ABSTRACT
Digital Light Processing (DLP) technology is a highly effective molding method for creating complex structural parts. However, the low solid content of SiC stereolithography slurry has been found to diminish the mechanical properties of the ceramic, regardless of whether it is sintered by Reactive Melt Infiltration (RMI) or Precursor Infiltration and Pyrolysis (PIP). This poses a significant challenge in improving the mechanical properties of SiC ceramics manufactured through DLP additive manufacturing. In response to this challenge, a novel method of RMI combined with infiltration is proposed in this study. The process involves the filling of the pores of the green body with pyrolytic carbon from PR or pyrolytic SiC from LHBPCS through circular infiltration, effectively increasing the content of SiC in the ceramics after reaction. Notably, the elastic modulus of RM-P2 and RM-L2 samples increased by 37.39% and 33.91%, respectively, demonstrating the potential of this method for preparing ceramics with high SiC content through stereolithography additive manufacturing. This innovative approach holds promise for overcoming the limitations previously associated with SiC ceramics produced through DLP additive manufacturing, opening up new possibilities for the development of high-performance SiC-based components.
1. Introduction
The exceptional properties of Silicon Carbide (SiC) ceramics, such as high strength, hardness, modulus, and radiation resistance [Citation1–4], have made them highly sought after in various industries, including automotive [Citation5], aerospace [Citation6], chemical, and nuclear sectors [Citation7–9]. These industries benefit from SiC ceramics’ durability and performance in extreme conditions. However, the very characteristics that make SiC ceramics so valuable also present significant challenges in their fabrication, particularly in machining or polishing. The material’s high hardness complicates traditional manufacturing processes, necessitating the development of innovative methods to efficiently shape and finish SiC components, especially those with large sizes or complex geometries [Citation10].
3D-printing technology emerges as a powerful solution to these challenges, offering the capability to rapidly fabricate complex structures through a layer-by-layer approach [Citation11]. This technology spans several techniques suitable for SiC ceramics, including Stereolithography (SLA/DLP/LCD) [Citation12], Selective Laser Sintering (SLS) [Citation13], Direct Ink Writing (DIW) [Citation14], and Laminated Object Manufacturing (LOM) [Citation15]. Among these, stereolithography stands out for its precision and accuracy, making it particularly promising for the production of SiC ceramics [Citation12].
Stereolithography-based additive manufacturing has already proven successful in creating polymer [Citation16], zirconia [Citation17], and alumina ceramics [Citation18] with applications in commercial products. This success lays the groundwork for expanding the use of stereolithography in SiC ceramic production. By overcoming the limitations associated with traditional machining and polishing through 3D printing, there is a significant opportunity to enhance the manufacturing process of SiC ceramics. This advancement could lead to more efficient production of high-quality SiC components, further expanding their application in critical and high-performance industries.
Due to the high optical absorbance in UV wavelength and low fluidity of SiC powder, it is difficult to prepare slurry with high solid content and viscosity meeting printing requirements [Citation19]. Ding et al. [Citation19] systematically studied the effects of resin monomer, dispersant, particle size, solid content and milling time on stereolithography slurry, and finally successfully printed 40 vol% SiC green body. The stereolithography slurry with low solid content will result in low density and high porosity of the green body printed. However, the sintering methods commonly used for 3D-printing SiC ceramics, such as Reactive Melt Infiltration (RMI) and Precursor Infiltration and Pyrolysis (PIP), are difficult to obtain high-performance SiC ceramics. He et al. [Citation20] densified 30 vol, 35 vol% and 40 vol% green body by PIP, and the relative density of 35 vol% after PIP treatment was the highest, reaching 84.8%. Nevertheless, its density is still low and its flexural strength is only 204.6 MPa. Zhang et al. [Citation11] used sodium alginate as a binder to prepare SiC ceramic composites through extrusion freeforming 3D-printing technology combined liquid silicon infiltration process. The SiC ceramic composites exhibited high flexural strengths of 238 MPa and 300 MPa with solid contents of 32.38vol% and 34.68vol%, respectively. Despite their high density, the low solid content in the green body results in an excess of free Si, which can adversely impact the ceramic’s high-temperature performance. While high-density ceramic parts can be achieved through stereolithography combined with RMI, the low-density green body can lead to significant residual Si in the ceramics, thereby compromising their mechanical properties. Additionally, obtaining high-density ceramic parts via stereolithography combined with PIP is challenging, as repeated cyclic infiltration and pyrolysis can create numerous closed pores that are difficult to eliminate. Zou et al. [Citation21] infiltrated green body prepared by SLS with PR and PR containing 30% nano carbon black, and densified them through RMI. The sample with PFnanoC infiltration’s flexural strength increased observably (22.1%). P.S. Grinchuk et al. [Citation22] prepared a C/SiC preform through cyclic PR infiltration and pyrolysis, followed by infiltration with Si melt. With an increase of impregnation number up to four, SiC fraction in the ceramics enlarges to 93 vol%. Infiltration before sintering can effectively increase the content of SiC in sintered ceramics.
