ABSTRACT
Low-temperature gaseous nitriding response of annealed 2205 stainless steel was investigated through a series of nitriding treatments at different temperatures in the range 380–430°C for 25 h. Reflected light microscopy, X-ray diffraction and hardness indentation were utilized to investigate the dependence of the formation of nitrides and cracks on pre annealing temperatures and subsequent nitriding parameters. For most annealing and nitriding conditions applied, the surface adjacent region of the steels was transformed into expanded austenite, or a mixture of expanded austenite and expanded ferrite, supplemented by the presence of iron-based nitrides. Nitriding at a relatively low temperature at a gentle nitriding potential was found to be acceptable as it resulted in a sound nitrided case, where neither chromium nitrides nor cracks developed. Annealing at a relatively low temperature can improve the threshold of nitriding temperature up to 400°C without cracking, while annealing at a high temperature promotes the formation of nitrides.
Introduction
Low-temperature nitriding at a temperature below 723 K is common practice for improvement of surface properties of stainless steels, such as fatigue and wear resistance [Citation1,Citation2]. The exhibited favorable surface properties result from large quantities of nitrogen that are dissolved in the surface zone of stainless steel, the formation of "expanded case" in interstitial supersaturated state. If nitride formation (e.g. CrN) is prevented, the corrosion properties, especially the pitting and crevice corrosion resistance, are significantly enhanced [Citation3]. The fundamental to low-temperature nitriding of austenitic stainless steels (e.g. AISI 304/316) has been well revealed [Citation2]; in particular, various methods [Citation4–7] for production of nitrogen expanded austenite on austenitic stainless steels developed and become gradually mature in industrial applications [Citation8,Citation9]. For duplex stainless steels (DSS), despite the excellent combination of mechanical strength and corrosion properties over that of austenitic stainless steels [Citation10,Citation11], it is indeed necessary to further improve its surface properties in applications where high wear resistance and corrosion resistance is required [Citation12,Citation13]. In this regard, low-temperature nitriding of DSS is considered promising means because interstitially dissolved nitrogen positively affect the mechanical properties and significantly contributes to the enhancement of the PREN (pitting resistance equivalent number) and MARC (measure of alloying for resistance to corrosion) over other alloy elements, thereby leading to a significant improvement of the resistance to crevice and pitting corrosion [Citation11,Citation14].
Low-temperature nitriding of DSS involves interactions between nitrogen with both BCC (ferrite or martensite) phase and FCC (austenite) phase, indicating a difference in thermodynamics and kinetics at the steel surface. Upon nitriding expanded austenite (γN) typically develops in austenite grains, while CrN nitrides as well as iron-based nitrides preferentially form within ferrite zone [Citation7,Citation15–17], and needle-like structures, commonly interpreted as deformation bands [Citation18], α″-Fe16N2 [Citation15], or γ'M – austenite [Citation17], incidentally develop in ferrite. This implies a relatively strict formulation of nitriding parameters for DSS, e.g. a lower nitriding temperature, aiming to prevent the development of nitrides. In addition, either a single nitrogen expanded austenite case [Citation4,Citation5,Citation19] or a mixed case comprising expanded austenite and expanded ferrite [Citation7,Citation20,Citation21], can develop on DSS during low-temperature nitriding, depending on steel composition, processing method, and nitriding parameters. A relatively high nitriding temperature (>673 K) which would provide a favorable kinetic condition is claimed to contribute to the development of a single expanded austenite case [Citation7,Citation19].
On the other hand, it is recognized in typical DSS (22∼24 Cr wt. %), nitrogen diffuses deeper in ferrite than in austenite [Citation22–24], in agreement with the fact that nitrogen diffusion is faster in the BCC phase than in the FCC phase. Ref. [Citation7] reports a thicker (observable) nitrided zone in austenite than in ferrite in plasma nitrided super DSS 2507 alloy, where the earlier precipitation of nitrides in ferrite associated with the high chromium content (∼26 wt%) is mainly responsible for the slower nitrogen diffusion. This is not a general rule in low-temperature nitriding of super DSS, as a contrary trend that a slight thicker case developed on the ferrite phase is observed in Ref. [Citation25]. Moreover, depending on the processing conditions, the lateral diffusion of nitrogen between ferrite and austenite can yield a homogeneous nitrided case [Citation26,Citation27].
