Abstract
Feline infectious peritonitis (FIP) is a fatal illness caused by a mutated feline coronavirus (FCoV). This disease is characterized by its complexity, resulting from systemic infection, antibody-dependent enhancement (ADE), and challenges in accessing effective therapeutics. Extract derived from Vigna radiata (L.) R. Wilczek (VRE) exhibits various pharmacological effects, including antiviral activity. This study aimed to investigate the antiviral potential of VRE against FCoV, addressing the urgent need to advance the treatment of FIP. We explored the anti-FCoV activity, antiviral mechanism, and combinational application of VRE by means of in vitro antiviral assays. Our findings reveal that VRE effectively inhibited the cytopathic effect induced by FCoV, reduced viral proliferation, and downregulated spike protein expression. Moreover, VRE blocked FCoV in the early and late infection stages and was effective under in vitro ADE infection. Notably, when combined with VRE, the polymerase inhibitor GS-441524 or protease inhibitor GC376 suppressed FCoV more effectively than monotherapy. In conclusion, this study characterizes the antiviral property of VRE against FCoV in vitro, and VRE possesses therapeutic potential for FCoV treatment.
Introduction
Feline coronavirus (FCoV) is a single-strand RNA virus in the alphacoronavirus genus with a 29 kb genome. It encodes the spike protein (S), envelope protein (E), nucleocapsid protein (N), membrane protein (M), and other non-structural proteins (Dye and Siddell Citation2007). The S protein binds to the cell receptors, facilitating viral entry via endocytosis or fusion. The viral genome is then released into the cytosol for replication and protein synthesis. Post-translational modifications, such as glycosylation of the S, M, and E proteins, occur in the endoplasmic reticulum. Virus particles are assembled in the ER-Golgi intermediate compartment and released via exocytosis (Jaimes and Whittaker Citation2018). The global prevalence of FCoV varies from 20 to 95%, as reported in several studies (Kummrow et al. Citation2005; Amer et al. Citation2012; Klein-Richers et al. Citation2020; Yen and Chen Citation2021; Zhou et al. Citation2021; Amoroso et al. Citation2022). FCoV comprises two biotypes: feline enteric coronavirus (FECV) and feline infectious peritonitis coronavirus (FIPV). FECV usually causes mild gastrointestinal symptoms, whereas FIPV often leads to serious systemic infection due to its cell tropism for monocytes and macrophages (Rottier et al. Citation2005). FECV is transmitted through the fecal-oral route and can infect domestic and wild cats, with a positive rate of 90% in multi-cat households. However, only 5% of infections spontaneously mutate into FIPV, resulting in the development of fatal feline infectious peritonitis (FIP) (Kipar and Meli Citation2014).
FIP is considered intractable owing to its high mortality rate and the challenges in diagnosis, prevention, and treatment. Previous attempts to develop an effective vaccination were unsuccessful because non-neutralizing antibodies not only failed to provide protection but also exacerbated the illness through antibody-dependent enhancement (ADE), regardless of whether the infection was caused by the same or a different serotype of FIPV (Hohdatsu et al. Citation1998; Takano et al. Citation2008). In recent years, there have been some breakthroughs in the development of FIPV therapeutics, including the antiviral drugs GS-441524 and GC376 (Kim et al. Citation2015; Perera et al. Citation2019; Delaplace et al. Citation2021; Coggins et al. Citation2023; Green et al. Citation2023). GS-442524 is an adenosine analog that inhibits polymerase and reduces viral load and symptoms in clinical studies (Pedersen et al. Citation2019; Addie et al. Citation2020; Coggins et al. Citation2023; Green et al. Citation2023; Zwicklbauer et al. Citation2023). GC376 is a 3CL-protease inhibitor, and in vivo studies have revealed its efficacy in symptom remission in FIP cases (Kim et al. Citation2015; Perera et al. Citation2019). Furthermore, GC376 blocked SARS-CoV-2 and led to the discovery of more potent inhibitors (Cáceres et al. Citation2021; Pang et al. Citation2023; Tan et al. Citation2023). Nevertheless, relapse and drug resistance have occurred in both GS-441524 and GC376 treatments (Murphy et al. Citation2018; Pedersen et al. Citation2018, Citation2019), and there are still challenges in feline patient care, including issues related to drug accessibility, cost, adverse effects, and the lengthy duration of treatment protocols (Jones et al. Citation2021).
Vigna radiata (L.) R. Wilczek, commonly known as mung bean, is a traditional medicine with numerous health benefits, including antihypertensive, anticancer, and anti-inflammatory properties (Hou et al. Citation2019). Furthermore, its antiviral activity has been explored in our prior research. The extract derived from the seed coats of V. radiata (L.) R. Wilczek (VRE) inhibited influenza A virus in vitro through various mechanisms, including the blockade of viral entry, interference with assembly, and the repression of viral release (Lo et al. Citation2020). Considering the urgent need for effective antivirals to combat FIP, this study investigated VRE's inhibitory effect against FIPV and its underlying antiviral mechanism. Additionally, due to the complex pathogenic mechanism of FIPV, cocktail therapy combining VRE with other previously used therapeutic compounds was evaluated.
Materials and methods
Compounds
Vigna radiata (L.) R. Wilczek extract (VRE) was provided by King’s Ground Biotech Co., Ltd. (Pingtung, Taiwan), following the preparation process outlined in the previous study, and the major components of VRE are vitexin and isovitexin (Lo et al. Citation2020). VRE was dissolved in DMSO and stored at −20 °C until use. GS-441524 (MedChemExpress) and GC376 (AdooQ Bioscience) were dissolved in DMSO and stored at −20 °C until use.
Cells
Fcwf-4 (ATCC #CRL-2827) is a macrophage cell line isolated from cat embryos with peritonitis. CrFK (ATCC #CCL-94) is a cell line isolated from cat cortical kidney tissue. Fcwf-4 and CrFK were maintained in DMEM (Gibco) containing 10% FBS (Gibco) and 1% PSA (Sigma-Aldrich) at 37 °C under 5% CO2. Feline peripheral blood mononuclear cells (PBMCs) were isolated from 50 ml of cat blood collected in CPDA-1 solution (JMS) (Ethical permission ID: NTU-109-EL-00088). For PBMC isolation, Histopaque-1077 (Sigma Aldrich) was used, following the instructions provided by the manufacturer. PBMCs were resuspended in DMEM containing 10% FBS and 1% PSA and maintained at 37 °C and 5% CO2. HEK293-UU4-S are HEK293 cells that stably express the spike protein ectodomain of the FIPV strain UU4 (serotype I FCoV) (Yang et al. Citation2020b). HEK293-UU4-S cells were maintained in DMEM containing 10% FBS, 1% PSA, and 1.5% Geneticin (Invitrogen) at 37 °C under 5% CO2.