In response to the limitations posed by low density and poor mechanical properties in the final products of reaction-sintered ceramics, our innovative method offers a solution to significantly enhance the SiC volume content. By implementing multiple impregnations to improve the residual carbon in the raw billets, we have successfully achieved a substantial increase in the SiC content in the sintered ceramics. The utilization of the LHBPCS recirculating impregnation method, followed by densification through reactive sintering, has yielded impressive results. Our printed samples, boasting a solids content of 40, underwent 1, 2, and 3 impregnations, with the latter resulting in the best performance. The reactive sintering process facilitated the reaction of pyrolytic carbon with Si and pyrolytic SiC, leading to a remarkable 37.39% increase in the modulus of elasticity. This breakthrough method not only addresses the drawbacks and challenges previously encountered but also paves the way for the production of reaction-sintered ceramics with superior mechanical properties and higher SiC volume content.
2. Experimental
2.1. Preparation of SiC ceramic slurry
1,6-Hexanediol diacrylate (HDDA, purity: 90%, stabilized with MEHQ), Trimethylolpropane triacrylate (TMPTA, purity: 85%, Contains 600 ppm MEHQ stabilizer), these two monomers were mixed in a volume ratio of 1:1. Phenylbis (2,4,6-trimethylbenzoyl) phosphine oxide (BAPO, purity: 97%) was used as photoinitiator for high activity, and the addition amount was 2 wt%. KOS110 (Guangzhou Kang’ou Shuang Trade Co., Ltd) was used as Dispersant and lubricant, and the addition amount was 4 wt%. They were mixed evenly by mechanical stirring, and then the photosensitive resin was obtained. 40 vol% silicon carbide powder (SiC, average particle size D50 = 30 μm) was added to the photosensitive resin, photosensitive resin and SiC powder evenly mixed through mechanical stirring.
2.2. Stereolithography
For the DLP printing process, 3D models for printing were used to design by SolidWorks software firstly. Then, models which were sliced in layers with a layer thickness of 75 μm were exported to STL format files. STL format model files were imported into a DLP printer (Beijing TenDimensions Technology Co., Ltd. Autoceram ceramic 3D printer, wavelength 405 nm). The photosensitive slurry was poured into the printing tank, and the exposure energy and exposure time were set as 12 mw/cm2 and 10s, respectively. The DLP printer started to print green body according to the sliced pattern from bottom to top. After every layer printing was finished, the platform moved upward and the scraper scraped the slurry flatly to print next layer. Repeated the above process continuously until the end of printing. After the entire body was obtained, it was taken out from the substrate and ultrasonic cleaned in deionized water for 60 s to rinse off the residual slurry on the surface. shows the schematic for the preparation route of SiC ceramics.
2.3. Cyclic infiltration and sintering
Green body was heated up to 900°C under vacuum atmosphere for half an hour to pyrolyze the binder, then debonded body was immersed in a container with phenolic resin (PR, PR: Anhydrous ethanol = 1:1, char yield is 42.63 wt%). The container was put into the liquid-phase densification equipment, it firstly was vacuumized to −0.1MPa and maintain vacuum for 20 min, then it was inflated to 2Mpa and maintain pressure for 30 min. After the infiltration process was over, infiltrated body was put into an oven at 150°C for 1 h to cure PR. PR infiltrated once body was heated up to 900°C under vacuum atmosphere for half an hour to pyrolyze PR. PR infiltrated and pyrolyzed once body is called “P1”. P1 was infiltrated with PR and pyrolyzed again, and PR infiltrated and pyrolyzed twice is called “P2”. Similarly, PR infiltrated and pyrolyzed thrice is called “P3”. P1, P2, and P3 were paved with a certain amount of silicon particles to reactive sintering.