It is noted that nitriding of DSS is prone to cracks, which nucleate within the nitrided case and propagate parallel to the surface [Citation3,Citation6,Citation8–11]. This is one serious obstacle to the transformation of low-temperature nitriding technology to industrial practice. Our previous research demonstrated that cracking can be prevented by combining a low nitriding temperature with a gentle nitriding potential [Citation28]. It follows from the above investigations that the most concerned variables are the temperature and the nitriding potential.
Phase fraction as well as accompanied composition partitioning between ferrite and austenite is a primary consideration in practical application of DSS [Citation10,Citation11]. While the favorable properties of duplex stainless steels are commonly attributed to equal amounts of ferrite and austenite, a slight adjustment is necessary in certain conditions, for instance, a higher fraction of austenite for the best toughness and processing characteristics [Citation29]. The deliberately adjusted phase fraction, in accordance with the production/application demands, may bring about a (net) change in thermodynamic and kinetic of nitriding, and possibly, affect the development of cracks.
The present work aims at elucidating the dependence of the formation of nitrides and cracks on pre annealing temperatures and nitriding parameters in duplex stainless steel. The microstructural evolution, nitriding kinetics, and the development of cracks in the nitrided case, on ferrite/austenite distributions, were investigated at various annealing and nitriding conditions.
Experimental
The substrates used in this study were slices of Ø 20 × 3 mm shaped with an abrasive wheel cutting machine from a DSS 2205 rod, which was supplied in hot rolled and 1060°C annealed condition. Chemical composition of the material is listed in .
Table 1. Chemical composition of 2205 steel.
The as-prepared slices were subjected to annealing at 1000°C and 1200°C for 2 h, respectively. It is demonstrated that the annealing treatments led to changes in phase fractions of austenite and ferrite and therefore composition partitioning between austenite and ferrite, without introducing any precipitates (details please refer to [Citation30]).
Prior to nitriding, the annealed slices were further processed into strips of 20 × 6 × 3 mm3; both surfaces of the strips were ground and polished with 3 μm diamond. Low-temperature gas nitriding (LTGN) was performed in a Netzsch Simultaneous Thermal Analyzer (STA) 449 for 25 h at 430°C, 400°C and 380°C, respectively. Pure ammonia gas or a 60:40 NH3:H2 gas mixture was chosen as the nitriding atmospheres. In all nitriding treatments, 5% N2 was added to the atmosphere through the measurement compartment (as protective gas), so that the nitriding potentials are infinite and 2.43 bar−1/2 for pure ammonia and NH3–H2 mixture, respectively.
Phase analysis was performed on the surface of the nitrided specimens by X-ray diffraction (XRD) on a D/max-2200-3KW equipped with Cu Kα-radiation, which has a wavelength of 1.5418 Å. Data was collected at 0.05°2θ step size and 4 s counting time per step in the scattering angle range 30–70°2θ. The nitrided specimens were sectioned and mechanically polished, followed by etching at room temperature with aqua-regia consisting of 75 vol% HCl and 25% vol% HNO3 for 10∼15 s. Microstructure of the nitride case was examined on cross section of the specimens using a Zeiss Axio optical microscope. Micro-hardness indentations were conducted on a VH1102 hardness indenter using a load of 10 g and dwelling time of 10 s. Presented hardness values are the average of at least 4 indentations.
Results
Microstructure of the nitrided case
LTGN in pure NH3 (KN = ∞)
presents light-optical micrographs of the cross-sections of specimens annealed and nitrided in pure ammonia at different temperatures. For all applied nitriding temperatures, a nitrided zone is present adjacent to the surface. The depth of the nitrided zone shows a strong dependence on the nitriding temperature, i.e. the higher the nitriding temperature, the deeper the nitrided zone.
Figure 1. Light-optical micrographs of DSS 2205 annealed and subsequently nitrided in pure NH3 for 25 h at 380°C, 400°C and 430°C, respectively.
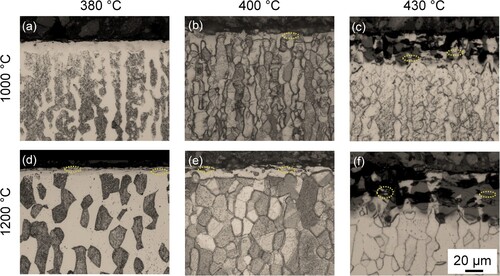
For the 1000°C annealing condition, gaseous nitriding at 380°C produced a continuous nitrided case, which features monophase after etching and fairly uniform in thickness ((a)). With increasing nitriding temperature, the nitrided case is no longer uniform, and dart-etching zone appear at a 430°C nitriding temperature ((c)). The X-ray diffractograms in (a) show that both ferrite and austenite were transformed into expanded austenite upon nitriding at 380°C, while at higher nitriding temperatures both expanded austenite and expanded ferrite developed. In particular, development of CrN precipitates as well as ϵ is identified, which is more pronounced for higher nitriding temperatures, consistent with the light-optical micrographs in (a–c). Furthermore, diffraction of γ'N-M4N1-x (M = Fe, Ni, Cr) iron nitride is observed for 400°C nitriding temperature, which appears more intense at 430°C.
