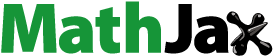
Abstract
Imidacloprid is a widely used pesticide in agriculture. It is being found in aquatic ecosystems in agricultural regions. This study aimed to evaluate its effects on the survival rates, acetylcholinesterase (AChE) and catalase (CAT) responses of larval Eristalis tenax hoverflies. The larvae were exposed for 3, 7 and 14 days to increasing concentrations of imidacloprid (0, 0.1, 0.5 and 2 mg L−1) both indoors at a constant temperature of 20 °C and outdoors under varying environmental conditions. The results revealed that indoors and outdoors, the mortality of E. tenax significantly increased with increasing imidacloprid concentration and duration of exposure. Median lethal concentrations (LC50) varied from 0.03 to 0.17 mg L−1 depending on the duration and conditions of exposure. Indoors, AChE activity decreased in all the treatments for all three exposure durations, whereas outdoors the decrease was observed after the short (3-day) and long (14-day) exposure durations. AChE inhibition ranged from 6% to 62% (indoors) and 12% to 62% (outdoors). Variations in CAT activity were observed for both experimental setups, with a decrease outdoors in larvae exposed to 0.5 mg L−1 for 7 days and a gradual dose-dependent increase indoors for exposure lasting 3 and 7 days. This study sheds light on the potential ecological implications of imidacloprid contamination which may cause the decline of aquatic insect populations and pollination rates, leading to disruptions of the food chain and the overall decline of aquatic and terrestrial ecosystem health.
Introduction
The pesticide industry has developed various agrochemicals useful for effective integrated pest management. Besides their usefulness in warding off pests, the undesired effects of these chemicals on non-target organisms have been pointed out.[Citation1,Citation2] One group of pesticides in particular, the neonicotinoids, is found in some of the most widely used agrochemicals worldwide. Imidacloprid alone is used on over 140 crops for the control of invertebrate pests, and in veterinary medicine as a topical ectoparasiticide.[Citation3,Citation4] It is registered in more than 120 countries.[Citation5] In South Africa where over 130 imidacloprid-containing insecticides are registered, their geographical distribution is associated with grain pretreatment and application rates range from 0.07 to 2.32 Kg ha−1.[Citation6]
Imidacloprid is a systemic insecticide synthesized for the first time in 1985.[Citation7] It is still widely used in developing countries such as South Africa where it was introduced in the 1990s.[Citation6] Like other insecticides of the neonicotinoid group, imidacloprid binds to postsynaptic nicotinic acetylcholine receptors (nAChRs) in insects and mimics the action of acetylcholine.[Citation3] The continuous activation of acetylcholine receptors eventually causes over stimulation of the central nervous system, inducing uncontrolled muscular contraction, eventually leading to paralysis and death.[Citation3]
Imidacloprid is a highly leaching compound with different dissipation times depending on soil type.[Citation5] As a consequence of runoff, it is frequently detected in surface waters near agricultural areas.[Citation8–10] Research suggests that the average concentration of imidacloprid in aquatic systems has increased during the past 15 years, indicating an increasing trend in the worldwide use of this insecticide.[Citation5,Citation11] In some aquatic ecosystems in agricultural regions, imidacloprid concentrations have been found to reach up to 0.32 mg L−1.[Citation5,Citation12] As such, this insecticide is a threat to aquatic insect populations[Citation12] and deleterious effects on non-target organisms have been reported.[Citation2,Citation9,Citation13,Citation14]
Among non-target organisms, there are beneficial insects that need aquatic media to complete their life cycle and which are regularly subject to pesticide exposure through surface waters.[Citation5,Citation12] For instance, the exposure of the hoverfly species Eristalis tenax to pesticides during its life cycle could hamper the emergence of adults because pre-emergence development involves two stages: the aquatic larva (which lasts between 13 to 24 days) and the aerial pupa.[Citation15,Citation16] Larvae face the dual challenges of avoiding predators[Citation17] and coping with the environmental stressors found in their growing media. Even though different neonicotinoids vary in toxicity,[Citation1] the literature shows a consistent tendency for a high acute-chronic ratio indicating considerably lower chronic effect concentrations than those required to produce acute toxicity.[Citation18] In a review focusing on aquatic invertebrates, Morissey et al.[Citation5] compiled data on median lethal concentrations (LC50) and half maximal effective concentrations (EC50) for 214 acute (24–96 h) and 36 chronic studies (7–28 days) and concluded that species vary in their sensitivity to neonicotinoids with sublethal endpoints in chronic tests usually more sensitive than sublethal endpoints in acute tests. For aquatic tests using Diptera, the mayfly Chironomus dilutes (Chironomidae) is the species most tested with an LC50 value of 0.0329 mg L−1 for imidacloprid acute tests, specifically. From Morissey et al.[Citation5] it can be seen that the majority of studies on neonicotinoids have focused on acute exposure effects (≤ 96h, 83%) rather than chronic exposure effects (17%). A recent study on the impact of the neonicotinoid thiamethoxam on the aquatic larvae of E. tenax showed decreased survival rates, but no associated effects on larval development and adult activity budgets were observed.[Citation16] Another study on the effects of imidacloprid on E. tenax revealed that sub-lethal exposure alters its physiological response to motion stimuli affecting flight control, hovering and routing.[Citation19] Easton and Goulson.[Citation2] also reported that given the choice, in the field, hoverflies such E. tenax and Episyrphis balteatus choose to avoid pan traps contaminated with imidacloprid. However, very often these exposure studies of E. tenax do not link imidacloprid exposure to biological effects. Consequently, the ecological implications of imidacloprid exposure in this species are not well known. Moreover, the sub-organismal effects of imidacloprid have not been examined in any species of this economically important group of insects, making this contribution a rare comparative study of biomarker responses in the hoverfly E. tenax under constant and varying environmental conditions.
