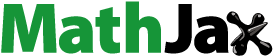
Abstract
Thermodynamics for ideal plastic deformation causing no disorganization of the structure in the deformed body, which was discussed by P. W. Bridgman studied (1950), revealed that the concept of entropy was still applicable to its irreversible process. Noting that the structural invariability in an ideal plastic body is physically equivalent to the prerequisite of thermodynamics, namely, thermodynamic quantities must be independent of the macroscopic body shape, the generalized concept of entropy can be extended to the other thermodynamic potentials such as internal energy, free energies and so on. Here the extended generalization for ideal plastic deformation is theoretically justified on the basis of the irreversible thermodynamics constructed by Prigogine and his discipline. Thermodynamic state of the ideally deformed body is found to be specified both by the generalized thermodynamic potential (S, U, F, H or G) and by the irreversible potential energy J which drives the plastic deformation.
PUBLIC INTEREST STATEMENT
“When a piece of straight wire of metal is subjected to bending force, the wire deforms plastically into a bent state. Once bent, the wire never returns to its original shape by itself. This one way process is called irreversible. The irreversible process is qualitatively understood by the second law of thermodynamics, namely by the law of entropy increase. Since thermodynamics deals with the equilibrium state, at first glance no entropy is defined for the irreversible process. A bulk body deforms irreversibly similar to the wire and therefore no entropy appears to be defined either. When no disorder is observed in the structure of the deformed body, however, the entropy can be defined and is found constant through the process of deformation. This type of plastic deformation is named ideal. On the basis of the ideal deformation, thermodynamics of the deformed body with disordered structures will be studied in future.”
1. Introduction
Thermodynamics of ideal plastic deformation at constant temperature and pressure was studied earlier by P. W. Bridgman in USA (1950) (Bridgman, Citation1950). He examined slip processes of the deformation in detail and found that all the thermal energy produced by the plastic deformation flowed into the heat bath out of the deformed body, leaving no structural disorganization in the deformed body at all. Consequently no entropy increase is observed in the ideally deformed body.
He was mainly interested in the dual aspect of entropy shown by the stress–strain loop (Figure ). The reversible aspect is reflected in the maintenance of the constant entropy during the ideal plastic deformation, whereas the irreversible aspect is reflected in the clockwise path of the loop as well as in the hysteresis loss. In order to elucidate the dual aspect above, he extended the concept of thermodynamic state mainly focusing on entropy and found that the thermodynamic state and entropy are still applicable to the irreversible process of the ideal plastic deformation. Hereafter the extended state and entropy are denoted as generalized state and entropy, respectively.
Figure 1. (a) Stress–strain loop OABCDEO of ideal plastic body (Bridgman, Citation1950). Only the clockwise path is allowed owing to the irreversible character of the plastic deformation. The loop is closed exactly at the starting point O and the identical loop could be repeated indefinitely. The enclosed area of the loop is known as hysteresis loss ().The yield stress and strain are denoted by
and ±
, respectively. The total strain
is composed of two kinds of strain, i.e. elastic (
) and plastic strain (
). (b) Plastic strain
, its increment
and decrement
.
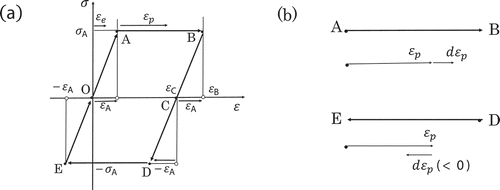
To the best of authors knowledge, no significant studies have been made on the thermodynamics of plastic deformation since Bridgman’s work, except for the work of J. Kestin and his groups (Kestin, Citation1987, Citation1993; Ponter, Bataille, & Kestin, Citation1979) and that of Kato (Citation2008). Kestin’s groups applied non-equilibrium thermodynamics to the Frank-Read source of dislocations, but unfortunately they could not complete their work. On the other hand, Kato (Citation2008) applied equilibrium thermodynamics to the plastic deformation with deep consideration. However, there remains an interesting issue related with the irreversible aspect of the ideal plastic deformation.
At about the same time of Bridgman’s work, I. Prigogine and his school in Belgium dealt with irreversible thermodynamics of chemical reactions, thermoelectricity and diffusion (Bridgman, Citation1950; Kestin, Citation1987; Kondenpudi & Prigogine, Citation1998). However, they did not apply their theory to the ideal plastic deformation that was one of the simplest irreversible phenomena. In this paper, we shall apply their irreversible thermodynamics to the ideal plastic deformation to justify Bridgman’s generalized entropy.