Viruses
Feline coronavirus NTU156 (type II FCoV strain) was kindly provided by Professor Ling-Ling Chueh at the National Taiwan University. The virus was propagated and titrated in Fcwf-4 cells using a plaque assay. In the plaque assay, Fcwf-4 cells (2 × 105/well) were seeded in 12-well plates one day before the experiment. Cells were incubated with serially diluted virus for 1 h. The cells were washed with PBS and incubated with 1 ml of 1.8% methylcellulose in DMEM for two days. The plates were fixed with 10% formalin and stained with 1% crystal violet. The plaque-forming unit (PFU) per ml of the virus was determined by counting viral plaques.
Cytotoxicity test
To confirm the safety of VRE in different tissues and in in vitro or ex vivo models, the cytotoxicity of VRE was examined in Fcwf-4, CrFK, and feline PBMCs. The cells were seeded in 96-well plates (2 × 104 cells/well) one day before the experiment. The cells were incubated with serially diluted VRE with a DMSO final concentration of 2%. After 3 days, cell viability was analyzed using a CCK-8 assay (Dojindo). The cells were incubated with a medium containing 2% DMSO, representing 100% cell viability, and the relative viability of the treated cells was counted. The concentration of cytotoxicity 50% (CC50) was determined by fitting the data onto a non-linear regression curve using Prism 9 (GraphPad Software).
Cytopathic effect reduction test
Fcwf-4 cells (2 × 104/well) were seeded in 96-well plates one day before the experiment. The cells were incubated with serially diluted VRE with a DMSO final concentration of 2% for 1 h and then infected with FIPV NTU156 (MOI = 0.01 or 0.001) for 1 h. After virus removal, the cells were washed and incubated with the same concentration of VRE. At 24 h post-infection (hpi), the cell viability was determined using the alamarblue assay (Bio-Rad). Infected cells incubated with 2% DMSO represented 0% viability, while uninfected cells incubated with 2% DMSO represented 100% viability. The viability of the experimental wells was calculated using the following formula: 1 − (the mean of OD570-OD600 from uninfected controls − the mean of OD570-OD600 from experimental wells)/(the mean of OD570-OD600 from uninfected controls − the mean of OD570-OD600 from untreated controls). The half-maximum inhibitory concentration (IC50) was estimated using a non-linear regression model using Prism 9.
Western blotting analysis
The cell lysates were sonicated and centrifuged at 10,000 × g for 10 min at 4 °C to remove cell debris. The supernatants were loaded onto a 10% SDS-PAGE gel (Bio-Rad) with 6X reducing buffer (T-Pro Biotechnology), and the proteins were transferred onto NC membranes (Bio-Rad). The membranes were blocked with 5% skim milk (BD Biosciences). To analyze the FCoV N protein, the mouse anti-FCoV N protein mAb (1:2000, Bio-Rad MCA2194) was used as a primary antibody. For analyzing the FCoV S protein, the cat serum (#F108215) obtained from clinical cases (Ethical permission ID: NTU-109-EL-00088) was used as a primary antibody (1:500 diluted). GAPDH (1:5000, ThermoFisher AM4300) was used as the loading control antibody. Goat anti-mouse IgG HRP conjugate (1:2000, Jackson ImmunoResearch) was used as the secondary antibody for N protein and GAPDH analysis. Goat anti-cat IgG HRP conjugate (1:2000, Bethyl Laboratories A20-120P) was used as the secondary antibody for S protein analysis. The protein signals were developed using an ECL detection kit (Bio-Rad) and visualized using the ChemiDoc XRS + System and Image Lab Software (Bio-Rad).
Quantitative RT-PCR (RT-qPCR)
Viral RNA was extracted using a viral nucleic acid extraction kit (Geneaid Biotech Ltd.). For M-N protein gene analysis, cDNA was synthesized using primer P1b, and the M-N protein gene was detected using qPCR using primers P009, P010, and probe P9/10, and the cycling program followed directions from a previous study (Hu et al. Citation2017). For S protein gene analysis, the cDNA was synthesized using oligo(dT), and it was analyzed using qPCR with primers nIcfs, nIubs, and SYBR Green (Addie et al. Citation2003). The cycling program was as follows: preheating at 95 °C for 2 min; 40 cycles of denaturation at 95 °C for 5 s; and primer annealing and extension at 55 °C for 30 s. Primers (28S-F and 28S-R) that detect 28S ribosomal mRNA were used to normalize mRNA expression. The cycling program was as follows: preheating at 95 °C for 2 min; 40 cycles of denaturation at 95 °C for 5 s; and primer annealing and extension at 60 °C for 10 s. The viral S gene was normalized to the 28S housekeeping gene as follows: ΔCt = Ct (S gene) − Ct (28S). The fold change compared to the untreated group was determined.
Immunofluorescence assay (IFA)
The cells were fixed with 4% paraformaldehyde, permeabilized with 0.3% Triton X-100, and blocked with 5% goat serum (Jackson ImmunoResearch). For N protein analysis, the mouse anti-FCoV N monoclonal antibody (Bio-Rad MCA2194) was used as a primary antibody (1:1000), while mAb 6-4-2 (kindly provided by Dr. Tomomi Takano and Dr. Tsutomu Hohdatsu at Kitasato University, Japan) was used as a primary antibody (1:5) for S protein analysis. The mAb 6-4-2 (IgG2a) recognizes the serotype II FCoV S protein, and the hybridoma supernatant was used in this experiment (Hohdatsu et al. Citation1991). The FITC-conjugated goat anti-mouse IgG (1:1000, Jackson ImmunoResearch, 115-095-003) was used as the secondary antibody. Nuclei were stained with DAPI (1:500, Invitrogen). Fluorescence signals were detected using the microscope (Olympus IX-83).
Antiviral activity test
Fcwf-4 cells (2 × 105/well) were seeded in 12-well plates one day before the experiment. FIPV NTU156 (MOI = 0.001) and Fcwf-4 cells were treated with serially diluted VRE with a DMSO final concentration of 2% simultaneously for 1 h. After incubation, the cells were infected with the VRE-virus mixture for 1 h. The cells were washed with PBS and incubated with serially diluted VRE for 24 h. The CPE was observed under the microscope, the supernatants were collected for titration using plaque assays, and the viral titers of each group relative to the untreated control (cells incubated with medium containing 2% DMSO) were calculated. The cells were collected for protein analysis using Western blotting.
Virucidal activity assay
To evaluate the virucidal ability of VRE against FIPV, 1200 μg/ml of VRE (CC10) was incubated with 10,000 PFU FIPV at 37 °C for 1 h. After incubation, the mixture was diluted and titrated using plaque assays.