A biomarker refers to any biological response following exposure to a pollutant that can be measured at the sub-individual level and cannot be detected in an intact organism, thus indicating a deviation from normal status.[Citation20] Pesticides and other xenobiotics may increase the formation of free radicals in cells[Citation21] and activate detoxification mechanisms such as antioxidant defence systems present in tissues and cells.[Citation22] This makes it relevant to assess adverse effects at life-cycle level as well as modifications of cellular and molecular defence systems that can both be used as biomarkers.[Citation23] Different biomarkers are routinely used to assess exposure to xenobiotics in aquatic organisms.[Citation24] Among them are two important biomarkers viz. catalase (CAT), which acts as reactive oxygen species (ROS) scavenger,[Citation25] and acetylcholinesterase (AChE), which hydrolyzes the neurotransmitter acetylcholine in cholinergic synapses of the nervous system.[Citation26]
The main aims of the present study were to examine the lethal and sublethal effects of imidacloprid on E. tenax and to assess the levels of sub-organismal stress using AChE and CAT biomarkers.
Materials and methods
Chemical
The neonicotinoid insecticide used in this study was a commercial grade formulation of imidacloprid [1-(6-chloro-3-pyridylmethyl)-N-nitroimidazolidin-2-ylideneamine] called Aphicide Plus® and containing 20 g L−1. Aphicide Plus® is produced by Efekto South Africa and was purchased in a local chain store.
Experimental approach
The hoverfly species E. tenax was the selected test organism. Larvae of E. tenax were collected in an artificially created oviposition medium from a local garden in the town of Harrismith, (South Africa, 28°27’73′’S 29°13’88′’E) and transported to the ecotoxicology research laboratory housed in the Department of Zoology and Entomology of the University of the Free State-QwaQwa Campus. The larvae were left to acclimatize for 24 h before the beginning of the experiments. Five days old larvae of body length lower than 6 mm were used for the experiments. Following the protocol of Basley et al.[Citation16] with few modifications, the exposure substrate was produced by filling two 10 L buckets with a mixture of fresh grass and tap water. The buckets were covered with a fine-proof muslin and left outside to allow grass decomposition for two weeks. The grass was then strained through the muslin to retain the ‘‘rotting plant water’’ which was used as exposure substrate. An additional 5 L bucket was filled with a mix of sawdust and tap water, after two weeks the “sawdust solids” were retained, and the water was discarded.
The final experimental substrate was made by mixing 700 mL of the rotting plant water with 300 mL of distilled water. This solution was then contaminated with imidacloprid to make a stock solution of 2 mg L−1. Solutions of 0.5 and 0.1 mg L−1 were subsequently made. 100 mL of each treatment and 3 g sawdust solids were mixed in 0.2 L plastic cups. The actual imidacloprid concentrations in the medium were analyzed by LC-MSMS + GC-MSMS (liquid chromatography-mass spectrometry and gas chromatography-mass spectrometry) at the limit of quantification of 0.01 mg kg−1. Total nominal (and actual) imidacloprid concentrations measured at the start of the toxicity experiment were 0 (0), 0.1 (0.15), 0.5 (0.4) and 2 (1.9) mg L−1 solution. Surface water contamination by imidacloprid has been found to range from 0.001 to 0.32 mg L−1.[Citation5,Citation12]
Groups of five larvae were thereafter randomly exposed to the following imidacloprid concentrations: 0 (negative control), 0.1, 0.5 and 2 mg L−1. The exposures were conducted in eight replicates (i.e. 40 larvae per concentration). Each plastic container was placed in a perforated tie-top plastic bag. This experiment was conducted both indoors at constant temperature and outdoors under variable environmental conditions.
The indoor experiment was run in a climate chamber at 20 °C ± 2 under a 12/12 light cycle. For the outdoor experiment, the exposure containers were placed in a greenhouse in closed trays only allowing for gaseous, filtered light (through the greenhouse roof) and heat exchanges. Daily temperatures were recorded for the entire duration of the outdoor experiment (). For each experiment (either indoors or outdoors), out of the eight replicates, three were used for biomarkers analysis.
During the experiment, the exposure treatments were monitored daily. Dead larvae were immediately removed from the containers. After 14 days, all exposures were terminated, although mortality and stress biomarkers (acetylcholine and catalase activities) were measured at day 3, 7 and 14. A recent study by Kamdem and Voua Otomo[Citation15] has reported E. tenax larval development time ranging from 13 to 24 days. The organisms used for biomarker assays were individually removed from the exposure containers and placed in 50 mL Eppendorf tubes. The Eppendorf tubes were stored at −80 °C until biomarker analyses could be conducted.
Biomarkers tissue sample preparation and protein determination
The sublethal effects of imidacloprid at suborganismal level were determined using one biomarker of oxidative stress (catalase content)[Citation27] and acetylcholinesterase inhibition as a neurotoxicity biomarker.[Citation28] The entire larval body was homogenized in 2 volumes (w/v) of a cold (4 °C) 0.01 M phosphate buffer (pH 7.4) for 30 s.[Citation29] The homogenates were then pooled from three individuals per treatment using an homogenizer and centrifuged at 10000 g (4 °C) for 10 min, the supernatant was poured into new Eppendorf tubes and kept at −20 °C.[Citation29] Total soluble protein content of the homogenized samples was determined using BCA protein assay kit (Thermo ScientificTM).
