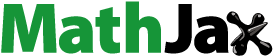
ABSTRACT
Calcium silicate is the basic component of bioactive materials that directly bond to living bone (bone-bonding property) in the body environment because calcium silicate can easily form a hydroxyapatite layer on its surface after exposure to body fluids. The organic modification of calcium silicate ceramics is expected to improve their physicochemical properties. Organic modifications of layered inorganic substances have been studied to allow drug loading and sustained release through structural changes. However, few studies have investigated the effect of organic modifications on drug-release carriers. In this study, layered calcium silicate hydrate (C-S-H) prepared via a hydrothermal process was chemically modified with alkyl groups using alkoxysilane. In addition, the loading behavior of the antibiotic levofloxacin was quantitatively analyzed. Structural analysis of the synthesized samples indicated that the interlayer distance and amount of organic content in C-S-H increased with increasing carbon chain length and concentration of alkoxysilane. Levofloxacin was successfully incorporated into the modified C-S-H. It has been suggested that drug incorporation occurs mainly via surface adsorption rather than intercalation into the interlayer.
1. Introduction
Calcium silicate-based materials have been known as important components of artificial bone since the invention of Bioglass® 45S5 [Citation1,Citation2]. An attractive characteristic of calcium silicate is capability to form hydroxyapatite on its surfaces in the body environment [Citation1,Citation2], which leads to direct bonding to living bone. This phenomenon is achieved by the dissolution of the calcium silicate substrate in the surrounding body fluids. The dissolution of calcium ions from the substrate increases the degree of supersaturation with respect to hydroxyapatite [Citation3], while the dissolution of the substrate forms favorable sites that consist of hydrated silica for the heterogeneous nucleation of hydroxyapatite on the surface [Citation3–5]. Thus, the controlled dissolution of calcium silicate is important for developing novel bone-bonding biomaterials.
Calcium silicate hydrate (C-S-H, CaxSiyOx + 2y·nH2O) is a type of layered compound that has a hetero-layer composed of calcium oxide polyhedron and silicate chains as a structural unit. This type of compound is commonly abbreviated as “C-S-H” [Citation6,Citation7]. The chemical structure of C-S-H is schematically shown in . Owing to the structural flexibility of the interlayer, organic functional groups can be readily incorporated between the layers, as schematically shown in , through synthesis with the appropriate starting compositions. Therefore, organic molecules or inorganic ions with therapeutic effects can be incorporated to improve biological functionality and control chemical durability. As Minet and collaborators previously reported, organic modification of C-S-H leads to structural changes at its interlayer [Citation8,Citation9]. They synthesized calcium silicate via a co-precipitation method using alkyl silanes with a range of chain lengths, from methyl to octadecyl groups [Citation8]. Based on X-ray diffractometry, these calcium silicates exhibited reflections attributable to the interlayer distance of their layered structure. Furthermore, the interlayer distance of the layered calcium silicates increased linearly with the alkyl chain length of the silane molecules mixed during their preparation.
The dissolution behavior of layered calcium silicates can be tuned by modifying their interlayer distances, charge densities, and hydrophilicity. The tunable dissolution of C-S-H is important for preparing novel bone-bonding biomaterials, as the dissolution behavior determines the release rate and bone-bonding ability of the administered drug. The authors of this manuscript have focused on organic modification of interlayers of C-S-H to control its dissolution. Our group previously reported that tunable dissolution behavior could be achieved by simultaneously incorporating aminopropyl and phenyl groups into the C-S-H crystalline structure [Citation10]. These materials were investigated by soaking in a buffer solution (pH 7.4) for 24 h, and they dissolved readily and released calcium and silicate ions [Citation10]. In addition, the amounts of released silicate and calcium ions tended to decrease with an increasing mass ratio of phenyl-to-aminopropyl groups in the samples. Because the charge density and hydrophilicity between layers change depending on the organic molecules incorporated into C-S-H, this result also suggests the possibility of controlled loading and sustained release of drugs and biomolecules in organically modified C-S-H.