Time-of-addition assay (ToA assay)
VRE was added in different infection states to investigate its antiviral mechanism. The following assay was conducted according to a previous study (Lo et al. Citation2020) with some modifications. Fcwf-4 cells (2 × 105/well) were seeded in 12-well plates overnight. FIPV NTU156 (MOI = 0.001 or 1) was treated with 1200 μg/ml of VRE (CC10) under six conditions. (1) Pretreated virus: FIPV was treated with VRE for 1 h, followed by infecting cells for 1 h. The cells were washed with PBS and incubated with fresh medium for 11 h. (2) Pretreated cell: The cells were incubated with VRE for 1 h before FIPV infection. After the infection, the cells were washed and incubated with a fresh medium for 11 h. (3) Pretreated cell + wash: The cells were incubated with VRE for 1 h. After incubation, the cells were washed with PBS, followed by FIPV infection for 1 h. The cells were washed and incubated with fresh medium for 11 h. (4) Treated infection + wash: VRE was added during FIPV infection. After 1 h of infection, the cells were washed and incubated with the fresh medium for 11 h. (5) After infection: The cells were infected with FIPV for 1 h and incubated with VRE for 11 h. (6) All: FIPV was preincubated with 1200 μg/ml VRE for 1 h. Simultaneously, Fcwf-4 cells were treated with 1200 μg/ml VRE for 1 h. After incubation, the cells were infected with the VRE-virus mixture for 1 h. The virus-containing medium was removed, and the cells were incubated with VRE for 11 h.
Another time-of-addition assay, where VRE was added at different time points during FIPV infection, was conducted to explore the detailed antiviral mechanism of VRE. The Fcwf-4 cells were infected with FIPV (MOI = 0.001), and 1200 μg/ml of VRE was added at 0, 2, 4, 6, and 8 hpi. The supernatants of all treatment groups were collected, and the viral titers were determined using plaque assays.
FIPV entry assay
The Fcwf-4 cells (2 × 104/well) were seeded in 96-well plates one day before the experiments. The cells were infected with FIPV NTU 156 (MOI = 0.1) for 1 h at 4 °C for virus binding to the cell surface but did not enter the cells. After 1 h of viral attachment, the cells were incubated at 37 °C for virus entry and treated with 1200 μg/ml of VRE for 1 h. N protein expression was analyzed using IFA at 8 hpi.
Spike protein expression analysis
The Fcwf-4 cells (2 × 104/well) were seeded in 96-well plates one day before experiments. The cells were infected with FIPV NTU 156 (MOI = 0.1) for 1 h at 37 °C and treated with 600 or 1200 μg/ml of VRE at 3–8 hpi, which is the late infection state. The expression of S protein was examined using IFA, whereas that of S mRNA was determined using RT-qPCR at 8 hpi. In the virus-free model, the HEK293-UU4-S cells that stably expressed S protein were incubated with 1200 μg/ml of VRE for 24 h at 37 °C. The cells were collected, and S protein expression was determined using Western blotting.
VRE antiviral activity assay under in vitro ADE infection
The ADE infection model was conducted according to a previous study (Hu et al. Citation2017). To establish the ADE model that induced a significantly increased viral titer, different dilution factors of mAb 6-4-2 (1:2.5, 1:5, 1:10, and 1:20) were mixed with FIPV NTU156 (MOI = 0.0035) in a 1:1 ratio at 4 °C for 1 h on a rotator. After incubation, the cells were inoculated with 200 μl of the virus–antibody mixture for 3 h at 37 °C. After infection, the cells were washed and incubated with the fresh medium. The supernatants were collected at 48 hpi, and the viral titer was determined using RT-qPCR. To verify VRE anti-FIPV activity under the ADE model, diluted mAb 6-4-2 was mixed with FIPV NTU156 (MOI = 0.0035) in a 1:1 ratio at 4 °C for 1 h. Simultaneously, the Fcwf-4 cells (2 × 105/well in a 12-well plate) were incubated with VRE for 1 h at 37 °C. After incubation, the cells were inoculated with 200 μl of the virus–antibody mixture. The cells were washed after 3 h of infection and incubated with 1200 μg/ml of VRE for 48 h. The viral titer was determined using RT-qPCR.
Combinational use of compounds against FIPV
The Fcwf-4 cells (2 × 104/well) were seeded in 96-well plates one day before experiments. We examined the synergistic effect and cytotoxicity of combining VRE with GS-441524 or VRE with GC376. Each drug was screened at six different concentrations, and the diagonal pairs of the full dose–combination matrix were tested. The cells were treated with the compound mixture at 37 °C for 1 h, and FIPV NTU156 (MOI = 0.01) was incubated with the same compound mixture simultaneously. The cells were infected with the virus-containing compound mixture for 1 h and were washed after virus removal, followed by incubation with the same concentration of the compound mixture for 24 h. Cell viability was validated by means of alamarblue assay, and the supernatants were collected for RT-qPCR analysis. The full drug response matrices were predicted using the DECREASE web application (Ianevski et al. Citation2019). The interaction of drug combinations was quantified based on the ZIP, Bliss, Loewe, and HSA reference model using SynergyFinder 3.0 web application (Berenbaum Citation1989; Ianevski et al. Citation2022).
Statistical analyses
The data were analyzed using unpaired t-tests or ANOVA using Dunnett’s multiple comparison tests using Prism 9. *p < 0.05, **p < 0.01, ***p < 0.001, and ****p < 0.0001 were considered significant.
Results
Cytotoxicity of V. radiata extract
As indicated in , the cytotoxicity of VRE in Fcwf-4, CrFK, and PBMC was measured using CCK-8 assays after 3 days of VRE incubation. The CC50 of VRE is 4539 μg/ml in Fcwf-4 cells, 1875 μg/ml in CrFK, and 4994 μg/ml in PBMC.
Vigna radiata extract dose-dependently inhibited FIPV
As shown in , the cells and viruses were pretreated with VRE, and VRE was also added during the entire infection period. In , the cells infected by FIPV detached or showed multinucleated giant cells in the untreated group. The CPE was reduced when the infected cells were treated with 300 or 600 μg/ml of VRE, whereas nearly no CPE was observed in the infected cells treated with 1200 μg/ml of VRE. The cytopathic effect reduction ability of VRE in indicated that the IC50 value was 65.8 μg/ml (MOI = 0.001) and 235.1 μg/ml (MOI = 0.01). In , the virus titer of each treated group was measured using plaque assays and Western blot analysis. VRE inhibited virus replication in a dose-dependent manner, and the Western blot analysis in indicated that the intracellular expression of FIPV N protein (50–56 kDa) significantly decreased under 1200 μg/ml of VRE treatment.