Figure 2. X-ray diffractograms for the surface-adjacent zone of the specimens pre-annealed at (a) 1000°C and (b) 1200°C, and subsequently nitrided at different temperatures in pure NH3.
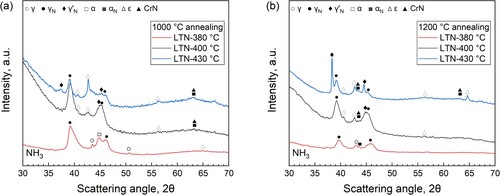
In comparison, the nitrided case at the 1200°C annealed specimen is found to be shallower, in which cracks parallel to the surface developed. Increasing nitriding temperature results in non-uniform growth of the nitrided zone toward the core in both annealed specimens, arc-like shape case/substrate interfaces are observed ((b,c,e,f)). Moreover, dark-etched zones are observed in the nitrided zone, indicating reduced corrosion resistance to the applied etchant. According to previous investigations, this is associated with the development of CrN nitrides. In addition, as marked in , cracking occurred at higher nitriding temperatures. The formation of expanded ferrite after nitriding at 380°C and the intense diffraction of γ'N ((b)) are associated with the higher fraction of ferrite after annealing at 1200°C. The X-ray diffractograms in (b) demonstrate that both expanded austenite and expanded ferrite develop at the surface of the specimen nitrided at 380°C, and for higher nitriding temperatures the diffraction of CrN and γ’N appears more intense. This is associated with the higher fraction of ferrite after annealing at 1200°C.
LTGN in NH3–H2 atmosphere (KN = 2.43 bar−1/2)
Micrographs of cross-sections of DSS 2205 annealed and nitrided in NH3–H2 atmosphere at different temperatures are shown in . As compared to the nitriding response in pure ammonia, a similar temperature influence on the growth kinetics of the nitrided case is observed upon nitriding with NH3–H2 mixtures, while for all temperature conditions the gentle nitriding potential resulted in a shallower nitrided case.
Figure 3. Light-optical micrographs of DSS 2205 annealed and subsequently nitrided in NH3–H2 mixtures for 25 h at 380°C, 400°C and 430°C, respectively.
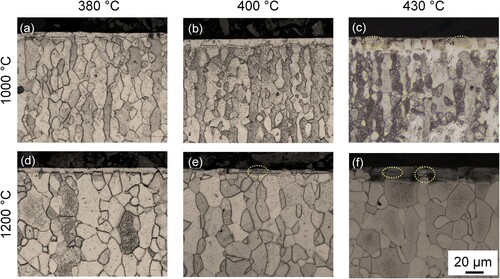
Nitriding at a relatively low temperature (i.e. ≤400°C) yielded a corrosion-resistant expanded case in the 1000°C annealed specimen, where no evident cracking is noticed ((a,b)). As the nitriding temperature is up to 430°C, both cracks and dark-etching zones appear. In 1200°C annealed specimens, nitriding at 380°C led to a sound case where no nitride is formed and cracking is suppressed ((d)). Increase in nitriding temperature (≥400°C) results in considerable development of both cracks and CrN nitrides. Nevertheless, this is not as prevalent as that for a higher nitriding potential.
shows X-ray diffractograms obtained from specimens annealed and subsequently nitrided in NH3–H2 atmosphere, which provides a lower nitriding potential compared to that for pure ammonia. It shows clearly that at all nitriding temperatures co-development of expanded austenite and expanded ferrite occurred. Despite the presence of dark-etched regions within the nitrided zone for both 400°C and 430°C conditions ((d–i)), XRD analyses indicate a lower content of CrN precipitates ((b,c)) compared to a pure NH3 condition, consistent with the microstructural observations in and .