Acetylcholinesterase inhibition
Acetylcholinesterase activity was measured according to the method of Ellman et al.[Citation30] The assay mixture contained the larval sample extract (5 µl), in 210 µl (w/v) of 0.09 M phosphate buffer (pH 7.4), 10 µl of 30 mM acetylthiocholine iodide and 10 µl of 10 mM of 5,5′-dithio-bis-(2-ni-trobenzoicacid) (DTNB). Tris buffer (0.09 M, pH 7.4) was used as a blank. The reaction was allowed to proceed for 5 min at 37 °C, and the absorbance of the reaction mixture was read at wavelength of 412 nm for 6 min at 1-min intervals (Beckmann DU 7400, USA). Acetylcholinesterase activity was expressed as nmol mg−1 protein min−1.
Catalase activity
Catalase activity was determined according to the method of Cohen et al.[Citation29] It consisted in measuring the ability of hydrogen peroxide (H2O2) to react with a standard excess of potassium permanganate (KMnO4). The reaction mixture contained the larval sample extract (10 µl), in 10 µl (w/v) 0.01 M phosphate buffer (pH 7.0) and 93 µl of 6 mM (30%) hydrogen peroxide (H2O2), 19 µl of 6 N sulfuric acid (H2SO4) and 130 µl of 0.01 N potassium permanganate (KMnO4). The entire procedure was carried out at 4 °C. The decomposition of hydrogen peroxide by the catalase present in the sample was determined at a wavelength of 490 nm within 30–60 s (Beckmann DU 7400, USA), and the results expressed in U mg−1 protein min−1 using the following equation:
where k is the first-order reaction rate constant, S0 is the mean of standard absorbance readings, S3 was previously measured as standard minus average absorbance of the samples, and t is the time taken to measure the reaction.
Statistical analysis
During the experiments (indoors and outdoors), the mortality in treated populations was corrected by using the formula of Abbott[Citation31] for control mortality: corrected mortality (%) = (% alive control – % alive treated) × 100/(% alive control). All data were tested for normality using the Kolmogorov–Smirnov test and equality of variances was tested according to Bartlett’s test (at 95% confidence intervals). The statistical difference of the larval mortality and activity of antioxidant enzymes of the test organism exposed to pesticide stress with respect to the control were determined by the use of one-way analysis of variance (ANOVA). Tukey’s post-hoc test was carried out to compare each treatment with the respective control. All statistical analyses were performed using GraphPad Prism version 5.00 (GraphPad Software, San Diego, CA, USA, www.graphpad.com). The LC50s for imidacloprid were calculated for each experiment and time interval using the freeware AAT Bioquest (https://www.aatbio.com/tools/lc50-calculator).
Results
Mortality rates of E. tenax larvae at different concentrations of imidacloprid after exposure for 3, 7 and 14 days are presented in . Regardless of the temperature regime (either constant or variable), hoverfly mortality increased when the concentration and the time of exposure increased.
Figure 2. Cumulative mortality of Eristalis tenax after exposure to imidacloprid after 3, 7 and 14 days, (a) indoors and (b) outdoors. The data are expressed as mean ± SE. Mortality data in the treatments were converted into percentage mortality based on mortality data from the respective controls. Different letters above the bars indicate significant differences between the treatments at p ˂ 0.05.
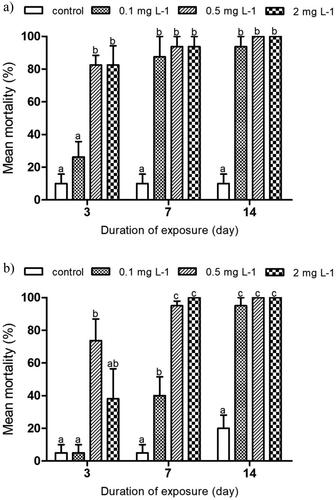
Indoors, after 3 days of exposure, larval mortality was significantly higher in the 0.5 and 2 mg L−1 imidacloprid treatments compared to the control (p < 0.05, ). After 7 and 14 days, all imidacloprid treatments caused significantly more mortality than the control (p < 0.05). These results translated into LC50 values of 0.12, 0.05 and 0.03 mg L−1 at day 3, 7 and 14, respectively. In the outdoor experiment, the 0.5 mg L−1 treatment was the only one to show a significantly higher larval mortality rate when compared to the control after 3 days of exposure (p < 0.05, ). After 7 and 14 days of exposure, larval mortality rates significantly increased in all imidacloprid treatments when compared to the respective controls (p < 0.05). The LC50 indices were 0.17, 0.11 and 0.04 mg L−1 at day 3, 7 and 14, respectively.