Figure 2. Antiviral activity of VRE against FIPV. (A) VRE was added during FIPV infection, and the antiviral activity of VRE was verified at 24 hpi using CPE analysis, alamarblue assay, Western blotting, and plaque assay. (B) Images of infected Fcwf-4 cells were observed at 24 hpi. Uninfected cells were used as controls. (C) The CPE reduction activity of VRE against FIPV was determined using alamarblue assay. Data in the plot represents the mean ± SD of three replicates. (D) Viral titers in the culture supernatant were determined using plaque assays. Virus titers between each treated group and the untreated control group were compared using one-way ANOVA followed by Dunnett’s multiple comparisons tests (ns: non-significant; ****p < 0.0001; **p < 0.01). Values are the mean ± SD of three replicates. (E) Western blotting analysis of FIPV N protein. GAPDH is the housekeeping protein used as the loading control.
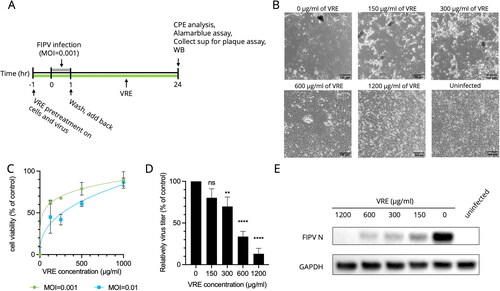
The virucidal activity of V. radiata extract against FIPV was limited
presents the experimental design for clarifying the virucidal ability of VRE. VRE (1200 μg/ml) was incubated with 10,000 PFU of FIPV for 1 h at 37 °C, and the virus was titrated using plaque assay. As shown in , the viral titer of the VRE-treated and untreated groups was not significantly different, implying that the virucidal activity of VRE against FIPV was limited, and further assays were conducted to explore its inhibitory mechanism.
Figure 3. VRE anti-FIPV activity in different infection states. (A) Schematic diagram of the VRE virucidal activity assay. VRE (1200 μg/ml) was incubated with 10,000 PFU FIPV at 37 °C for 1 h, and the viral titer was determined using plaque assay. The VRE-FIPV mixture was inoculated to the Fcwf-4 cells for 1 h, and the cells were fixed and stained with crystal violet at 48 hpi. (B) The viral titer between the VRE-treated and untreated groups was compared using unpaired t-tests (ns: non-significant). (C) VRE anti-FIPV mechanism was analyzed by adding 1200 μg/ml of VRE in six different infection states. At 12 hpi, the supernatants were collected, and viral titers were determined using plaque assay. (D) Virus titers between each treated and untreated control group were compared using one-way ANOVA followed by Dunnett’s multiple comparisons tests (ns: non-significant; **p < 0.01; *p < 0.05). Data are shown as mean ± SD (n = 3). (E) The representative images of cells treated/untreated with 1200 μg/ml of VRE under infection of MOI = 1 for 12 h. (F) VRE anti-FIPV mechanism was analyzed by adding 1200 μg/ml of VRE at five time points: 0, 2, 4, 6, and 8 hpi. The supernatants were collected at 12 hpi for titration. (G) Virus titers in the culture supernatant were determined using plaque assays. The virus titers between each treated group and the untreated control group were compared using one-way ANOVA followed by Dunnett’s multiple comparisons tests (***p < 0.001; **p < 0.01; *p < 0.05). Data are shown as mean ± SD (n = 3).
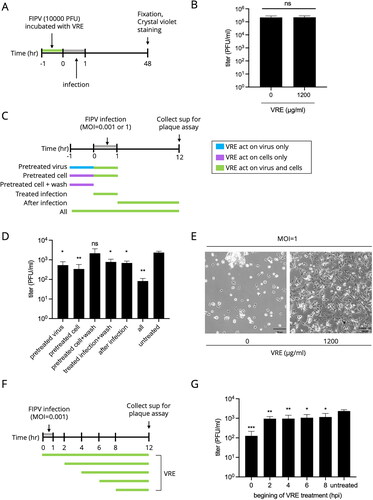
Vigna radiata extract reduced FIPV activity in the early and late infection phases
illustrates assays that investigated the inhibitory mechanism of VRE against FIPV. showed antiviral activity when VRE was added in different infectious states under the infection of MOI = 0.001. The ‘pretreated virus’ treatment group indicated decreased viral titer when compared to the untreated group, implying that VRE may interfere with the interaction of viral attachment. The ‘pretreated cell’ treatment significantly reduced viral titer, indicating that VRE may act on cell receptors and, thus, interfere with virus-cell interactions during viral attachment. However, in the ‘pretreated cell + wash’ group, the viral titer was not significantly different from the untreated group, suggesting that VRE did not have a prophylactic effect against FIPV. The ‘treated infection + wash’ treatment reduced virus replication, meaning VRE might interfere with virus-cell interactions during viral attachment. The virus titer in ‘after infection’ treatment decreased, suggesting that VRE might act in the late stage of the infection. The ‘all’ group showed a significantly lower virus titer than the untreated group. To sum up, VRE reduced FIPV activity in both early and late infection phases. Additionally, the antiviral activity of VRE under a high infection dose was investigated. The Fcwf-4 cells were infected with FIPV (MOI = 1) and treated with VRE (1200 μg/ml) during the entire infection period. The images shown in exhibit that VRE provided CPE protection under a MOI = 1 infection for 12 h; however, the infection of MOI = 1 was too virulent against Fcwf-4 cells and resulted in difficulty in exploring the anti-FIPV mechanism of VRE.
As depicted in , an assay was conducted to examine which phase of the viral lifecycle is inhibited by VRE by adding VRE at five different time points in a single replication cycle. In , the virus titer significantly decreased when the VRE was added at 0, 2, 4, 6, and 8 hpi. The most pronounced antiviral effect was observed when VRE was added at 0 hpi, which is the earliest stage of the infection cycle. Furthermore, virus replication was blocked when VRE was added at 8–12 hpi, implying that VRE moderately inhibited the late stages of the infection, such as assembly and release.
The FIPV intracellular entry inhibition activity of V. radiata extract was limited
As shown in , an FIPV entry assay was conducted considering the anti-FIPV ability of VRE in the early infection state. The Fcwf-4 cells were inoculated with FIPV and incubated at 4 °C for viral attachment. After attachment, the cells were shifted to 37 °C for viral entry and treated with VRE (1200 μg/ml) for 1 h. The antiviral activity was determined at 8 hpi by examining N protein expression using IFA. As the represented IFA images shown in , the N protein expression between the VRE-treated and untreated groups was not significantly different, suggesting that the intracellular entry inhibition ability of VRE was limited.
Figure 4. Entry test of VRE against FIPV. (A) Fcwf-4 cells were incubated with FIPV at 4 °C for virus binding for 1 h. After viral binding, the cells were incubated at 37 °C and treated with VRE for 1 h. IFA analysis was conducted at 8 hpi to analyze N protein expression. (B) Representative immunofluorescence images of different concentrations of VRE. FITC (green) signals represent N protein, whereas DAPI (blue) represents the nuclei (magnification: 100×).