Hardness-depth profile
The hardness profiles measured on the cross section of the annealed and low-temperature nitrided DSS 2205, along ferrite and austenite bands, are given in . A similar trend of hardness evolution with depth is observed in both austenite and ferrite bands for all nitriding conditions. The significant hardening effect is achieved in the surface-adjacent zone of about 10∼30 μm, depending on the treatment conditions. A sudden drop in hardness appear at a position close to the case/substrate interface in all nitrided specimens. For all nitrided specimens, the hardness distribution is higher in ferrite bands than in austenite bands. Noting that for most nitriding conditions, the maximum hardness appears close to the medium of the nitrided case rather than at the outermost surface. This could be ascribed to the presence of cracks and the structure of the nitrided cases. The hardness close to the outermost surface for a lower nitriding potential is higher than for a higher nitriding potential. The higher ferrite amount, corresponding to a higher pre annealing temperature, results in deeper nitrided case. With lowering the nitriding temperature or nitriding potential, the case depth, i.e. the strong hardening zone adjacent to the surface, decreases.
Discussion
Depending on the pre-annealing condition, nitriding temperature and nitriding potential, the LTGN responses are different. At a certain nitriding potential, both CrN and ϵ tend to develop with increasing temperature. This indicates that a nitriding temperature below 400°C is a necessary choice to prevent the precipitation of CrN nitrides which negatively influence the corrosion resistance of the nitrided case, consistent with the previous investigation [Citation6]. It is noted that for most processing conditions, a gentle nitriding potential yielded more CrN and ϵ, while the pre-annealing treatments had no measurable influence. According to the above findings, it is seen that the development of CrN nitrides mostly occurred in the ferrite phase upon nitriding in NH3–H2 mixtures, while nitriding in pure NH3 resulted the development of CrN nitrides in both ferrite and austenite zones. This is attributed to the higher Cr content in ferrite, and possibly, associated with the favorable crystallographic orientation relation between CrN and ferrite [Citation31]. This also explains the pre-annealing induced discrepancy in the microstructure of the nitrided case. Irrespective of the nitriding potential, the formation of CrN nitrides is more prevalent for a higher pre-annealing temperature corresponding to a higher content of ferrite.
Despite the presence of the ϵ phase in the nitrided specimens, no sufficient evidence has been adduced upon whether the ϵ phase is stress-induced (martensite) or chemically induced (nitrides). Generally, the formation of ϵ-martensite is promoted by the composition induced stresses associated with high nitrogen contents [Citation4]. A lower content of nitrogen in the nitrided case is anticipated upon nitriding in NH3–H2 mixtures, which offer a lower nitriding potential. The stress induced by nitrogen expansion is expected to be lower than that from a higher nitriding potential, thereby providing a relatively unfavorable condition for the formation of ϵ-martensite. On the other side, the lower nitriding potential for the NH3–H2 series also provides a lower chemical driving force for ϵ nitride formation. The observed difference in the nitriding response between the nitrided conditions is explained in Ref. [Citation28]. It is the competition between CrN formation and ferrite to austenite conversion that determines the outcome of the nitriding response of the ferrite phase in DSS. Dissolving N in ferrite promotes a transformation to austenite, while the higher content of Cr and a crystal structure ferrite results in an early nucleation of CrN. At a lower temperature (380°C) and a higher nitriding potential (pure NH3), the higher nitrogen content contributes to the development of monophase expanded austenite, thereupon the higher nitrogen content and the higher volume expansion promote the formation of ϵ phase ((a)). For the 1200°C annealing condition, however, dissolving more nitrogen is necessary to transform more ferrite into austenite and consequently expanded austenite, so the amount of ϵ phase would be lower or even unmeasurable ((b)). For a lower nitriding potential, the transformation from ferrite to austenite could also occur at the outermost layer where the highest nitrogen content is present. In deeper zone the dissolved nitrogen content is insufficient to enable austenite formation, and expanded ferrite develops ((b)). No expanded ferrite was detected in the 1200°C annealed specimens, which could be related to the higher diffusion kinetic generated by the presence of more ferrite. The lateral diffusion of nitrogen would lower the nitrogen content at the (local) outermost surface layer, where a relatively small amount of ϵ phase developed, but the formation of expanded austenite occurred ((b)). CrN nitrides are more prone to form at higher temperatures (400°C and 430°C). At a lower nitriding potential, nano-scale CrN formed in the ferrite phase, the left N is insufficient to convert ferrite into austenite and consequently γ'N nucleates on top of ferrite. This can explain the higher hardness obtained at a lower nitriding potential (). For a higher nitriding potential, sufficient N is added to transform ferrite into austenite and thereafter into expanded austenite, but also CrN will develop, which is more pronounced for 1200°C annealed case because the existence of more ferrite ( and ).