shows the effect of imidacloprid on AChE activity in E. tenax after exposure for 3, 7 and 14 days indoors (a) and outdoors (b). The 2 mg L−1 imidacloprid treatment was not represented on the graphs because mortality rates in this treatment did not allow reliable biomarker assessment. The indoor experiment showed a statistically significant reduction in AChE activity in all treatments compared to the control (p ˂ 0.05; ) for all exposure periods. Interestingly, about 30% and 35% inhibition in AChE activity (15.95 ± 0.22 and 14.76 ± 0.11 nmol mg−1 protein min−1) was recorded after 3 days of E. tenax exposure to 0.1 and 0.5 mg L−1, respectively compared to the control (22.97 ± 0.22 nmol mg−1 protein min−1). This inhibition was reduced to 6% and 22% (32.18 ± 0.26 and 26.58 ± 0.21 versus 34.16 ± 0.36 nmol mg−1 protein min−1 [control]) on day 7, and then resurged to reach 39% and 62% (31.28 ± 0.21 and 19.61 ± 0.14 versus 51.82 ± 0.82 nmol mg−1 protein min−1 [control]) after 14 days of exposure to 0.1 and 0.5 mg L−1, respectively. In the outdoor experiment, AChE activity was significantly reduced when compared to the respective control at day 3 and day 14 of the experiment (p ˂ 0.05; ). AChE activity for contaminated hoverfly larvae was about 28% and 48% (40.03 ± 3.21 and 29.25 ± 2.12 nmol mg−1 protein min−1) in 0.1 and 0.5 mg L−1, respectively after 3 days of exposure when compared to control (56.14 ± 3.99 nmol mg−1 protein min−1). The activity was then slightly reduced to 15% and 12% (48 ± 2.30 and 45.57 ± 2.74 nmol mg−1 protein min−1) after 7 days of exposure to 0.1 and 0.5 mg L−1, respectively – with no significant difference from the respective control (52.15 ± 4.43 nmol mg−1 protein min−1). This inhibition then exponentially progressed to 62% and 60% (3.04 ± 0.39 and 3.26 ± 0.12 versus 8.13 ± 0.16 nmol mg−1 protein min−1) at day 14 in the 0.1 and 0.5 mg L−1 treatment, respectively. After the 14-day exposure, the lowest AChE activity of 3.04 nmol mg−1 protein min−1 was found in the 0.5 mg L−1 treatment exposed outdoors. Similarly, indoors, the lowest AChE activity of 14.76 nmol mg−1 protein min−1 was observed in the larvae exposed to the same 0.5 mg L−1 treatment.
Figure 3. Activity of acetylcholinesterase (AChE) in the hoverfly larvae of Eristalis tenax exposed to imidacloprid contaminated substrate for 3, 7 and 14 days, a) indoors and b) outdoors. Data is expressed as mean ± SE. Different letters indicate significant differences between the treatments at p ˂ 0.05.
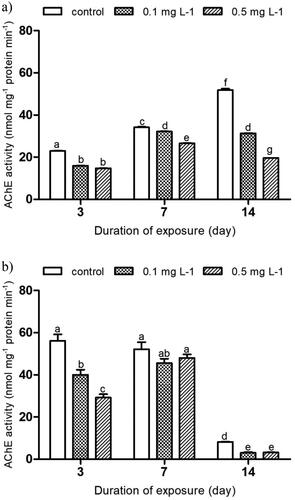
CAT activity varied depending on imidacloprid concentrations and exposure duration. In the indoor experiment, CAT activity in E. tenax larvae exposed to 0.5 mg L−1 increased significantly to 53% of the control after 3 days of exposure (24.16 ± 2.85 versus 15.79 ± 3.36 U mg−1 protein min−1 [control], p ˂ 0.05, ). After 7 days of exposure, CAT activity in larvae exposed to 0.1 and 0.5 mg L−1 continued to exponentially increase to 124% and 115% of the control (33.88 ± 0.80 and 32.45 ± 2.78 versus 15.09 ± 3.57 U mg−1 protein min−1 [control], p ˂ 0.05). Inversely, after 14 days of exposure, CAT activity in larvae exposed to 0.5 mg L−1 significantly dropped by 26% of the control (26.60 ± 3.59 versus 35.96 ± 3.75 U mg−1 protein min−1 [control], p ˂ 0.05). In the outdoor experiment, a significant decrease in CAT activity was only observed in E. tenax larvae exposed to 0.5 mg L−1 compared to control at day 7 of the exposure (13.87 ± 0.56 versus 24.02 ± 8.67 U mg−1 protein min−1 [control], p ˂ 0.05, ). At day 14, a significant increase in CAT activity was only recorded in the 0.1 mg L−1 treatment when compared to the control (19.32 ± 2.67 versus 5.41 ± 1.27 U mg−1 protein min−1 [control], p ˂ 0.05, ).
Discussion
The present study shows that the neonicotinoid imidacloprid has a considerable lethal effect on non-target organism such as E. tenax. summarizes published studies on the chronic toxicity of imidacloprid to aquatic Diptera. To the best of our knowledge no prior study has assessed the toxicity of imidacloprid to E. tenax. Our study represents the first attempt to investigate the effect of imidacloprid on the survival and biomarker responses of this species. From previous studies and the present one, even at low doses, imidacloprid is toxic to the midge and hoverfly (see ). In the present study, it is noticeable that the longer the exposure period the greater the toxicity of imidacloprid to E. tenax, regardless of the environmental conditions (indoors vs. outdoors). The explanation could be that the individuals that face continuous stress are increasingly subjected to over stimulation of the central nervous system which eventually leads to death. By comparing the LC50 values for each exposure duration, it appears that outdoor, imidacloprid was less potent during the first seven days compared to indoor. This means that in controlled environment, imidacloprid might be more toxic than in nature where biotransformation reactions involving detoxifying mechanisms (e.g., Tomizawa and Casida[Citation3]) could be optimal to enforce insect tolerance for a certain time. Additionally, outdoor, imidacloprid is exposed to environmental factors such as sunlight and other elements that might cause it to degrade quicker, rendering it less potent.[Citation2]
Table 1. Published chronic imidacloprid median lethal (LC50) and effective (EC50) concentrations (mg L−1) for the Diptera species tested in the present and previous studies.