2. Stress–strain loop and thermodynamics
Figure ) shows a stress and strain loop of an ideal plastic body. Only a clockwise path of the loop (OABCDO) is allowed owing to the irreversible character of plastic deformation. All the deformation dealt in the present work is assumed to be at constant temperature and pressure
so that they are not shown explicitly unless otherwise stated.
The plastic deformation proceeds along the path from A to B at constant yield stress and from D to E at
(Figure ). The plastic deformation specified above will be abbreviated as PD. We shall be concerned with the thermodynamics of PD.
The loop of ideal plastic deformation is assumed to cycle indefinitely so that the structure of the body must return exactly to the original one after each cycle completed (Bridgman, Citation1950). Consequently the thermodynamic state of the plastically deformed body is uniquely defined by a set of stress and total strain
of the loop. Either
or
alone is not sufficient. What PD does is to change the shape of the body keeping the structure unchanged (Figure ).
Figure 2. A series of deformed states A, B and C of Figure ) is schematically illustrated. The unstrained body is denoted by O. The yield stress () is given by weight (
). A stopper is set for B to suppress the indefinite extension of the body. PD is observed from A through B.
is the total plastic strain given. All the difference between the states A and B is in their body shapes and no difference in their structures (Section 1.).
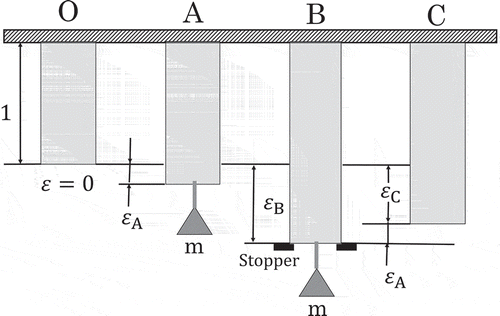
The structural invariability is physically equivalent to the prerequisite for thermodynamics, i.e. independence of thermodynamics from the body shape. This equivalence will play an important role in generalizing thermodynamic quantities other than Bridgman’s entropy.
3. Entropy, internal energy and Helmholtz energy
PD from A to B and from D to E of Figure changes the shape of the body without any structural disorganization. Because of the independence of thermodynamics from the body shape, the thermodynamic state must remain unchanged from A to B and from D to E. In other words, the same thermodynamic state and potentials at A and D are maintained throughout the subsequent PD. Consequently all the states from A to B and those from D to E must be reversibly exchangeable. We shall confirm this observation based on Prigogine’s irreversible thermodynamics later on.
Employing notations in the text book of Kondepudi and Prigogine (Kondenpudi & Prigogine, Citation1998), the entropy differential of the deformed body is given by a sum of
and
respectively due to the irreversible deformation and due to the reversible exchange of energy with the heat bath (Figure ). Namely
Figure 3. Entropy differential () of the ideal plastic body (darkly shaded). It consists of
and
where the former is due to the irreversible plastic deformation and the latter due to the reversible exchange of energy with the heat bath (slightly shaded). When the heat flows out of the body, the sign of
is taken to be negative. Force
(given by external weight
of Figure ) is applied to extend the body.
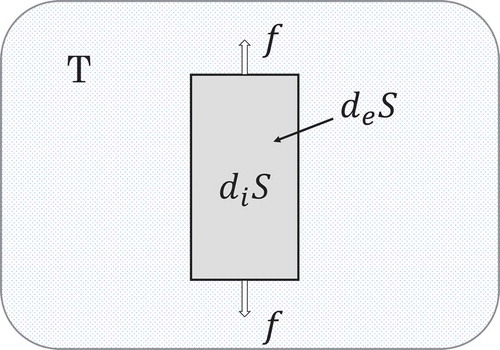
For the sake of convenience, let us call Equaton (1) Prigogine’s equation.
Because of the structural invariability, all the work done during PD is converted into heat and flows into the heat bath without leaving any effect in the deformed body. We obtain
and therefore
The inequality in the first equation of (2) is attributable to the clockwise path of the stress-strain loop (Figure ). Equation (3) tells us that the entropy of the body () remains constant during PD. This is nothing but the prediction of Bridgman’s argument about the generalized entropy.
Equation (3) enables us to generalize the other thermodynamic potentials in the same way. Let us generalize internal energy at first.
where is the work done on the body and
is the heat flowing out of the body into the heat bath. Owing to Equation (3), we have
Internal energy () remains constant during PD and is thus generalized as entropy is (See Section 1. and Equation (3)).