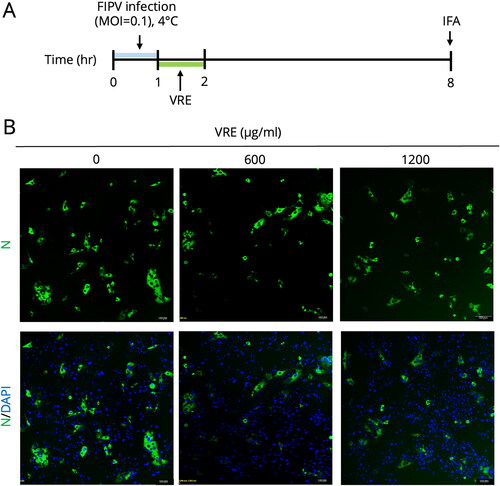
Vigna radiata extract decreased the production of S protein
In , as VRE showed inhibition against FIPV during the late stage of infection, and VRE is known to have α-glucosidase inhibition activity (Lo et al. Citation2020), we considered that VRE might affect the production of S protein, which is highly glycosylated when adding VRE in the late phase of a single viral replication cycle (3–8 hpi). In the IFA images shown in , S protein production was reduced when treated with 600 and 1200 μg/ml of VRE, whereas in , S mRNA expression was not significantly different between infected cells with or without VRE treatment. In the virus-free cell model, we explored whether VRE affected S protein expression (), where HEK293-UU4-S cells stably expressing FIPV S protein were incubated with VRE for 24 h, and S protein expression was assessed using Western blot. The result in indicated that the S protein expression of HEK293-UU4-S cells decreased when the cells were treated with VRE for 24 h.
Figure 5. FIPV spike protein analysis. (A) Schematic diagram of testing for VRE inhibition of spike protein. Fcwf-4 cells were infected for 1 h, and VRE was added at 3–8 hpi. IFA and RT-qPCR were performed at 8 hpi to measure S protein expression or mRNA. (B) Representative immunofluorescence images of VRE treated in the late phase of FIPV infection. FITC (green) signals represent S protein, whereas DAPI (blue) represents the nuclei (magnification: 100×). (C) RT-qPCR results were used to quantify S mRNA expression. Data are shown as mean ± SD (n = 3). The S gene mRNA expression between the treated and untreated groups was compared using unpaired t-tests (ns: non-significant). (D) Schematic diagram of the S protein inhibitory effect of VRE when incubated with S protein stably expressing HEK293-UU4-S cells. (E) Western blotting analysis of FIPV S protein in HEK293-UU4-S cells. GAPDH is the housekeeping protein used as the loading control.
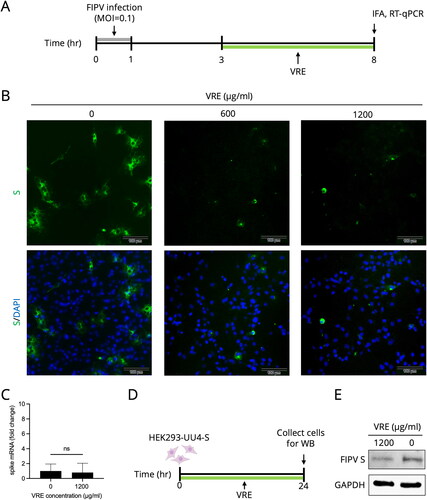
Vigna radiata extract blocked FIPV under the in vitro ADE model
As ADE is a severe phenomenon in FIP, we aimed to confirm the antiviral ability of VRE against FIPV under ADE infection (). We tested the virus titer using different dilution factors of mAb 6-4-2 in the FIPV infection and the dilution of 1:10 induced a more significant titer difference between infection with/without antibody (data not shown); therefore, the ADE infection model was conducted using 1:10 diluted mAb 6-4-2. The results in indicate that when VRE was added during ADE infection, the virus titer decreased significantly compared to that of the untreated group, in a dose-dependent manner.
Figure 6. VRE anti-FIPV activity in the in vitro ADE model. (A) Experimental design to examine the inhibitory activity of VRE on FIPV under ADE infection conditions. Different concentrations of VRE were added during ADE infection, and the viral titer was determined using RT-qPCR. (B) The viral titers between the treated and untreated groups were compared using one-way ANOVA followed by Dunnett’s multiple comparisons tests (ns: non-significant; **p < 0.01; *p < 0.05). Data are shown as mean ± SD (n = 3).
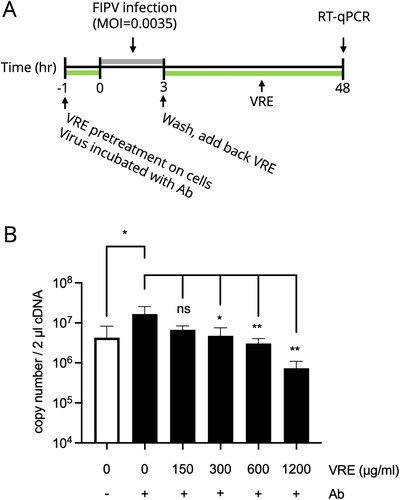
The combinational use of V. radiata extract and GS-441524 in FIPV-infected cells is additive
As shown in , we tested the synergy of VRE combined with GS-441524 since GS-441524 is an RNA-dependent RNA polymerase inhibitor that shows FIPV inhibition in previous studies. The combination treatments did not cause severe cytotoxicity (Supplementary Figure 1(A)). As shown in , the CPE inhibition of the diagonal elements of the dose–response matrix was examined, and the full dose–response matrix was predicted using the DECREASE model. Combined treatment with VRE and GS-441524 provided better CPE protection than monotherapy. Moreover, the virus was eliminated when treated with 500 μg/ml of VRE and 2.5 μM of GS-441524 (Supplementary Figure 1(B)). The synergistic effect () calculated using SynergyFinder showed that the mean synergy score was 6.52 (ZIP), 10.48 (Loewe), 6.67 (Bliss), and 12.16 (HSA), suggesting that the interaction between VRE and GS-441524 is additive.
Figure 7. Combinational use of VRE and GS-441524 or GC376 on anti-FIPV activity. (A) Combinations of different concentrations of VRE and GS-441524 were tested during FIPV infection. (B) Dose–response matrix of the combination of VRE and GS-441524. The diagonal elements of the full dose–response matrix were examined (left), and the full dose–response matrix was predicted using the DECREASE model. (C) ZIP, Loewe, Bliss, and HSA score maps for the combination of VRE and GS-441524. A synergy score below −10 indicates an antagonistic interaction between the drugs. Scores ranging from −10 to 10 suggest an additive interaction, whereas scores above 10 indicate a synergistic interaction. (D) Different concentrations of VRE and GC376 were combined during FIPV infection. (E) Dose–response matrix of the combination of VRE and GC376. The diagonal elements of the full dose–response matrix were examined (left), and the full dose–response matrix was predicted using the DECREASE model. (F) ZIP, Loewe, Bliss, and HSA score maps for the combination of VRE and GC376. A synergy score below −10 suggests antagonism between the drugs. Scores within −10 to 10 imply an additive effect, and scores above 10 indicate synergy.