Basley et al.[Citation16] is the only other study that has tested the toxicity of a neonicotinoid (thiamethoxam) on the larvae of E. tenax. They observed the lowest survival rates in larvae treated with 1 mg L−1 thiamethoxam and generated a 9 to 13-day LC50 of 0.215 mg L−1 compared to the present study where the 14-day LC50 of imidacloprid was 0.03 mg L−1. The current results suggest that imidacloprid could be more toxic than thiamethoxam. Some authors have argued that there are no real differences in toxicity among neonicotinoid insecticides, and that guidelines for other neonicotinoids should be harmonized to that of imidacloprid.[Citation38] Contrary to the conclusions by Mineau and Palmer,[Citation38] Raby et al.[Citation32] assessing the chronic toxicities of six neonicotinoids to the midge Chironomus dilutes, generated different values for a 14-day LC50 for acetamiprid (0.0011 mg L−1), clothianidin (> 0.0025 mg L−1), dinotefuran (0.0150 mg L−1) and thiacloprid (0.00073 mg L−1). With regard to imidacloprid, they generated a 14-day LC50 of 0.0002 mg L−1. Their LC50 for thiamethoxam was higher than that of imidacloprid (0.0458 mg L−1), showing that imidacloprid may be more toxic to Diptera than thiamethoxam but also that different neonicotinoids certainly exert different degrees of toxicity on this organism.
In the field of aquatic toxicology, AChE a biomarker reflecting susceptibility, is widely used in biomonitoring studies as a biomarker of exposure, especially when neonicotinoid insecticides are involved.[Citation24,Citation39] The stress induced by neonicotinoid insecticides is usually expressed in the form of biochemical imbalances. In the present study, the assessment of AChE activity in tissues of E. tenax after treatment with imidacloprid revealed an inhibition of AChE irrespective of the exposure set up (indoors vs. outdoors). This significant decrease in AChE activity is a result of the interaction of the insecticide with nAChR sites,[Citation3,Citation40] showing the evidence for imidacloprid being the causative agent behind this inhibition of AChE activity. We observed a general positive correlation between the imidacloprid concentrations, and the duration of exposure (3, 7 and 14 days) associated with the degree of AChE activity indoor (). In general, inhibition of AChE activity was higher after 14 days of exposure irrespective of the experimental setup, which was supported by almost 100% mortality in imidacloprid treatments. Connell et al.[Citation41] reported that in fish species, mortality may occur in individuals for whom 80–90% of AChE activity is inhibited, and toxic effects may be observed when 50% of AChE is inhibited. In the case of E. tenax larvae in the present study, mortality already occurred when values for AChE inhibition were between 6%-22% (indoors) and 12%-15% (outdoors). In the outdoor experiment, AChE activity was higher than in the indoor experiment (). In the outdoor experiment, fluctuations in AChE activity were different from the ones indoors and were characterized by relatively high AChE activity at day 3 and day 7 and statistically low activity at day 14. This perhaps showed the influence of the outdoor environment. Filtered light, gaseous and heat exchanges were higher during this experiment. Together with the daily temperature amplitude (15–25 °C), they were the reason behind these fluctuations. This water-soluble insecticide seems to degrade faster in warm and sunlit environments,[Citation2] which may produce less potent metabolites.[Citation3] It has been reported that the nitrosoguanidine metabolite of imidacloprid has moderate to high insect nAChR potency, whereas the guanidine metabolite is deactivated against insect nAChRs.[Citation42] In addition, there was a drastic decrease in AChE activity at day 14. This reduction at day 14 contrasts with observations in the fish Tilapia mossambica by Kabeer et al.[Citation43] who found that after a high accumulation of acetylcholine in the tissues resulting from AChE inhibition due to malathion (an organophosphate), the inhibitory effect produced by the pesticide was reduced with increasing duration of exposure, which led to increased levels of AChE activity. Similar findings were reported by Khalil[Citation24] who observed high levels of AChE activity in freshwater snails Lanistes carinatus after 28 days of exposure to chlorpyrifos.
In the outdoor experiment, a significant increase in CAT activity was observed in E. tenax exposed to 0.1 mg L−1 of imidacloprid on the 14th day (). In the indoor experiments, a significant increase in CAT enzyme was also observed in E. tenax exposed to 0.1 (at day 7) and 0.5 (at day 3 and 7) (). A possible explanation would be that the initial increase of oxygen free radicals stimulates CAT activity to combat the high pollutant load experienced by E. tenax during these specific periods, which may be associated with significant inhibition of AChE. These results are also supported by Khalil[Citation24] who studied the effects of chlorpyrifos on levels of CAT in snail tissues. This author found that during the initial exposure period of 7 days, CAT activity in snails exposed to chlorpyrifos increased significantly compared to the control. Contrariwise, the significant decrease CAT activity in the outdoor experiment in E. tenax exposed to 0.5 mg L−1 of imidacloprid on the 7th day (outdoors) and 14th day (indoors) could be explained by the reduction in energy budget of the organism over time. Some authors argued that an organism exposed to sublethal concentrations of chemical stressors should experience a reduction of its physiological energy budget over time.[Citation44,Citation45] Rodrigues et al.[Citation27] found decreased CAT levels in larvae of Chironomus riparius (Diptera) exposed to 0.0096 mg L−1 of the insecticide chlorantraniliprole for 24h.
Since CAT activity represents the first line of defence (along with super-oxide dismutase) when it comes to oxidative stress,[Citation20,Citation22] the lower levels of CAT activity in E. tenax exposed outdoors could be explained by decreased toxic effects of degradation products of imidacloprid under environmental conditions. Our results show that within the concentration range studied, the protective mechanisms of CAT in E. tenax were not able to efficiently guard it against increasing levels of oxidative stress and prevent the high mortality rates observed in . Nevertheless, in the present study, the experiments confined to the laboratory were useful to single out the toxicity of imidacloprid to E. tenax, whereas exposures under varying environmental conditions made it possible to identify parameters that may come into play in the field. The differences in biomarker responses observed between indoor and outdoor experiments point to the complexity of extrapolating laboratory findings to the environment.
