospray deposited amorphous and crystalline nanostructured ZnO thin films. Ceram Int. 2012;38(3):1975–1980. doi: 10.1016/j.ceramint.2011.10.031
- Lee MJ, Lee TI, Lim J, et al. Effect of the deposition temperature and a hydrogen post-annealing treatment on the structural, electrical, and optical properties of ga-doped ZnO films, electron. Mater Lett. 2009;5(3):127–133. doi: 10.3365/eml.2009.09.127
- Lele S, Anantharaman TR. Influence of crystallite shape on particle size broadening of debye-scherrer reflections. Proc Indian Acad Sci. 1966;64(5):261–274. doi: 10.1007/BF03047543
- Zaier A, Meftah A, Jaber AY, et al. Annealing effects on the structural, electrical and optical properties of ZnO thin films prepared by thermal evaporation technique. J King Saud Univ Sci. 2015;27(4):356–360. doi: 10.1016/j.jksus.2015.04.007
- Co ND, Cuong LV, Tu BD, et al. Effect of crystallization temperature on energy-storage density and efficiency of lead-free Bi0.5(Na0.8K0.2)0.5TiO3 thin films prepared by sol–gel method. J Sci Adv Mater Devices. 2019;4(3):370–375. doi: 10.1016/j.jsamd.2019.04.008
- Gahtar A, Benali A, Benramache S, et al. Effect of annealing time on the structural, morphological, optical and electrical properties of NiS thin films. Chalcogenide Lett. 2022;19(2):103–116. doi: 10.15251/CL.2022.192.103
- Razak KA, Yip CJ, Sreekantan S. Synthesis of (Bi0.5Na0.5)TiO3 (BNT) and pr doped BNT using the soft combustion technique and its properties. J Alloys Compd. 2011;509(6):2936–2941. doi: 10.1016/j.jallcom.2010.11.163
- Bokuniaeva AO, Vorokh AS. Estimation of particle size using the Debye equation and the Scherrer formula for polyphasic TiO2 powder. J Phys Conf Ser. 2019;1410(1):012057. doi: 10.1088/1742-6596/1410/1/012057
- Badapanda T, Sahoo S, Nayak P. Dielectric, ferroelectric and piezoelectric study of BNT-BT solid solutions around the MPB region. IOP Conf Ser Mater Sci Eng. 2017;178:012032. doi: 10.1088/1757-899X/178/1/012032
- Difeo M, Osinaga S, Febbo M, et al. Influence of the (Bi0.5Na0.5)TiO3–BaTiO3 lead-free piezoceramic geometries on the power generation of energy harvesting devices. Ceram Int. 2021;47(8):10696–10704. doi: 10.1016/j.ceramint.2020.12.184
- Prasertpalichat S, Siritanon T, Nuntawong N, et al. Structural characterization of A-site nonstoichiometric (1 − x)Bi0.5Na0.5TiO3–xBatio3 ceramics. J Mater Sci. 2019;54(2):1162–1170. doi: 10.1007/s10853-018-2939-3
- Wang J, Zhou C, Li Q, et al. Dual relaxation behaviors and large electrostrictive properties of Bi0.5Na0.5TiO3–Sr0.85Bi0.1TiO3 ceramics. J Mater Sci. 2018;53(12):8844–8854. doi: 10.1007/s10853-018-2186-7
- Chen PY, Chen CS, Tu CS. Effects of texture on microstructure, raman vibration, and ferroelectric properties in 92.5%(Bi0.5Na0.5)TiO3–7.5%BaTiO3 ceramics. J Eur Ceram. 2016;36(7):1613–1622. doi: 10.1016/j.jeurceramsoc.2016.01.038
- Nesterović A, Vukmirović J, Stijepović I, et al. Structure and dielectric properties of (1-x)Bi0.5Na0.5TiO3–xBaTiO3 piezoceramics prepared using hydrothermally synthesized powders. R Soc Open Sci. 2021;8(7):202365. doi: 10.1098/rsos.202365
- Selvamani R, Singh G, Sathe V, et al. Dielectric, structural and Raman studies on (Na 0.5 Bi 0.5 TiO 3) (1 − x) (BiCrO 3) x ceramic. J Phys Condens Matter. 2011;23(5):055901. doi: 10.1088/0953-8984/23/5/055901
- Jaita P, Jarupoom P. Enhanced electric field-induced strain and electrostrictive response of lead-free BaTiO3-modified Bi0.5(Na0.80K0.20)0.5TiO3 piezoelectric ceramics. J Asian Ceram Soc. 2021;9(3):975–987. doi: 10.1080/21870764.2021.1930953
- Chaisan W, Yimnirun R, Ananta S. Two-stage sintering of barium titanate ceramics and resulting characteristics. Ferroelectrics. 2007;346(1):84–92. doi: 10.1080/00150190601180380
- Khamman O, Watcharapasorn A, Pengpat K. Fin grained bismuth sodium titanate ceramics prepared via vibro-milling method. J Mater Sci. 2006;41(16):5391–5394. doi: 10.1007/s10853-006-0405-0
- Chen IW, Wang XH. Sintering dense nanocrystalline ceramics without final-stage grain growth. Nature. 2000;404(6774):168–171. doi: 10.1038/35004548
- Rai R, Coondoo I, Rani R. Impedance spectroscopy and piezoresponse force microscopy analysis of lead-free (1 − x) K0.5Na0.5NbO3 − xLiNbO3 ceramics. Curr Appl Phys. 2013;13(2):430–440. doi: 10.1016/j.cap.2012.09.009
- Chiang YM, Birnie DP, WD K. Physical ceramics: principles for ceramics science and engineering. New York: John Wiley & Sons, Inc; 1997.