The development of cracks is closely related to the incoherent thermal shrinkage of the expanded austenite and expanded ferrite [Citation28]. The observation that a higher nitriding potential proceeds the formation of the cracks could be ascribed to the higher stress in both phases as a consequence of higher nitrogen contents. Thereby, the combination of temperature and nitriding potential should be chosen to prevent the development of CrN nitrides and cracks. The current findings demonstrate that temperatures below 400°C combining with a gentle nitriding potential are applicable for obtaining nitrided case without the formation of nitrides and cracks. Noting that pre-annealing at a lower temperature (e.g. 1000°C) which generates lower ferrite content potentially improve the nitriding temperature up to 400°C. On this condition, no evident cracks and CrN nitrides formed, and a relatively high nitriding kinetic is obtained.
Conclusion
Low-temperature gaseous nitriding was performed on annealed 2205 duplex stainless steels. Depending on the various combinations of temperature and nitriding potential applied, nitriding yielded expanded austenite, or a mixture of expanded austenite and expanded ferrite atop the steel.
Precipitation of CrN nitrides upon nitriding is more sensitive to the nitriding temperature than the nitriding potential as well as pre-annealing conditions. Nitriding at a temperature below 400°C produced a precipitation-free nitrided case on 2205 steel.
Combination of a low temperature and a gentle nitriding potential can prevent the development of cracks in the nitrided case. Pre-annealing at a relatively low temperature can improve the threshold of nitriding temperature up to 400°C without cracking.
Pre-annealing at a high temperature can contribute to the nitriding kinetic but promote the formation of nitrides.
Disclosure statement
No potential conflict of interest was reported by the author(s).
Data availability statement
All metadata pertaining to this work will be made available on request.
Additional information
Funding
References
- Bell T. Source book on nitriding. Metals Park, Ohio: ASM International; 1977. p. 266.
- Mittemeijer EJ, Somers MAJ. Thermochemical surface engineering of steels. Cambridge: Woodhead Publishing; 2014.
- Li CX, Bell T. Corrosion properties of active screen plasma nitrided 316 austenitic stainless steel. Corros Sci. 2004;46:1527–1547. doi: 10.1016/j.corsci.2003.09.015
- Christiansen T, Somers MAJ. Low temperature gaseous nitriding and carburising of stainless steel. Surf Eng. 2005;21:445–455. doi: 10.1179/174329405X68597
- Huang R, Wang J, Zhong S, et al. Surface modification of 2205 duplex stainless steel by low temperature salt bath nitrocarburizing at 430°C. Appl Surf Sci. 2013;271:93–97. doi: 10.1016/j.apsusc.2013.01.111
- Zhang ZL, Bell T. Structure and corrosion resistance of plasma nitrided stainless steel. Surf Eng. 1985;1:131–136. doi: 10.1179/sur.1985.1.2.131
- de Oliveira WR, Kurelo BCES, Ditzel DG, et al. On the S-phase formation and the balanced plasma nitriding of austenitic-ferritic super duplex stainless steel. Appl Surf Sci. 2018;434:1161–1174. doi: 10.1016/j.apsusc.2017.11.021
- Li CX. Active screen plasma nitriding - an overview. Surf Eng. 2010;26:135–141. doi: 10.1179/174329409X439032
- Hummelshøj TS, Christiansen TL, Somers MAJ. Towards commercialisation of fast gaseous nitrocarburising of stainless steel. In: Dansk Metall. Selsk. Vintermøde 2010. 2010; p. 105–110.
- Nilsson JO. Super duplex stainless steels. Mater Sci Technol (United Kingdom). 1992;8:685–700. doi: 10.1179/mst.1992.8.8.685
- Gunn R. Duplex stainless steels: microstructure, properties and applications. Cambridge, England: Woodhead Publishing; 1997.
- Li X, Dou W, Tian L, et al. Combating the tribo-corrosion of LDX2404 lean duplex stainless steel by low temperature plasma nitriding. Lubricants. 2018;6:93.