The results of this study shed some light on the ecological implications of the exposure of pollinating insects such as E. tenax to imidacloprid. The high mortalities observed in E. tenax larvae exposed to imidacloprid suggest a potential risk to the adult population of this species. As a pollinator, E. tenax contributes to plant pollination, and a decrease in its population can disrupt plant pollination, affecting fruit and seed reproduction. In South Africa, E. tenax which is found everywhere[Citation46] shares a habitat with many other pollinators and has been shown to positively impact the pollination of several plant groups.[Citation47] Eristalis tenax larvae are a food source for many aquatic and terrestrial predators.[Citation17] Decreasing larval populations due to imidacloprid contamination can directly affect the species that depend on them as a food source, thus disrupting regional food chains. As a systemic insecticide, imidacloprid presents a major risk to aquatic and terrestrial ecosystems, as it can spread from the areas of application to neighboring ecosystems, threatening the overall health of these ecosystems.[Citation12] Regulatory interventions are, therefore, required to ensure the sustainable preservation of important aquatic saprophagous species like E. tenax.
Conclusion
In this study, clear toxic effects of imidacloprid on E. tenax larvae were detected irrespective of the experimental setup. The results of the biomarker responses showed that exposure of E. tenax to imidacloprid inhibited AChE much more indoors than outdoor. The pattern of the protective mechanism of CAT in E. tenax was almost the same in the indoor and outdoor experiments, even if CAT activity was higher in the indoor experiments. This study shows the importance of conducting both laboratory experiments and outdoor experiments under environmentally realistic conditions. Even though field experiments and replicated evidence are needed to confirm the extent of the adverse effects of imidacloprid on E. tenax larvae, the findings of this study highlight the dangers associated with the overuse of this pesticide in countries such as South Africa, where it is still freely sold over the counter. Further studies should investigate the sublethal effects of imidacloprid on the development and behavior of E. tenax larvae, as well as the synergistic effects of imidacloprid and other pesticides on the aquatic larval stages and adult behavior of E. tenax.
Data availability statement
The datasets generated during the current study are available from the corresponding author on reasonable request.
Disclosure statement
The authors declare no competing interests.
Additional information
Funding
References
- Pisa, L. W.; Amaral-Rogers, V.; Belzunces, L. P.; Bonmatin, J. M.; Downs, C.; Goulson, D.; Kreutzweiser, D. P.; Krupke, C.; Liess, M.; Mcfield, M.; et al. Effects of Neonicotinoids and Fipronil on Non-Target Invertebrates. Environ. Sci. Pollut. Res. Int. 2015, 22, 68–102. DOI: 10.1007/s11356-014-3471-x.
- Easton, A. H.; Goulson, D. The Neonicotinoid Insecticide Imidacloprid Repels Pollinating Flies and Beetles at Field-Realistic Concentrations. PLoS ONE. 2013, 8, e54819. DOI: 10.1371/journal.pone.0054819.
- Tomizawa, M.; Casida, J. E. Neonicotinoid Insecticide Toxicology: Mechanisms of Selective Action. Annu. Rev. Pharmacol. Toxicol. 2005, 45, 247–268. DOI: 10.1146/annurev.pharmtox.45.120403.095930.
- Botha, C. J.; Du Plessis, E. C.; Coetser, H.; Rosemann, M. Analytical Confirmation of Imidacloprid Poisoning in Granivorous Cape Spurfowl (Pternistis capensis). J. S Afr. Vet. Assoc. 2018, 89, e1–e5. DOI: 10.4102/jsava.v89i0.1637.
- Morrissey, C. A.; Mineau, P.; Devries, J. H.; Sanchez-Bayo, F.; Liess, M.; Cavallaro, M. C.; Liber, K. Neonicotinoid Contamination of Global Surface Waters and Associated Risk to Aquatic Invertebrates: A Review. Environ. Int. 2015, 74, 291–303. DOI: 10.1016/j.envint.2014.10.024.
- Dabrowski, J. M. Investigation of the Contamination of Water Resources by Agricultural Chemicals and the Impact on Environmental Health. Volume 2: Prioritising Human Health Effects and Mapping Sources of Agricultural Pesticides Used in South Africa. WRC Report No. TT 642/15. Pretoria, South Africa: Water Research Commission, 2015.
- Elbert, A.; Nauen, R.; Leicht, W. Imidacloprid, a Novel Chloronicotinyl Insecticide: Biological Activity and Agricultural Importance. In Insecticides with Novel Modes of Action: Mechanism and Application; Ishaaya, I.; Degheele, D., Eds.; Berlin Heidelberg: Springer Verlag, 1998, 50–74
- Samson-Robert, O.; Labrie, G.; Chagnon, M.; Fournier, V. Neonicotinoid-Contaminated Puddles of Water Represent a Risk of Intoxication for Honey Bees. PLoS ONE. 2014, 9, e108443. DOI: 10.1371/journal.pone.0108443.
- Anderson, J. C.; Dubetz, C.; Palace, V. P. Neonicotinoids in the Canadian Aquatic Environment: A Literature Review on Current Use Products with a Focus on Fate, Exposure, and Biological Effects. Sci. Total Environ. 2015, 505, 409–422. DOI: 10.1016/j.scitotenv.2014.09.090.