There is a growing demand for multifunctional properties in artificial bone in addition to affinity with bone tissue. Although the synthesis of organically modified C-S-H has been previously reported [Citation8–10], little investigation has been performed into their optimization for biomedical applications, such as loading and controlled drug release. In this study, to obtain further information on the synthesis and characterization of organically modified C-S-H for biomedical applications, various amounts of sodium silicate and alkoxysilane were used as silicon sources for the preparation of organically modified C-S-H via a synthetic route under solvothermal conditions. Their microstructures were studied in terms of crystalline structure, interlayer distance, thermal stability, and surface area. The loading capacity of levofloxacin, a fluoroquinolone-based antibiotic, was also investigated. Levofloxacin was selected because it is often recommended for the treatment of bone and joint infections [Citation11].
2. Experimental method
2.1. Synthesis of organically modified C-S-H
Non-organically modified C-S-H were prepared by the hydrolysis of 5.31 g of Ca(NO3)2 4 H2O dissolved in 25 cm3 of water and 50 cm3 of ethanol containing 6.20 cm3 of tetraethyl orthosilicate. NaOH (1 g) was added to the mixture at room temperature and stirred at 500 rpm for 1 h. The mixture was transferred to a Teflon autoclave and hydrothermally treated for 48 h at 180°C. The precipitate was washed twice with ethanol and once with distilled water and centrifuged. The formed C-S-H was collected after being dried for 1 d at 40°C.
Organically modified C-S-H was prepared by the hydrolysis of a mixture of 0.0225 mol of total silicon source of alkoxysilane and Na2SiO3 in 37.5 cm3 of aqueous solution containing 5.31 g of Ca(NO3)2·4 H2O. Ethyltrimethoxysilane (C2H5Si(OCH3)3) or trimethoxy(propyl)silane (C3H7Si(OCH3)3) was selected as the alkoxysilane. The addition of NaOH, autoclaving, and washing of the formed precipitates were the same as those for the non-organically modified C-S-H. For all samples, the Ca/Si molar ratio was fixed at 1.0, and the molar fraction of alkoxysilane to the total silicon source varied from 20 to 80 mol%. The samples are referred to as R X:(100-X), where R represents the organic functional group of the alkoxysilane (R = Ethyl or Propyl), and X is the molar fraction of the alkoxysilane to the total silicon source.
X-ray diffraction (XRD; RINT2100/PC, Rigaku Co., Japan) was performed to analyze the crystalline phases. The samples were ground into a fine powder, placed on a glass cover, and then analyzed using CuK radiation (
= 0.154 nm) at 40 kV and 20 mA. The interlayer distance d was calculated from the XRD peak using the following equation:
To investigate the amounts of the organic phase and water molecules in the interlayer of the C-S-H crystals and the organic composition, thermogravimetry-differential thermal analysis (TG-DTA; DTG-60AH, Shimadzu Co., Japan) was conducted. Measurements were performed at a heating rate of 10 °C·min−1 up to 800°C in a nitrogen environment. Platinum crucibles were used and approximately 10 mg of each sample was loaded. In addition, the chemical bonds in the synthesized C-S-H were analyzed using Fourier-transform infrared (FT-IR) spectroscopy (FT/IR-6100, JASCO Co., Japan). The specific surface areas of the samples were measured using the Brunauer – Emmett – Teller (BET) nitrogen adsorption method (Nova 1000e, Quantachrome Instruments, USA).
2.2. Drug loading
Thirty milligrams of the prepared C-S-H samples were dispersed into 4.63 cm3 of ethanol by ultrasonication. Next, 0.375 cm3 of 2 mg·cm−3 levofloxacin solution was added to the resulting mixture and then homogenized by ultrasonication. The containers were immediately sealed and subjected to oscillation at a constant rate of 160 rpm at 37°C for 10 h. The resulting mixture was separated by centrifugation, washed with ethanol once, and dried at 60°C. In addition, to investigate the relationship between different starting drug concentrations and drug loading properties, the ethyl 20:80 sample was soaked in levofloxacin solutions ranging from 0.050 to 0.350 mg·cm−3. The levofloxacin concentration in the medium was analyzed using UV-vis spectroscopy (NanoDrop 2000/2000c, Thermo Fisher Scientific Inc., US) at a maximum absorption wavelength of 298 nm. Levofloxacin-loaded C-S-H was also analyzed using TG-DTA and XRD.