Debonded body was immersed in a container with liquid hyperbranched Polycarbosilane (LHBPCS, Ningbo institute of Materials Technology & Engineering, ceramic yield at 1400°C is 73.25 wt%). The container was put into the liquid-phase densification equipment, it firstly was vacuumized to −0.1 Mpa and maintain vacuum for 20 min, then it was inflated to 2 Mpa and maintain pressure for 30 min. After the infiltration process was over, infiltrated body was putted into an oven at 200°C for 1 h to cure LHBPCS. LHBPCS infiltrated once body was heated up to 1200°C under argon atmosphere for half an hour to pyrolyze LHBPCS. LHBPCS infiltrated and pyrolyzed once body is called “L1”. L1 was infiltrated with LHBPCS and pyrolyzed again, and LHBPCS infiltrated and pyrolyzed twice is called “L2”. Similarly, LHBPCS infiltrated and pyrolyzed thrice is called “L3”. L1, L2, and L3 were paved with a certain amount of silicon particles to reactive sintering.
2.4. Characterization
Microstructure and morphology of samples were investigated through scanning electron microscopy (SEM, Phenom ProX, The Netherlands). The crystalline phase composition and Oxygen content of the reduced body were analyzed by X-ray diffraction (XRD, D8 ADVANCE, Germany Bruker) and energy-dispersive spectrum analysis (EDS, Phenom ProX, Netherlands). Moreover, the mechanical properties of rectangular block sample were determined by three-point bending method with a span of 30 mm and a crosshead speed of 0.5 mm/min and Single Edge Notched Beam method with a span of 20 mm and the loading rate is 0.05 mm/min through Instron universal testing machine (Instron-5566, UK INSTRON). The sample size for testing flexural strength and elastic modulus is 4 × 3 × 36 mm. The sample size for testing fracture toughness is 5 × 2.5 × 20, the notch radius of the sample is 0.15 mm and the notch depth of the sample is 2.5 mm. The density of sample was measured by Archimedes drainage method.
3. Results and discussion
3.1. Characterization of SiC preform
The green body printed has a high density because the particles are tightly bonded under the action of cured resin. Debonded body produces more pores which is due to the low solid content in the printing paste in the process of pyrolysis of resin [Citation19]. The pore size and pore volume can be effectively reduced by impregnating PR and LHBPCS [Citation21], and the SiC content of the sintered body can be improved by filling the holes with pyrolytic carbon from PR to generate more SiC in the process of RMI [Citation22] and filling with derived SiC from LHBPCS [Citation23]. Pore size distribution of samples infiltrated by PR or LHBPCS with different times is shown in ). As shown in the figure, the pore size distribution of debonded body is mainly about 5 μm. The pore size distribution and pore volume of samples infiltrated by PR or LHBPCS with different times decreases with the increase of infiltration times, which is mainly due to the attachment of pyrolytic carbon or SiC derived from LHBPCS to particles in debonded body ). However, some pore sizes of L1, L2, L3 are larger than the original pore size. It is believed that this is due to the rapid heating rate and gas overflow during the pyrolysis of LHBPCS [Citation24]. There are no such phenomenon in P1, P2, and P3.
3.2. Microstructure and phase composition of SiC ceramic composites
shows the XRD pattern of RM-Ref, RM-P1, RM-P2, RM-P3, RM-L1, RM-L2, and RM-L3. The phase corresponding to each peak has been marked according to the standard XRD card. With the increase of infiltration times, the peak intensity of Si decreases. The content of free Si can be effectively reduced by cyclic infiltration, whether it is infiltrated with PR or LHBPCS. The reduction of free Si content can also be clearly observed in the microstructure (). The reduction of free Si is attributed to the fact that PR or LHBPCS enters the pores of the debonded body and fills the pore structure with pyrolytic products, which reduces the pore size and the volume of pore, thus reducing the amount of molten silicon entering the debonded body during the process of reaction sintering. In addition, the peak intensity of β-SiC at 35.7° increases with the increase of impregnation times, which is due to the fact that SiC generated by the reaction of pyrolytic C with Si and the SiC produced from LHBPCS pyrolysis are both β-SiC phase [Citation25].
Figure 3. XRD pattern of RM-Ref, RM-P1, RM-P2, RM-P3 (a) and RM-Ref, RM-L1, RM-L2, and RM-L3 (b); the position of the most intensive peak of β-SiC at 35.7° (c) & (d).