As to Helmholtz energy () we obtain
where we make use of Equations (3), (6) and constant . In a similar manner
is generalized for PD.
We thus proved the invariance of the thermodynamic potentials ,
and
during PD.
Integrating (Equation (2)), we obtain the hysteresis loss (
).
4. Enthalpy and Gibbs energy
Now let us ask whether or not enthalpy () and Gibbs energy (
) can be generalized in the same manner.
First of all we point out that differentials of enthalpy and Gibbs energy must be zero simply because thermodynamic state is generalized for PD (Section 3.).
Applying Legendre transformations to and
intentionally, we would obtain
and
where we substituted into the above equations. We have an extra term
in the equations
which is denoted by .
The extra term arises simply because Legendre transformations have been applied to and
without due attention. The thermodynamic state of PD is not specified by
alone but by a set of stress
and total strain
(Figure )) and therefore the Legendre transformations (Equations (9) and (10)) are no longer valid for PD.
Integrating Equation (11), however, we obtain a physically significant quantity , i.e.
where we assume and
as the initial condition.
is seen to be the potential energy of the weight (
) required to drive PD. The clockwise path of the loop implies
(Figure ) and thus PD proceeds always in the direction of decreasing
(Equation (12)).
We note that PD is externally driven, for instance, by the downward motion of weight m (Figure ) and is nothing to do with the thermodynamic state of the deformed body itself. This is the reason why differentials of the generalized and
remain constant throughout PD.
To sum up, the thermodynamic state of PD is specified by the generalized state variables (,
,
,
or
) and additionally by the potential energy
.
5. Summary
(1) Thermodynamics of ideal plastic deformation studied by Bridgman is reexamined in two ways. One is based on the independence of thermodynamic formulation from the macroscopic body shape and the other on Prigogine’s irreversible thermodynamics.
(2) The thermodynamic state and the thermodynamic potentials are so generalized as to be applicable to the irreversible process of ideal plastic deformation.
(3) The generalization above enables us to understand the dual aspect of reversibility and irreversibility inherent to the ideal plastic deformation.
(4) Thermodynamic state of ideal plastic deformation is specified by generalized thermodynamic potentials (,
,
,
and
) and potential energy
.
(5) The ideal plastic deformation always proceeds in the direction of decreasing . The potential energy
arises from the external force applied to the deformed body and is responsible for the hysteresis loss.
Acknowledgements
We thank Dr. Masahiro Koiwa for encouraging us to complete the present work. One of us (K.O.) thanks Dr. Masaharu Kato for letting him know Kestin’s work.
Additional information
Funding
Notes on contributors
Keiichi Ogawa
“The first author was impressed by the pioneering work of Bridgman (Citation1950), the thermodynamics of ideal plastic deformation and generalized entropy in his graduate school days. It was only recently reconsidered when he came across the book, Modern Thermodynamics by Kondenpudi and Prigogine (Citation1998). He noted that their argument on the falling of an object to the bottom of a viscous fluid was closely parallel to the ideal plastic deformation. We then find that not only entropy but also all the other thermodynamic potentials are generalized for the irreversible process of ideal plastic deformation. Application of equilibrium thermodynamics to the irreversible process is a tricky issue. It takes more than half a year of serious and frequent discussions among us to elucidate the thermodynamics of ideal plastic deformation. We hope that the present result plays a role similar to that of ideal gases.”
References
- Bridgman, P. W. (1950). The thermodynamics of plastic deformation and generalized entropy. Review of Modern Physics, 22, 56–63.
- Kato, M. (2008). Selected topics in strength of materials II—thermodynamics and kinetics of deformation. Materia Japan, 47, 318–322. (in Japanese).
- Kestin, J. (1987). Patterns, defects and microstructures in nonequilibrium systems-applications in materials science (pp. 202–219). Leiden, Netherlands: Martinus Nijhoff Publishers.
- Kestin, J. (1993). Internal variables in the local-equilibrium approximation. Journal of Non-Equilibrium Thermodynamics, 18, 360–379.
- Kondenpudi, D., & Prigogine, I. (1998). Modern thermodynamics (pp. 88- 90 ). West Sussex, England: Wiley.
- Ponter, A. R. S., Bataille, J., & Kestin, J. (1979). A thermodynamic model for the time dependent plastic deformation of solids. Journal of de Mecanique, 18, 511–539.
- Quoted in “Note added on reading proof” of Ref.1.