- Tahchieva AB, Llorca-Isern N, Cabrera JM. Duplex and superduplex stainless steels: microstructure and property evolution by surface modification processes. Metals (Basel). 2019;9:347. doi: 10.3390/met9030347
- Bottoli F, Winther G, Christiansen TL, et al. Low-temperature nitriding of deformed austenitic stainless steels with various nitrogen contents obtained by prior high-temperature solution nitriding. Metall Mater Trans A Phys Metall Mater Sci. 2016;47:4146–4159. doi: 10.1007/s11661-016-3559-7
- Kliauga AM, Pohl M. Effect of plasma nitriding on wear and pitting corrosion resistance of X2 CrNiMoN 22 5 3 duplex stainless steel. Surf Coatings Technol. 1998;98:1205–1210. doi: 10.1016/S0257-8972(97)00240-5
- Yan J, Gu T, Qiu S, et al. Phase transformations during the low-temperature nitriding of AISI 2205 duplex stainless steel. Metall Mater Trans B Process Metall Mater Process Sci. 2015;46:1461–1470. doi: 10.1007/s11663-015-0298-x
- Dalton JC, Ernst F, Heuer AH. Low-temperature nitridation of 2205 duplex stainless steel. Metall Mater Trans A Phys Metall Mater Sci. 2020;51:608–617. doi: 10.1007/s11661-019-05553-x
- Larisch B, Brusky U, Spies HJ. Plasma nitriding of stainless steels at low temperatures. Surf Coatings Technol. 1999;116-119:205–211. doi: 10.1016/S0257-8972(99)00084-5
- Haruman E, Sun Y, Adenan MS. A comparative study of the tribocorrosion behaviour of low temperature nitrided austenitic and duplex stainless steels in NaCl solution. Tribol Int. 2020;151:106412. doi: 10.1016/j.triboint.2020.106412
- Tschiptschin AP, Varela LB, Pinedo CE, et al. Development and microstructure characterization of single and duplex nitriding of UNS S31803 duplex stainless steel. Surf Coatings Technol. 2017;327:83–92. doi: 10.1016/j.surfcoat.2017.08.018
- Alphonsa J, Raja VS, Mukherjee S. Study of plasma nitriding and nitrocarburizing for higher corrosion resistance and hardness of 2205 duplex stainless steel. Corros Sci. 2015;100:121–132. doi: 10.1016/j.corsci.2015.07.014
- Chiu LH, Su YY, Chen FS, et al. Microstructure and properties of active screen plasma nitrided duplex stainless steel. Mater Manuf Process. 2010;25:316–323. doi: 10.1080/10426911003748020
- Bobadilla M, Tschiptschin A. On the nitrogen diffusion in a duplex stainless steel. Mater Res. 2015;18:390–394. doi: 10.1590/1516-1439.337714
- Pinedo CE, Varela LB, Tschiptschin AP. Low-temperature plasma nitriding of AISI F51 duplex stainless steel. Surf Coatings Technol. 2013;232:839–843. doi: 10.1016/j.surfcoat.2013.06.109
- Lima JFV, Scheuer CJ, Brunatto SF, et al. Kinetics of the UNS S32750 super duplex stainless steel low-temperature plasma nitriding. Mater Res. 2022;25:17–28.
- Li XY, Roberts R, Dou WB, et al. Low temperature plasma surface alloying and characterisation of a superduplex stainless steel. Int Heat Treat Surf Eng. 2014;8:61–64. doi: 10.1179/1749514813Z.00000000090
- Pintaude G, Rovani AC, das Neves JCK, et al. Wear and corrosion resistances of active screen plasma-nitrided duplex stainless steels. J Mater Eng Perform. 2019;28:3673–3682. doi: 10.1007/s11665-019-04114-y
- Wang B, Christiansen TL, Somers MAJ. Low-temperature gaseous nitriding of 2205 duplex stainless steel: effect of temperature and nitriding potential. In: ESSC DUPLEX Conf., Bardolino, Italy; 2022.
- TMR Stainless. Practical guidelines for the fabrication of duplex stainless steels. London: International Molybdenum Association; 2014.
- Wang B, Christiansen TL, Somers MAJ. Influence of ferrite-austenite distribution in 2205 duplex stainless steel on high-temperature solution nitriding behaviour. Surf Coatings Technol. 2023;453:129134. doi: 10.1016/j.surfcoat.2022.129134
- Somers MAJ, Lankreijer RM, Mittemeijer EJ, et al. Excess nitrogen in the ferrite matrix of nitrided binary iron-based alloys. Philos Mag A Phys Condens Matter Struct Defects Mech Prop. 1989;59:353–378.