- Pham KN, Lee HB, Han HS, et al. Dielectric, ferroelectric, and piezoelectric properties of Nb-substituted Bi1/2(Na0.82K0.18)1/2TiO3 lead-free ceramics. Phys Soc. 2012;60:207–211. doi: 10.3938/jkps.60.207
- Hussain A, Zamman A, Iqbal Y. Dielectric, ferroelectric and field induced strain properties of Nb-modified Pb-free 0.99Bi0.5(Na0.82K0.18)TiO3-0.01LiSbO3 ceramics. J Alloy Compd. 2013;574:320–324. doi: 10.1016/j.jallcom.2013.05.140
- Supriya S. Research progress, doping strategies and dielectric-ferroelectric anomalies of rare earth-based Bi0.5Na0.5TiO3 perovskites. J Rare Earths. 2023. doi: 10.1016/j.jre.2023.10.009
- Xu B, Paillard C, Dkhil B, et al. Pinched hysteresis loop in defect-free ferroelectric materials. Phys Rev B. 2016;94(14):140101(R. doi: 10.1103/PhysRevB.94.140101
- Zuo R, Ye C, Fang X, et al. Tantalum doped 0.94Bi0.5Na0.5TiO3–0.06BaTiO3 piezoelectric ceramics. J Eur Ceram. 2008;28(4):871–877. doi: 10.1016/j.jeurceramsoc.2007.08.011
- Hao J, Shen B, Zhai J, et al. Switching of morphotropic phase boundary and large strain response in lead-free ternary (Bi0.5Na0.5)TiO3–(K0.5Bi0.5)TiO3–(K0.5Na0.5)NbO3 system. J Appl Phys. 2013;113(11):114106. doi: 10.1063/1.4795511
- Haertling GH. Ferroelectric ceramics: history and technology. J Am Ceram Soc. 1999;82(4):797–818. doi: 10.1111/j.1151-2916.1999.tb01840.x
- Sun Y, Chang Y, Wu J, et al. Ultrahigh energy harvesting properties in textured lead-free piezoelectric composites. J Mater Chem A. 2019;7(8):3603–3611. doi: 10.1039/C8TA10312G
- De U, Sahu KR, De A. Ferroelectric materials for high temperature piezoelectric applications. Solid State Phenom. 2015;232:235–278. doi: 10.4028/www.scientific.net/SSP.232.235
- Leng H, Wang Y, Yan Y, et al. Water quenched and acceptor-doped textured piezoelectric ceramics for off-resonance and on-resonance devices. Small. 2023;19(1):2204454. doi: 10.1002/smll.202204454
- Shin DJ, Kang WS, Koh JH, et al. Comparative study between the pillar‐ and bulk‐type multilayer structures for piezoelectric energy harvesters. Phys Status Solidi A. 2014;211(8):1812–1817. doi: 10.1002/pssa.201330505
- Choi YJ, Yoo MJ, Kang HW, et al. Dielectric and piezoelectric properties of ceramic-polymer composites with 0–3 connectivity type. J Electroceram. 2013;30(1–2):30–35. doi: 10.1007/s10832-012-9706-7
- Mahmud I, Ur SC, Man SY. Effects of Fe2O3 addition on the piezoelectric and the dielectric properties of 0.99Pb(Zr0.53Ti0.47)O3-0.01Bi(Y1-xFex)O3 ceramics for energy-harvesting devices. J Korean Phys Soc. 2014;65(2):133–144. doi: 10.3938/jkps.65.133
- Kwon YH, Shin DJ, Koh JH. (1 − x)(Bi,Na)TiO3−x (Ba,Sr)TiO3 lead-free piezoelectric ceramics for piezoelectric energy harvesting. J Korean Phys Soc. 2015;66(7):1067–1071. doi: 10.3938/jkps.66.1067
- Gowdhaman P, Annamalai V, Thakur OP. Piezo, ferro and dielectric properties of ceramic-polymer composites of 0-3 connectivity. Ferroelectrics. 2016;493(1):120–129. doi: 10.1080/00150193.2016.1134028