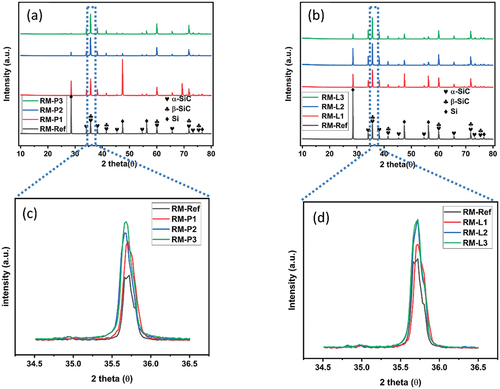
Figure 4. The flexural strength and elastic modulus change diagram of RM-Ref, RM-P1, RM-P2, RM-P3 (a) and RM-Ref, RM-L1, RM-L2, and RM-L3 (b); the density and apparent porosity change diagram of RM-Ref, RM-P1, RM-P2, RM-P3 (c) and RM-Ref, RM-L1, RM-L2, and RM-L3 (d).
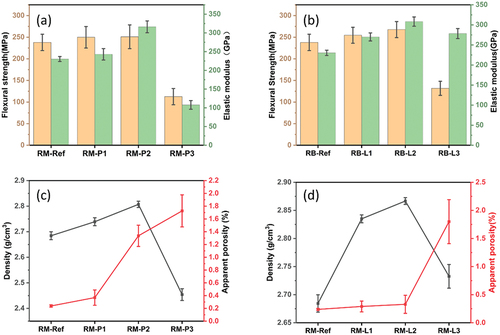
L1, L2, L3, P1, P2, and P3 were densified by RMI. The flexural strength and elastic modulus of sintered samples are shown in . It can be clearly seen from figure that the flexural strength of sintered ceramic infiltrated by PR or LHBPCS increases slightly with the increase of infiltration times when the number of infiltration times is less than or equal to two. However, when the number of infiltration times is three, the flexural strength of RM-P3 and RM-L3 are only 112.533 ± 18.8528 MPa and 131.752 ± 16.297 MPa; the elastic modulus of sintered ceramic infiltrated by PR or LHBPCS reaches the maximum value when the number of infiltration times is two, which is 37.39% and 33.91%, respectively, higher than that of RM-Ref. When the number of infiltration times is three, the elastic modulus of RM-L3 still remains at 278.34 ± 12.5 GPa. However, the elastic modulus of RM-P3 is only 107.669 ± 11.505 Gpa.
The microstructure of RM-Ref, RM-P1, RM-P2, RM-P3, RM-L1, RM-L2, and RM-L3 is shown in . It can be seen from the figure that the main components in ceramics are SiC (dark color) and Si (light color). The content of SiC can be effectively increased by cyclic infiltration of PR or LHBPCS, which is the reason why the density increases with the increase of infiltration times when the impregnation times are less than or equal to two . In addition, RM-P2, RM-L2, and RM-L3 contain a little free C, while RM-P3 contains a large amount of free carbon. This is because when pyrolysis C from PR reacts with Si, SiC generated at the interface between Si and C will hinder the continuation of the reaction [Citation26]. Similarly, a small amount of free C will also be generated during the pyrolysis of LHBPCS [Citation27], but a large amount of free C will not be left in the ceramic like RM-P3. In addition to the presence of free C, a large number of closed pores can be observed with the increase of infiltration times. The appearance of closed pores in RM-P1, RM-P2, and RM-P3 is mainly due to the volume expansion caused by the reaction of C and Si [Citation12], thus blocking the infiltration channel of molten Si. For RM-L1, RM-L2, and RM-L3, it is mainly due to the closed pores formed during the Precursor Infiltration and Pyrolysis [Citation20], so that the ceramic can not be densified. For RM-L3 and RM-P3, the appearance of closed pores or a large amount of free carbon lead to low density of ceramics and the porosity of the ceramics is high. The apparent porosity of them is 1.8 ± 0.391% and 1.726 ± 0.25%, respectively . The existence of closed pores indicates that the ceramic is not dense, which is why the content of SiC increases but the flexural strength does not improve significantly. However, the elastic modulus reflects the rigidity of materials. The increase of SiC content can significantly improve the rigidity of ceramic, although the appearance of a few closed pores. For RM-P3 and RM-L3, the elastic modulus of them decreases because a large amount of free C and closed pores lead to the reduction of SiC content in ceramics. The density of RM-L3 and RM-P3 is only 2.732 ± 0.021 g/cm3 and 2.454 ± 0.023 g/cm3, respectively. Especially for RM-P3, it can be seen from that a large amount of free C blocks the channel.