- Schaafsma, A.; Limay-Rios, V.; Baute, T.; Smith, J.; Xue, Y. Neonicotinoid Insecticide Residues in Surface Water and Soil Associated with Commercial Maize (Corn) Fields in Southwestern Ontario. PLoS ONE. 2015, 10, e0118139. DOI: 10.1371/journal.pone.0118139.
- CCME. Canadian Water Quality Guidelines: Imidacloprid. scientific Supporting Document. Winnipeg: Canadian Council of Ministers of the Environment, 2007.
- Van Dijk, T. C.; Van Staalduinen, M. A.; Van der Sluijs, J. P. Macro-Invertebrate Decline in Surface Water Polluted with Imidacloprid. PLoS ONE. 2013, 8, e62374. DOI: 10.1371/journal.pone.0062374.
- Henry, M.; Béguin, M.; Requier, F.; Rollin, O.; Odoux, J.-F.; Aupinel, P.; Aptel, J.; Tchamitchian, S.; Decourtye, A. A Common Pesticide Decreases Foraging Success and Survival in Honey Bees. Science 2012, 336, 348–350. DOI: 10.1126/science.1215039.
- Macaulay, S. J.; Buchwalter, D. B.; Matthaei, C. D. Water Temperature Interacts with the Insecticide Imidacloprid to Alter Acute Lethal and Sublethal Toxicity to Mayfly Larvae. New Zeal. J. Mar. Fresh. Res. 2020, 54, 115–130.
- Kamdem, M. M.; Voua Otomo, P. Developmental Performance of Eristalis tenax Larvae (Diptera: Syrphidae): Influence of Growth Media and Yeast Addition during Captive Rearing. JEZ-A: Ecol. Integrative Physiol. 2023, 339, 1–11.
- Basley, K.; Davenport, B.; Vogiatzis, K.; Goulson, D. Effects of Chronic Exposure to Thiamethoxam on Larvae of the Hoverfly Eristalis tenax (Diptera, Syrphidae). PeerJ 2018, 6, e4258. DOI: 10.7717/peerj.4258.
- Rotheray, G. E.; Gilbert, F. S. The Natural History of Hoverflies. Forrest Text, Tresaith, Wales, 2011.
- Sánchez-Bayo, F.; Goka, K.; Hayasaka, D. Contamination of the Aquatic Environment with Neonicotinoids and Its Implication for Ecosystems. Front. Environ. Sci. 2016, 4, 1–14. DOI: 10.3389/fenvs.2016.00071.
- Rigosi, E.; O’Carroll, D. C. The Cholinergic Pesticide Imidacloprid Impairs Contrast and Direction Sensitivity in Motion Detecting Neurons of an Insect Pollinator. bioRxiv 2018, 295576.
- van der Oost, R.; Beyer, J.; Vermeulen, N. P. E. Fish Bioaccumulation and Biomarkers in Environmental Risk Assessment: A Review. Environ. Toxicol. Pharmacol. 2003, 13, 57–149. DOI: 10.1016/s1382-6689(02)00126-6.
- Freeman, B.; Crapo, J. Biology of Disease. Free Radicals and Tissue Injury. Lab. Invest. 1982, 47, 412–426.
- Sithole, S.; Nyoka, N. N.-K.; Kamdem, M. M.; Voua Otomo, P. Dietary Deprivation Reduces the Deleterious Effects of Carbaryl on the Survival and Activity of Both Catalase and Acetylcholinesterase in Earthworms. Ecotoxicol. Environ. Saf. 2023, 263, 115293. DOI: 10.1016/j.ecoenv.2023.115293.
- Downs, C. A.; Dillon, J. R. T.; Fauth, J. E.; Woodley, C. M. A Molecular Biomarker System for Assessing the Health of Gastropods (Ilyanassa obsoleta) Exposed to Natural and Anthropogenic Stressors. J. Exp. Mar. Biol. Ecol. 2001, 259, 189–214. DOI: 10.1016/s0022-0981(01)00233-7.
- Khalil, A. M. Toxicological Effects and Oxidative Stress Responses in Freshwater Snail, Lanistes carinatus, following Exposure to Chlorpyrifos. Ecotoxicol. Environ. Saf. 2015, 116, 137–142. DOI: 10.1016/j.ecoenv.2015.03.010.
- Felton, G. W.; Summers, C. B. Antioxidant Systems in Insects. Arch. Insect Biochem. Physiol. 1995, 29, 187–197. DOI: 10.1002/arch.940290208.
- Smallman, B. N.; Mansingh, A. The Cholinergic System in Insect Development. Annu. Rev. Entomol. 1969, 14, 387–408. DOI: 10.1146/annurev.en.14.010169.002131.
- Rodrigues, A. C. M.; Gravato, C.; Quintaneiro, C.; Golovko, O.; Žlábek, V.; Barata, C.; Soares, A. M. V. M.; Pestana, J. L. T. Life History and Biochemical Effects of Chlorantraniliprole on Chironomus riparius. Sci. Total Environ. 2015, 508, 506–513. DOI: 10.1016/j.scitotenv.2014.12.021.
- Tennekes, H. A.; Sánchez-Bayo, F. Time-Dependent Toxicity of Neonicotinoids and Other Toxicants: Implications for a New Approach to Risk Assessment. J. Environ. Anal. Toxicol 2011, S4, 1.
- Cohen, G.; Dembiec, D.; Marcus, J. Measurement of Catalase Activity in Tissue Extracts. Anal. Biochem. 1970, 34, 30–38. DOI: 10.1016/0003-2697(70)90083-7.