3. Results
3.1. Structure of organically-modified C-S-H
shows the powder XRD patterns of ethyl- and propyl-modified C-S-H and non-modified C-S-H. A characteristic peak of C-S-H at 2θ = 29.9° was observed for all the samples. In addition, a strong 001 diffraction was observed near 2θ = 6°. The highly sharp peaks at 2θ = 29.2°, 36.0°, 39.0°, 43.3°, and 48.5° observed for organically-modified samples were assigned to calcite (PDF#47–1743). shows the estimated interlayer distances of the prepared samples. For all samples, the interlayer distance was increased by the organic modification. It increased with increasing alkoxysilane content. In addition, propyl-modified C-S-H showed a longer interlayer distance than ethyl-modified C-S-H.
Figure 2. X-ray diffraction patterns of (a) ethyl-modified C-S-H and (b) propyl-modified C-S-H and non-modified C-S-H.

The FT-IR spectra of the prepared samples are shown in . Vibrations of the Si – O bonds were observed in the range of 900–1100 cm−1 for both the non-modified and modified samples. In addition, the stretching vibration of the Si – C bonds was observed in the range of 1350–1450 cm−1 for all the modified C-S-H.
Figure 4. FT-IR spectra of ethyl-modified C-S-H (left) and propyl-modified C-S-H (right) as well as non-modified C-S-H.

TG-DTA was performed on the samples to study their thermal decomposition, specifically focusing on the decomposition of organic groups and interlayer water. shows the TG-DTA profile of ethyl (20:80) as a representative sample. Three steps are observed in the TG curves. The remarkable mass loss below 200°C is accompanied by an endothermic peak originating from the losses of water molecules physically absorbed and intercalated at the interlayer of C-S-H (interlayer water). The second step, beginning at approximately 480°C or above, is due to the loss of organic species inside the particle. The third step, at approximately 800°C or above, is due to the exothermic transformation into β-wollastonite [Citation10]. presents the percentages of mass loss of water molecules and organic groups calculated from the TG profiles of all the samples. The mass loss owing to the interlayer water tends to decrease, and that is because the organic group increases with increasing molar fraction of alkoxysilane for all the samples. However, ethyl (50:50) did not follow this trend.
Table 1. Percentages of mass losses of water molecules and organic groups calculated from TG profiles of all the samples.
The specific surface areas of the organically modified C-S-H are summarized in . It tends to increase with an increase in the amount of alkoxysilane. Ethyl-modified C-S-H exhibited a higher specific surface area than propyl-modified C-S-H.
Table 2. Specific surface areas of organically modified C-S-H.
3.2. Levofloxacin loading into organically modified C-S-H
No notable changes were observed in the XRD patterns before and after levofloxacin loading (data not shown). shows the amount of levofloxacin loaded onto 30 mg of the modified C-S-H. The samples with 50 mol% alkoxysilane showed the highest levofloxacin loading capacity for both ethyl- and propyl-modified C-S-H. Propyl-modified C-S-H showed a higher loading than ethyl-modified C-S-H at an alkoxysilane content of 40 mol% or less, but the opposite trend was observed at 50 mol% or more. To investigate the maximum loading capacity of the organically modified C-S-H, the amount of levofloxacin loaded onto ethyl 20:80 was plotted against the initial levofloxacin concentration (). The loading amount increased monotonically with the initial concentration. However, no plateau was observed, even at a high initial drug concentration.
Figure 7. Relationship between initial concentration of levofloxacin and loaded amount onto 30 mg of ethyl 20:80.