4. Conclusion
In conclusion, a cutting-edge method has been developed for creating silicon carbide (SiC) ceramic composites with heightened SiC concentrations, utilizing Digital Light Processing (DLP) in conjunction with infiltration and Reaction Molding Infiltration (RMI). This study explores the effects of varying the number of infiltration cycles using polymer resin (PR) or liquid hyperbranched polycarbosilane (LHBPCS) on both the microstructure and mechanical properties of the precursor form and the resulting reaction-bonded SiC (RB-SiC) ceramic composites. It has been shown that an optimal number of infiltrations with PR or LHBPCS can significantly enhance the SiC content within RB-SiC ceramics. Notably, a dual infiltration process produces RM-P2 and RM-L2 composites with superior flexural strength and elastic modulus, attributed to increased SiC content and a reduction in defects such as closed pores and residual carbon. The pyrolytic carbon from PR reacts with silicon, while the SiC produced from LHBPCS effectively boosts the SiC content in the ceramics. This manufacturing approach shows great promise for improving the mechanical properties of RB-SiC ceramic composites.
Disclosure statement
No potential conflict of interest was reported by the author(s).
Additional information
Funding
References
- Liu JW, Zhou XB, Tatarko P, et al. Fabrication, microstructure, and properties of SiC/Al4SiC4 multiphase ceramics via an in-situ formed liquid phase sintering. J Adv Ceram. 2020 Apr;9(2):193–203. doi: 10.1007/s40145-020-0359-8
- Khodaei M, Yaghobizadeh O, Alhosseini SHN, et al. The effect of oxide, carbide, nitride and boride additives on properties of pressureless sintered SiC: a review. J Eur Ceram Soc. 2019 Jul;39(7):2215–2231. doi: 10.1016/J.JEURCERAMSOC.2019.02.042
- Yan YJ, Huang ZR, Dong SM, et al. Pressureless sintering of high-density ZrB 2 –SiC ceramic composites. J Am Ceram Soc. 2006 Nov;89(11):3589–3592. doi: 10.1111/J.1551-2916.2006.01270.X
- Ryazanov AI, Klaptsov AV, Koyama A, et al. Radiation swelling of SiC under neutron irradiation. J Nucl Mater. 2002 Dec;307-311:1107–1111. doi: 10.1016/j.egypro.2014.11.886
- Tavangarian F, Hui D, Li GQ. Crack-healing in ceramics. Compos Pt B-Eng. 2018 Jul;144:56–87. doi: 10.1016/j.compositesb.2018.02.025
- Wang XL, Gao XD, Zhang ZH, et al. Advances in modifications and high-temperature applications of silicon carbide ceramic matrix composites in aerospace: a focused review. J Eur Ceram Soc. 2021 Aug;41(9):4671–4688. doi: 10.1016/J.JEURCERAMSOC.2021.03.051
- Lacroix M, Nguyen P, Schweich D, et al. Pressure drop measurements and modeling on SiC foams. Chem Eng Sci. 2007 Jun;62(12):3259–3267. doi: 10.1016/j.ces.2007.03.027
- Wang W, Gao X, Li Z, et al. Fiber-laying-assisted material extrusion additive manufacturing of continuous carbon fiber reinforced SiC ceramic matrix composites. Mater Sci Eng A. 2024;890:890. doi: 10.1016/j.msea.2023.145944
- Wang W, Gao X, Zhang L, et al. Large-scale material extrusion-based additive manufacturing of short carbon fibre-reinforced silicon carbide ceramic matrix composite preforms. Virtual Phys Prototyping. 2023;18(1). doi: 10.1080/17452759.2023.2245801
- Ji RJ, Liu YH, Zhang YZ, et al. Machining performance of silicon carbide ceramic in end electric discharge milling. Int J Refract Met Hard Mat. 2011 Jan;29(1):117–122. doi: 10.1016/J.IJRMHM.2010.09.001
- Zhang H, Yang Y, Liu B, et al. The preparation of SiC-based ceramics by one novel strategy combined 3D printing technology and liquid silicon infiltration process. Ceram Int. 2019 Jun;45(8):10800–10804. doi: 10.1016/j.ceramint.2019.02.154