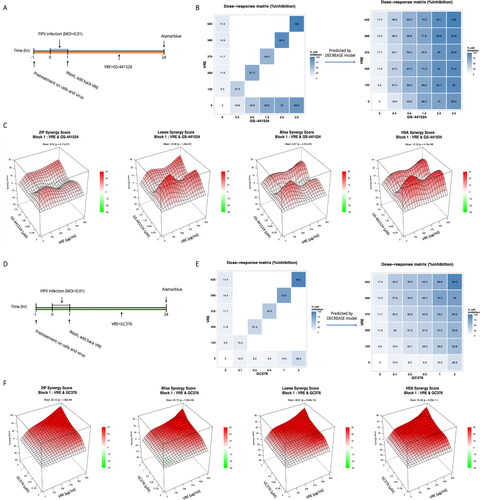
The combinational use of V. radiata extract and GC376 in FIPV-infected cells is synergistic
In , we verified the synergy of VRE combined with GC376 because GC376 is a 3 C-like protease inhibitor that blocks FIPV in clinical cases (Kim et al. Citation2015; Perera et al. Citation2019). The combination treatments did not cause severe cytotoxicity (Supplementary Figure 1(C)). As shown in , the CPE inhibition of the diagonal elements of the dose–response matrix was examined, and the full dose–response matrix was predicted using the DECREASE model. The combined therapy of VRE and GC376 inhibited FIPV-induced CPE more effectively than monotherapy; furthermore, virus replication was abolished when combining 500 μg/ml of VRE with 1.6 μM GC376 (Supplementary Figure 1(D)). The synergistic effect calculated by SynergyFinder indicated that the mean synergy score was 23.12 (ZIP), 22.71 (Bliss), 28.61 (Loewe), and 31.46 (HSA), suggesting that the interaction between VRE and GC376 is synergistic ().
Discussion
This study elucidated the in vitro antiviral activity of VRE against FIPV. It was observed that VRE conferred protection to cells from CPE and impeded virus replication. This inhibition was achieved through interference with viral attachment during the early phase of infection and a reduction in spike glycoprotein production in the later phase. As the major ingredients of VRE are vitexin and isovitexin, and VRE is known to have α-glucosidase inhibition activity (Lo et al. Citation2020), we assumed that the ability of VRE to reduce S protein expression may involve hindering protein maturation processes, such as glycosylation, leading to improper assembly and reduced virus production. Furthermore, despite VRE demonstrating enhanced inhibition in the early stages of the viral replication cycle, it does not appear to interfere with FIPV intracellular entry. This observation aligns with the characteristics of other flavonoids, such as isoginkgetin and luteolin, which have been reported to inhibit FIPV in the early replication stages but do not block virus entry (Triratapiban et al. Citation2023).
ADE is a sophisticated and serious problem in FIPV infection, where the presence of sub-neutralizing antibodies that recognize viral spike proteins facilitates virus infection. In addition to increased virus yield, elevated cytokine expression in the ADE model has been reported. One study showed that TNF-α production increased in primary alveolar macrophages inoculated with a mixture of FIPV and mAb 6-4-2 (Takano et al. Citation2007). In our study using Fcwf-4 cells as the cellular model, however, TNF-α levels did not change before and after virus infection (data not shown), indicating that the TNF-α production between in vitro and ex vivo models of macrophages was different. Therefore, only the virus titer, not the TNF-α level, was examined in the antiviral activity of VRE in the ADE model.
Cocktail therapies are applied to many diseases owing to their sophisticated pathogenesis. In the drug combination antiviral test against FIPV, we mixed VRE with GS-441524 or GC376. The combination of 625 μg/ml of VRE and 3.5 μM of GS-441524 completely prevented FIPV-induced CPE and similar antiviral activity was observed in the treatment of 625 μg/ml of VRE combined with 2 μM of GC376. Furthermore, the IC50 of GS-441524 is 3.5 μM and 0.4–2 μM for GC376, whereas 4 μM of GS-441524 and 10 μM of GC376 completely inhibited FIPV infection in vitro (Ke et al. Citation2020; Yang et al. Citation2020a). This suggests that our two combination therapies for FIPV infection can block the virus more effectively than monotherapy with GS-441524 or GC376. In addition, GC376 resistance in naturally FIPV-infected cats has been documented (Pedersen et al. Citation2018), and resistance to GS-441524 presumably occurs in both naturally and experimentally FIPV-infected cats (Murphy et al. Citation2018; Pedersen et al. Citation2019). Risk factors for antiviral resistance occurrence include prolonged use, monotherapy, and inappropriate dosing (Vitiello Citation2022). As our combination treatments completely block virus replication under dosages lower than monotherapy, they may shorten the treatment time and reduce the risk of drug resistance. Furthermore, as GS-441524, GC376, and flavonoids follow different metabolic pathways and are metabolized by distinct enzymes, VRE is not expected to compete with GS-441524 or GC376, thereby supporting the feasibility of the combination treatments used in this study (Kim et al. Citation2016; Murota et al. Citation2018; Murphy et al. Citation2018; Pedersen et al. Citation2018; Chandel Citation2021).
Toward potential clinical use, the cytotoxicity of VRE was explored in cell lines derived from different tissues and feline PBMCs, providing evidence of VRE safety in both in vitro and ex vivo feline models. We believe that administering VRE to cats is possible because mung beans can be a feed additive for many animals (Lee et al. Citation2017; Al-Homidan et al. Citation2020). Moreover, our study established antiviral testing models that offer platforms for drug screening and can be utilized to develop novel antiviral agents against FIPV. Further studies should be conducted to validate the pharmacokinetics and pharmacodynamics of VRE, as well as its efficacy in treating clinical cases. In conclusion, the in vitro antiviral effect of VRE on FIPV was demonstrated in our study and has the potential to serve as a cocktail therapeutic protocol with current antiviral agents.
Author contributions
HWC (Chen) conceived and designed the experiments. AAC, YCL, CCP, YMK, and HFC performed the experiments and analyzed the data. YCC, HWC (Chang), and CHL contributed essential reagents and comments. AAC drafted the manuscript. HWC and CHL edited the manuscript. All authors read and approved the final manuscript.
Supplemental Material
Download MS Word (182.5 KB)Disclosure statement
No potential conflict of interest was reported by the author(s).
Data availability statement
The data that support the findings of this study are available from the corresponding author, upon reasonable request.