TG-DTA was performed on the levofloxacin-loaded C-S-H to study thermal decomposition, with a specific focus on the decomposition of absorbed levofloxacin to investigate the levofloxacin taken up by C-S-H quantitatively. The mass loss was greater for all levofloxacin-loaded C-S-H than for C-S-H alone, which was calculated using the following equation, assuming that the inorganic/organic ratio of the sample was equal before and after levofloxacin loading:
Before: Before levofloxacin loading
After: After levofloxacin loading
shows the difference in mass loss in the ethyl-modified and propyl-modified C-S-H, as measured by TG. The calculated levofloxacin content was greater for the propyl-modified C-S-H than for the corresponding ethyl-modified C-S-H.
Table 3. Percentages of mass losses of levofloxacin and organic groups calculated from TG profiles of levofloxacin-loaded ethyl- and propyl-modified C-S-H.
4. Discussion
4.1. Structure of organically modified C-S-H
Organically modified C-S-H was successfully prepared via hydrothermal synthesis in the presence of an alkoxysilane. The presence of the stretching vibration of the Si – C bonds in the FT-IR spectra revealed that the organic groups were covalently linked to the inorganic part of the C-S-H via Si – C bonds (see ).
The interlayer distance of the modified C-S-H increased with increasing alkoxysilane content (). In addition, it was greater for the propyl-modified C-S-H than for the ethyl-modified C-S-H, even for the same fraction of organic modification. By contrast, the TG results revealed that the organic fraction increased with increasing organic species in the samples, leading to a greater mass loss due to combustion (See ). The incorporation of a large amount of alkoxysilane with a longer hydrocarbon chain is effective in expanding the interlayer distance of C-S-H.
Calcite formation was observed only for the organically modified C-S-H, despite the fact that CO2 dissolution may have occurred in all samples during the hydrothermal reaction (See ). The interlayer became more hydrophobic when the alkyl groups were incorporated. Consequently, the reaction of some calcium ions remaining in the medium with the surrounding carbonate ions was facilitated. Calcite does not form in organically modified C-S-H synthesized at ambient pressure [Citation8,Citation9]. The investigation of the mechanism of calcite formation is currently ongoing, and we hope to elucidate it in future contributions. However, due to the requirement for hydrolysis of the alkoxy groups, the formation of organo-modified C-S-H is believed to progress more slowly than that of C-S-H, leading to increased calcite formation. Besides, developing a synthesis method that minimizes calcite formation by controlling the hydrothermal conditions and removing CO2 gas from the medium will be necessary.
As for the interlayer water, the absorption bands at 1630 and 3000–3700 cm−1 corresponding to the stretching and bending vibrations of water molecules, respectively, were negligible in the FT-IR spectra (See ; note that the latter region is not shown), suggesting that a small amount of water is present in C-S-H. In addition, the TG results indicated that the water content decreased slightly with an increase in the organic fraction (). The enhanced hydrophobicity owing to organic modification further inhibited water intercalation.
4.2. Levofloxacin loading into organically-modified C-S-H
The UV – vis results showed that levofloxacin was successfully loaded into the organically modified C-S-H (). This is also supported by the TG results, which showed an enhanced loss of organic phases upon levofloxacin loading (See ). For both the ethyl- and propyl-modified samples, C-S-H modified with 50 mol% alkoxysilane showed the maximum drug adsorption. This could be owing to the relatively high specific surface area, which contributed to enhanced loading. The diameter of quinoline, with a chemical structure corresponding to the hetero 2-membered ring of levofloxacin, is 0.711 nm [Citation12], which is comparable to the interlayer distance of organically modified C-S-H. In addition, no changes were observed in the interlayer distances of the samples, even after loading with levofloxacin. Based on these results, it was predicted that drug loading occurred in the form of surface adsorption instead of incorporation into the interlayer. Furthermore, the levofloxacin uptake was enhanced at higher initial concentrations. However, the saturated capacity of levofloxacin uptake was not observed in the current study, suggesting that multilayer surface adsorption of levofloxacin occurred. Levofloxacin is adsorbed in multiple layers, depending on the conditions of the substrate [Citation13,Citation14].