- Ellman, G. L.; Courtney, K. D.; Andres, V.; Feather-Stone, R. M. A New and Rapid Colorimetric Determination of Acetylcholinesterase Activity. Biochem. Pharmacol. 1961, 7, 88–95. DOI: 10.1016/0006-2952(61)90145-9.
- Abbott, W. S. A Method of Computing the Effectiveness of an Insecticide. 1925. J. Am. Mosq. Control Assoc. 1987, 3, 302–303.
- Raby, M.; Zhao, X.; Hao, C.; Poirier, D. G.; Sibley, P. K. Chronic Toxicity of 6 Neonicotinoid Insecticides to Chironomus dilutus and Neocloeon triangulifer. Environ. Toxicol. Chem. 2018, 37, 2727–2739. DOI: 10.1002/etc.4234.
- Maloney, E. M.; Morrissey, C. A.; Headley, J. V.; Peru, K. M.; Liber, K. Can Chronic Exposure to Imidacloprid, Clothianidin, and Thiamethoxam Mixtures Exert Greater than Additive Toxicity in Chironomus dilutus? Ecotoxicol. Environ. Saf. 2018, 156, 354–365. DOI: 10.1016/j.ecoenv.2018.03.003.
- Naveen, N. C.; Fojtova, D.; Blahova, L.; Rozmankova, E.; Blaha, L. Acute and (Sub)Chronic Toxicity of the Neonicotinoid Imidacloprid on Chironomus riparius. Chemosphere 2018, 209, 568–577. DOI: 10.1016/j.chemosphere.2018.06.102.
- Cavallaro, M. C.; Morrissey, C. A.; Headley, J. V.; Peru, K. M.; Liber, K. Comparative Chronic Toxicity of Imidacloprid, Clothianidin, and Thiamethoxam to Chironomus dilutus and Estimation of Toxic Equivalency Factors. Environ. Toxicol. Chem. 2017, 36, 372–382. DOI: 10.1002/etc.3536.
- Roessink, I.; Merga, L. B.; Zweers, H. J.; Van den Brink, P. J. The Neonicotinoid Imidacloprid Shows High Chronic Toxicity to Mayfly Nymphs. Environ. Toxicol. Chem. 2013, 32, 1096–1100. DOI: 10.1002/etc.2201.
- Stoughton, S. J.; Liber, K.; Culp, J.; Cessna, A. Acute and Chronic Toxicity of Imidacloprid to the Aquatic Invertebrates Chironomus tentans and Hyalella azteca under Constant- and Pulse-Exposure Conditions. Arch. Environ. Contam. Toxicol. 2008, 54, 662–673. DOI: 10.1007/s00244-007-9073-6.
- Mineau, P.; Palmer, C. Neonicotinoid Insecticides and Birds: The Impact of the Nation’s Most Widely Used Insecticides on Birds. American Bird Conservancy, Virginia, USA, 2013.
- Gerber, R.; Smit, N. J.; van Vuren, J. H. J.; Ikenaka, Y.; Wepener, V. Biomarkers in Tigerfish (Hydrocynus vittatus) as Indicators of Metal and Organic Pollution in Ecologically Sensitive Subtropical Rivers. Ecotoxicol. Environ. Saf. 2018, 157, 307–317. DOI: 10.1016/j.ecoenv.2018.03.091.
- Azevedo-Pereira, H. M. V. S.; Lemos, M. F. L.; Soares, A. M. Effects of Imidacloprid Exposure on Chironomus riparius Meigen Larvae: Linking Acetylcholinesterase Activity to Behavior. Ecotoxicol. Environ. Saf. 2011, 74, 1210–1215. DOI: 10.1016/j.ecoenv.2011.03.018.
- Connell, D.; Lam, P.; Richardson, B.; Wu, R. Introduction to Ecotoxicology. Oxford, United Kingdom: Blackwell Science Ltd; 1999.
- Tomizawa, M.; Lee, D. L.; Casida, J. E. Neonicotinoid Insecticides: Molecular Features Conferring Selectivity for Insect versus Mammalian Nicotinic Receptors. J. Agric. Food Chem. 2000, 48, 6016–6024. DOI: 10.1021/jf000873c.
- Kabeer, I. A. S.; Sailatha, D.; Rao, K. V. R. Impact of Malathion on Acetylcholinesterase in the Tissue of the Fish, Tilapia mossambica (Peters)—a Time Course Study. J. Biosci. 1980, 2, 37–41. DOI: 10.1007/BF02703131.
- Adams, S. M.; Greeley, M. S. Ecotoxicological Indicators of Water Quality: Using Multi-Response Indicators to Assess the Health of Aquatic Ecosystems. Water Air Soil Pollut. 2000, 123, 103–115. DOI: 10.1023/A:1005217622959.
- Kamdem, M. M.; Kubheka, N.; Nyoka, N.-K. Using Folsomia candida (Collembola) for the Ecological Assessment of Sediment Samples from Three Rivers, South Africa. International Journal of Energy and Water Resources 2024, 8, 1–11. https://doi.org/10.1007/s42108-024-00282-3.
- Kamdem, M. M.; Ramoejane, M.; Voua Otomo, P. Local-Scale DNA Barcoding of Afrotropical Hoverflies (Diptera: Syrphidae): a Case Study of the Eastern Free State of South Africa. Insects 2023, 14, 692. DOI: 10.3390/insects14080692.
- Kehinde, T.; Samways, M. J. Effects of Vineyard Management on Biotic Homogenization of Insect–Flower Interaction Networks in the Cape Floristic Region Biodiversity Hotspot. J. Insect Conserv. 2014, 18, 469–477. DOI: 10.1007/s10841-014-9659-z.