Additional information
Funding
References
- Addie DD, Curran S, Bellini F, Crowe B, Sheehan E, Ukrainchuk L, Decaro N. 2020. Oral Mutian(R)X stopped faecal feline coronavirus shedding by naturally infected cats. Res Vet Sci. 130:222–229. doi: 10.1016/j.rvsc.2020.02.012.
- Addie DD, Schaap IAT, Nicolson L, Jarrett O. 2003. Persistence and transmission of natural type I feline coronavirus infection. J Gen Virol. 84(Pt 10):2735–2744. doi: 10.1099/vir.0.19129-0.
- Al-Homidan IH, Ebeid TA, Al-Muzaini A, Abou-Emera OK, Mostafa MM, Fathi MM. 2020. Impact of dietary fenugreek, mung bean, and garden cress on growth performance, carcass traits, blood measurements, and immune response in broiler chickens. Livest Sci. 242:104318. doi: 10.1016/j.livsci.2020.104318.
- Amer A, Suri AS, Rahman OA, Mohd HB, Faruku B, Saeed S, Azmi TIT. 2012. Isolation and molecular characterization of type I and type II feline coronavirus in Malaysia. Virol J. 9(1):278. doi: 10.1186/1743-422X-9-278.
- Amoroso MG, Serra F, Miletti G, Cardillo L, de Martinis C, Marati L, Alfano F, Ferrara G, Pagnini U, De Carlo E, et al. 2022. A retrospective study of viral molecular prevalences in cats in southern Italy (Campania Region). Viruses-Basel. 14(11):2583. doi: 10.3390/v14112583.
- Berenbaum MC. 1989. What is synergy? Pharmacol Rev. 41(2):93–141.
- Cáceres CJ, Cardenas-Garcia S, Carnaccini S, Seibert B, Rajao DS, Wang J, Perez DR. 2021. Efficacy of GC-376 against SARS-CoV-2 virus infection in the K18 hACE2 transgenic mouse model. Sci Rep. 11(1):9609. doi: 10.1038/s41598-021-89013-w.
- Chandel NS. 2021. Nucleotide Metabolism. Cold Spring Harb Perspect Biol. 13(9):a040592. doi: 10.1101/cshperspect.a040576.
- Coggins SJ, Norris JM, Malik R, Govendir M, Hall EJ, Kimble B, Thompson MF. 2023. Outcomes of treatment of cats with feline infectious peritonitis using parenterally administered remdesivir, with or without transition to orally administered GS-441524. J Vet Intern Med. 37(5):1772–1783. doi: 10.1111/jvim.16803.
- Delaplace M, Huet H, Gambino A, Le Poder S. 2021. Feline coronavirus antivirals: a review. Pathogens. 10(9):1150. doi: 10.3390/pathogens10091150.
- Dye C, Siddell SG. 2007. Genomic RNA sequence of feline coronavirus strain FCoV C1Je. J Feline Med Surg. 9(3):202–213. doi: 10.1016/j.jfms.2006.12.002.
- Green J, Syme H, Tayler S. 2023. Thirty-two cats with effusive or non-effusive feline infectious peritonitis treated with a combination of remdesivir and GS-441524. J Vet Intern Med. 37(5):1784–1793. doi: 10.1111/jvim.16804.
- Hohdatsu T, Nakamura M, Ishizuka Y, Yamada H, Koyama H. 1991. A study on the mechanism of antibody-dependent enhancement of feline infectious peritonitis virus infection in feline macrophages by monoclonal antibodies. Arch Virol. 120(3–4):207–217. doi: 10.1007/BF01310476.
- Hohdatsu T, Yamada M, Tominaga R, Makino K, Kida K, Koyama H. 1998. Antibody-dependent enhancement of feline infectious peritonitis virus infection in feline alveolar macrophages and human monocyte cell line U937 by serum of cats experimentally or naturally infected with feline coronavirus. J Vet Med Sci. 60(1):49–55. doi: 10.1292/jvms.60.49.
- Hou DZ, Yousaf L, Xue Y, Hu JR, Wu JH, Hu XS, Feng NH, Shen Q. 2019. Mung bean (Vigna radiata L.): bioactive polyphenols, polysaccharides, peptides, and health benefits. Nutrients. 11(6):1238. doi: 10.3390/nu11061238.
- Hu CJ, Chang WS, Fang ZS, Chen YT, Wang WL, Tsai HH, Chueh LL, Takano T, Hohdatsu T, Chen HW. 2017. Nanoparticulate vacuolar ATPase blocker exhibits potent host-targeted antiviral activity against feline coronavirus. Sci Rep. 7(1):13043. doi: 10.1038/s41598-017-13316-0.
- Ianevski A, Giri AK, Aittokallio T. 2022. SynergyFinder 3.0: an interactive analysis and consensus interpretation of multi-drug synergies across multiple samples. Nucleic Acids Res. 50(W1):W739–W743. doi: 10.1093/nar/gkac382.
- Ianevski A, Giri AK, Gautam P, Kononov A, Potdar S, Saarela J, Wennerberg K, Aittokallio T. 2019. Prediction of drug combination effects with a minimal set of experiments. Nat Mach Intell. 1(12):568–577. doi: 10.1038/s42256-019-0122-4.
- Jaimes JA, Whittaker GR. 2018. Feline coronavirus: insights into viral pathogenesis based on the spike protein structure and function. Virology. 517:108–121. doi: 10.1016/j.virol.2017.12.027.
- Jones S, Novicoff W, Nadeau J, Evans S. 2021. Unlicensed GS-441524-like antiviral therapy can be effective for at-home treatment of feline infectious peritonitis. Animals. 11(8). doi: 10.3390/ani11082257.
- Ke YY, Peng TT, Yeh TK, Huang WZ, Chang SE, Wu SH, Hung HC, Hsu TA, Lee SJ, Song JS, et al. 2020. Artificial intelligence approach fighting COVID-19 with repurposing drugs. Biomed J. 43(4):355–362. doi: 10.1016/j.bj.2020.05.001.
- Kim Y, Liu H, Kankanamalage AC, Weerasekara S, Hua DH, Groutas WC, Chang KO, Pedersen NC. 2016. Correction: reversal of the progression of fatal coronavirus infection in cats by a broad-spectrum coronavirus protease inhibitor. PLOS Pathog. 12(5):e1005650. doi: 10.1371/journal.ppat.1005650.
- Kim Y, Shivanna V, Narayanan S, Prior AM, Weerasekara S, Hua DH, Kankanamalage AC, Groutas WC, Chang KO. 2015. Broad-spectrum inhibitors against 3C-like proteases of feline coronaviruses and feline caliciviruses. J Virol. 89(9):4942–4950. doi: 10.1128/JVI.03688-14.