5. Conclusion
The interlayer of C-S-H can be organically modified using alkoxysilane as the precursor. The obtained C-S-H was loaded the loading ability of with levofloxacin. However, it was assumed that levofloxacin was not incorporated into the interlayer but was mainly adsorbed onto the surface layer. In the future, developing a chemical treatment method that can further expand the interlayer to increase levofloxacin loading would be desirable.
Acknowledgments
This research was supported in part by the Leading Initiative for Excellent Young Researchers (LEADER), Ministry of Education, Culture, Sports, Science, and Technology (MEXT), Japan, JSPS Kakenhi JP22K04711, JP22K19894, and Yoshida Foundation for the Promotion of Science, Japan.
Disclosure statement
No potential conflict of interest was reported by the author(s).
Additional information
Funding
References
- Kokubo T. Recent progress in glass-based materials for biomedical applications. J Ceram Soc Jpn. 1991;99(1154):965–973. doi: 10.2109/jcersj.99.965
- Hench LL. Bioceramics. J Am Ceram Soc. 1998;81(7):1705–1728. doi: 10.1111/j.1151-2916.1998.tb02540.x
- Ohtsuki C, Kokubo T, Yamamuro T. Mechanism of apatite formation on CaO SiO2 P2O5 glasses in a simulated body fluid. J Non-Cryst Solids. 1992;143:84–92. doi: 10.1016/S0022-3093(05)80556-3
- Cho SB, Nakanishi K, Kokubo T, et al. Dependence of apatite formation on silica gel on its structure: effect of heat treatment. J Am Ceram Soc. 1995;78(7):1769–1774. doi: 10.1111/j.1151-2916.1995.tb08887.x
- Tanahashi M, Yao T, Kokubo T, et al. Apatite coated on organic polymers by biomimetic process: improvement in its adhesion to substrate by NaOH treatment. J Appl Biomater. 1994;5(4):339–347. doi: 10.1002/jab.770050409
- Gard J, Taylor H. Calcium silicate hydrate (II)(“CSH (II)”). Cem Concr Res. 1976;6(5):667–677. doi: 10.1016/0008-8846(76)90031-4
- Cong X, Kirkpatrick RJ. 29 Si and 17O NMR investigation of the structure of some crystalline calcium silicate hydrates. Adv Cem Based Mater. 1996;3(3):133–143. doi: 10.1016/S1065-7355(96)90045-0
- Minet J, Abramson S, Bresson B, et al. New layered calcium organosilicate hybrids with covalently linked organic functionalities. Chem Mater. 2004;16(20):3955–3962. doi: 10.1021/cm034967o
- Minet J, Abramson S, Bresson B, et al. Organic calcium silicate hydrate hybrids: a new approach to cement based nanocomposites. J Mater Chem. 2006;16(14):1379–1383. doi: 10.1039/B515947D
- Nakamura J, Suzuki Y, Narukawa R, et al. Preparation of layered calcium silicate organically-modified with two types of functional groups for varying chemical stability. J Asian Ceram Soc. 2020;9(1):113–123. doi: 10.1080/21870764.2020.1854925
- Asseray N, Bourigault C, Boutoille D, et al. Levofloxacin at the usual dosage to treat bone and joint infections: a cohort analysis. Int J Antimicrob Agents. 2016;47(6):478–481. doi: 10.1016/j.ijantimicag.2016.03.003
- Corma A, Fornés F, Rey F. Quinoline as a probe molecule for determination of external Brönsted and Lewis acidity in zeolites. Zeolites. 1993;13(1):56–59. doi: 10.1016/0144-2449(93)90023-V
- Yan B, Niu CH. Modeling and site energy distribution analysis of levofloxacin sorption by biosorbents. Chem Eng J. 2017;307:631–642. doi: 10.1016/j.cej.2016.08.065
- Iwuozor KO, Abdullahi TA, Ogunfowora LA, et al. Mitigation of levofloxacin from aqueous media by adsorption: a review. Sustain Water Resr Man. 2021;7(6):100. doi: 10.1007/s40899-021-00579-9