- Zhang H, Yang Y, Hu KH, et al. Stereolithography-based additive manufacturing of lightweight and high-strength Cf/SiC ceramics. Addit Manuf. 2020 Aug;34:9. doi: 10.1016/j.addma.2020.101199
- Chen AN, Wu JM, Liu K, et al. High-performance ceramic parts with complex shape prepared by selective laser sintering: a review. Adv Appl Ceram. 2018;117(2):100–117. doi: 10.1080/17436753.2017.1379586
- Wang WQ, Bai XJ, Zhang L, et al. Additive manufacturing of Csf/SiC composites with high fiber content by direct ink writing and liquid silicon infiltration. Ceram Int. 2022 Feb;48(3):3895–3903. doi: 10.1016/j.ceramint.2021.10.176
- Windsheimer H, Travitzky N, Hofenauer A, et al. Laminated object manufacturing of preceramic-paper-derived Si-SiC composites. Adv Mater. 2007 Dec;19(24):4515±. doi: 10.1002/ADMA.200700789
- Eckel ZC, Zhou CY, Martin JH, et al. 3D PRINTING additive manufacturing of polymer-derived ceramics. Science. 2016 Jan;351(6268):58–62. doi: 10.1126/science.aad2688
- He RX, Liu W, Wu ZW, et al. Fabrication of complex-shaped zirconia ceramic parts via a DLP-stereolithography-based 3D printing method. Ceram Int. 2018 Feb;44(3):3412–3416. doi: 10.1016/j.ceramint.2017.11.135
- Wu HD, Liu W, He RX, et al. Fabrication of dense zirconia-toughened alumina ceramics through a stereolithography-based additive manufacturing. Ceram Int. 2017 Jan;43(1):968–972. doi: 10.1016/J.CERAMINT.2016.10.027
- Ding GJ, He RJ, Zhang KQ, et al. Dispersion and stability of SiC ceramic slurry for stereolithography. Ceram Int. 2020 Mar;46(4):4720–4729. doi: 10.1016/j.ceramint.2019.10.203
- He RJ, Ding GJ, Zhang KQ, et al. Fabrication of SiC ceramic architectures using stereolithography combined with precursor infiltration and pyrolysis. Ceram Int. 2019 Aug;45(11):14006–14014. doi: 10.1016/j.ceramint.2019.04.100
- Zou Y, Li CH, Tang YH, et al. Preform impregnation to optimize the properties and microstructure of RB-SiC prepared with laser sintering and reactive melt infiltration. J Eur Ceram Soc. 2020 Dec;40(15):5186–5195. doi: 10.1016/j.jeurceramsoc.2020.07.023
- Grinchuk PS, Kiyashko MV, Abuhimd HM, et al. Advanced technology for fabrication of reaction-bonded SiC with controlled composition and properties. J Eur Ceram Soc. 2021 Sep;41(12):5813–5824. doi: 10.1016/J.JEURCERAMSOC.2021.05.017
- Xiong HW, Zhao LZ, Chen HH, et al. Building SiC-based composites from polycarbosilane-derived 3D-SiC scaffolds via polymer impregnation and pyrolysis (PIP). J Eur Ceram Soc. 2021 Feb;41(2):1121–1131. doi: 10.1016/j.jeurceramsoc.2020.09.059
- Gu J, Lee SH, Vu VH, et al. Fast fabrication of SiC particulate-reinforced SiC composites by modified PIP process using spark plasma sintering – effects of green density and heating rate. J Eur Ceram Soc. 2021 Jul;41(7):4037–4047. doi: 10.1016/j.jeurceramsoc.2021.02.025
- Im DW, Kim TE, Bae JC, et al. Manufacturing of Cf/SiC composites through combined CVI and high-pressure PIP process. Asian J Chem. 2014 Jul;26(13):4133–4139. doi: 10.14233/ajchem.2014.17732
- Margiotta JC, Zhang DJ, Nagle DC. Microstructural evolution during silicon carbide (SiC) formation by liquid silicon infiltration using optical microscopy. Int J Refract Met Hard Mat. 2010 Mar;28(2):191–197. doi: 10.1016/j.ijrmhm.2009.09.002
- Li X, Pei XL, Zhong XQ, et al. Highly effective free-radical-catalyzed curing of hyperbranched polycarbosilane for near stoichiometric SiC ceramics. J Am Ceram Soc. 2019 Mar;102(3):1041–1048. doi: 10.1111/JACE.15966