- Kipar A, Meli ML. 2014. Feline infectious peritonitis still an enigma? Vet Pathol. 51(2):505–526. doi: 10.1177/0300985814522077.
- Klein-Richers U, Hartmann K, Hofmann-Lehmann R, Unterer S, Bergmann M, Rieger A, Leutenegger C, Pantchev N, Balzer J, Felten S. 2020. Prevalence of feline coronavirus shedding in german catteries and associated risk factors. Viruses. 12(9):1000. doi: 10.3390/v12091000.
- Kummrow M, Meli ML, Haessig M, Goenczi E, Poland A, Pedersen NC, Hofmann-Lehmann R, Lutz H. 2005. Feline coronavirus serotypes 1 and 2: seroprevalence and association with disease in Switzerland. Clin Diagn Lab Immunol. 12(10):1209–1215. doi: 10.1128/CDLI.12.10.1209-1215.2005.
- Lee DN, Hung YS, Yang TS, Lin JH, Weng CF. 2017. Aspergillus awamori-fermented mung bean seed coats enhance the antioxidant and immune responses of weaned pigs. J Anim Physiol Anim Nutr. 101(5):e342–e351. doi: 10.1111/jpn.12611.
- Lo CW, Pi CC, Chen YT, Chen HW. 2020. Vigna radiata (L.) R. Wilczek extract inhibits influenza a virus by targeting viral attachment, penetration, assembly, and release. Front Pharmacol. 11:584973. doi: 10.3389/fphar.2020.584973.
- Murota K, Nakamura Y, Uehara M. 2018. Flavonoid metabolism: the interaction of metabolites and gut microbiota. Biosci Biotechnol Biochem. 82(4):600–610. doi: 10.1080/09168451.2018.1444467.
- Murphy BG, Perron M, Murakami E, Bauer K, Park Y, Eckstrand C, Liepnieks M, Pedersen NC. 2018. The nucleoside analog GS-441524 strongly inhibits feline infectious peritonitis (FIP) virus in tissue culture and experimental cat infection studies. Vet Microbiol. 219:226–233. doi: 10.1016/j.vetmic.2018.04.026.
- Pang X, Xu W, Liu Y, Li H, Chen L. 2023. The research progress of SARS-CoV-2 main protease inhibitors from 2020 to 2022. Eur J Med Chem. 257:115491. doi: 10.1016/j.ejmech.2023.115491.
- Pedersen NC, Kim Y, Liu HW, Kankanamalage ACG, Eckstrand C, Groutas WC, Bannasch M, Meadows JM, Chang KO. 2018. Efficacy of a 3C-like protease inhibitor in treating various forms of acquired feline infectious peritonitis. J Feline Med Surg. 20(4):378–392. doi: 10.1177/1098612X17729626.
- Pedersen NC, Perron M, Bannasch M, Montgomery E, Murakami E, Liepnieks M, Liu H. 2019. Efficacy and safety of the nucleoside analog GS-441524 for treatment of cats with naturally occurring feline infectious peritonitis. J Feline Med Surg. 21(4):271–281. doi: 10.1177/1098612X19825701.
- Perera KD, Rathnayake AD, Liu H, Pedersen NC, Groutas WC, Chang KO, Kim Y. 2019. Characterization of amino acid substitutions in feline coronavirus 3C-like protease from a cat with feline infectious peritonitis treated with a protease inhibitor. Vet Microbiol. 237:108398. doi: 10.1016/j.vetmic.2019.108398.
- Rottier PJM, Nakamura K, Schellen P, Volders H, Haijema BJ. 2005. Acquisition of macrophage tropism during the pathogenesis of feline infectious peritonitis is determined by mutations in the feline coronavirus spike protein. J Virol. 79(22):14122–14130. doi: 10.1128/JVI.79.22.14122-14130.2005.
- Takano T, Hohdatsu T, Toda A, Tanabe M, Koyama H. 2007. TNF-alpha, produced by feline infectious peritonitis virus (FIPV)-infected macrophages, upregulates expression of type II FIPV receptor feline aminopeptidase N in feline macrophages. Virology. 364(1):64–72. doi: 10.1016/j.virol.2007.02.006.
- Takano T, Kawakami C, Yamada S, Satoh R, Hohdatsu T. 2008. Antibody-dependent enhancement occurs upon re-infection with the identical serotype virus in feline infectious peritonitis virus infection. J Vet Med Sci. 70(12):1315–1321. doi: 10.1292/jvms.70.1315.
- Tan B, Joyce R, Tan H, Hu Y, Wang J. 2023. SARS-CoV-2 main protease drug design, assay development, and drug resistance studies. Acc Chem Res. 56(2):157–168. doi: 10.1021/acs.accounts.2c00735.
- Triratapiban C, Lueangaramkul V, Phecharat N, Pantanam A, Lekcharoensuk P, Theerawatanasirikul S. 2023. First study on in vitro antiviral and virucidal effects of flavonoids against feline infectious peritonitis virus at the early stage of infection. Vet World. 16(3):618–630. doi: 10.14202/vetworld.2023.618-630.
- Vitiello A. 2022. Sars-Cov-2 and risk of antiviral drug resistance. Ir J Med Sci. 191(5):2367–2368. doi: 10.1007/s11845-021-02820-y.
- Yang CW, Peng TT, Hsu HY, Lee YZ, Wu SH, Lin WH, Ke YY, Hsu TA, Yeh TK, Huang WZ, et al. 2020a. Repurposing old drugs as antiviral agents for coronaviruses. Biomed J. 43(4):368–374. doi: 10.1016/j.bj.2020.05.003.
- Yang TJ, Chang YC, Ko TP, Draczkowski P, Chien YC, Chang YC, Wu KP, Khoo KH, Chang HW, Hsu SD. 2020b. Cryo-EM analysis of a feline coronavirus spike protein reveals a unique structure and camouflaging glycans. Proc Natl Acad Sci USA. 117(3):1438–1446. doi: 10.1073/pnas.1908898117.
- Yen SJ, Chen HW. 2021. Feline coronaviruses identified in feline effusions in suspected cases of feline infectious peritonitis. Microorganisms. 9(9):1801. doi: 10.3390/microorganisms9091801.
- Zhou Q, Li Y, Huang J, Fu NS, Song X, Sha X, Zhang B. 2021. Prevalence and molecular characteristics of feline coronavirus in southwest China from 2017 to 2020. J Gen Virol. 102.
- Zwicklbauer K, Krentz D, Bergmann M, Felten S, Dorsch R, Fischer A, Hofmann-Lehmann R, Meli ML, Spiri AM, Alberer M, et al. 2023. Long-term follow-up of cats in complete remission after treatment of feline infectious peritonitis with oral GS-441524. J Feline Med Surg. 25(8):1098612X231183250. doi: 10.1177/1098612X231183250.