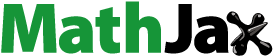
ABSTRACT
The aim of this study is to chemically, physically, and mechanically characterize Grewia bicolor (GB) fibers harvested in the NGONG locality located in the North Cameroon region for potential use in the elaboration of tannin matrix composites. The fibers are extracted from three zones of the stem (top (T), middle (M), and bottom (B)) by biological retting with stagnant water, and the various standard norms are respected for different tests. Analysis by Fourier transform infrared spectroscopy (FTIR) and chemical composition show that GB fibers consist mainly of cellulose (50.73–53.67%), hemicellulose (20.95–24.73%), and lignin (14.26–17.26%). Physical properties give: true density (1.33–1.35 g/cm3), bulk density (0.61–0.72 g/cm3), porosity (48.37–51.4%), linear density (17.28–29.44 tex), water content (13.16–13.59%), water absorption rate (121.86–134.54%), and a moisture recovery rate at 75% relative humidity (11.12–11.76%). Mechanical parameters give Young’s modulus (17.21–29.56 GPa), stress at break (504.71–936.09 MPa), and strain at break (4.65–5.37%). Analysis of variance (ANOVA) combined with Tukey’s test for mechanical properties show that the bottom and middle fibers are identical at the 95% confidence level. GB fibers can be used in the reinforcement of tannin matrix composites with preferable and indiscriminate sampling in the bottom and middle zones of the stem.
摘要
本研究的目的是对在位于喀麦隆北部地区的NGONG地区收获的Grewia双色(GB)纤维进行化学、物理和机械表征,以潜在地用于制备单宁基复合材料. 通过用死水生物脱胶,从茎的三个区域(顶部(T)、中间(M)和底部(B))提取纤维,并在不同的测试中遵守各种标准规范. 傅立叶变换红外光谱(FTIR)和化学成分分析表明,GB纤维主要由纤维素(50.73–53.67%)、半纤维素(20.95–24.73%)和木质素(14.26–17.26%)组成. 物理性质给出: 真实密度(1.33–1.35 g/cm3)、体积密度(0.61–0.72 g/cm3)、孔隙率(48.37–51.4%)、线密度(17.28–29.44 tex)、含水量(13.16–13.59%)、吸水率(121.86–134.54%)和75%相对湿度下的水分回收率(11.12–11.76%). 力学参数给出了杨氏模量(17.21–29.56 GPa)、断裂应力(504.71–936.09 MPa)和断裂应变(4.65–5.37%). 方差分析(ANOVA)结合Tukey的机械性能测试表明,底部和中间纤维在95%的置信水平下是相同的. GB纤维可用于单宁基复合材料的增强,在茎的底部和中部区域进行优选和不加选择的取样.
Introduction
The use of composite materials in various applications (transport, construction, medical, leisure) has seen considerable progress due to the excellent properties they offer (lightness, mechanical strength, ease of processing) (Djebloun Citation2018; Mbang et al. Citation2023; Noah et al. Citation2020). Plant fibers, which are one of its constituents, are of growing interest from both an economic and environmental point of view (Dallel Citation2012). They are becoming increasingly attractive thanks to their properties, such as good mechanical strength, low weight, biodegradability, and low cost (Hicham and Abdessalam Citation2019).
In the context of sustainable development, scientific research is focusing on limiting global warming and its effects by trying to find new, more environmentally friendly production and consumption alternatives (Vidil Citation2019). These involve not only the development of plant-based composites as substitutes for synthetic fibers (Bureaux Citation2011) or as an alternative solution (Betene Ebanda Citation2012), but also the use of eco-labeled resins (Ndiwe et al. Citation2019).
Synthetic resins made from petroleum waste, which generate toxic gases that have a direct impact on the destruction of the ozone layer (Anderson and Thornback Citation2012; Mbang et al. Citation2023; Morgenstern et al. Citation2018) are being replaced by bio-adhesives (Konai et al. Citation2015; Pizzi et al. Citation2009). Several research projects have developed adhesives of animal and plant origin (Rhazi Citation2015). Resins based on tannins, lignins, or proteins have been developed as adhesives for wood and have been used to develop composite materials with good environmental properties over the years (Mansouri, Pizzi, and Salvado Citation2007; Nenonene Citation2009; Ping, Gambier, et al. Citation2012; Ping, Pizzi, et al. Citation2012; Pizzi Citation2006; Santos et al. Citation2017; Sealy-Fisher and Pizzi Citation1992; Trosa and Pizzi Citation2001; Wedaïna et al. Citation2021; Xi et al. Citation2020). In addition, tannin-based resins, being the oldest to be marketed, have the advantage over the others of not requiring the addition of synthetic resin derived from petroleum products, as is the case for the others, which is of significant interest (Pizzi Citation2006; Pizzi et al. Citation2009; Thébault et al. Citation2014; Arias et al. Citation2022).
Various types of plant fibers, such as flax, jute, hemp, kenaf, and other natural fibers, are used as reinforcement in tannin matrix composites (Chupin Citation2014; Kueny Citation2013; Nicollin et al. Citation2012; Pizzi et al. Citation2009; Saad Citation2013; Sauget, Nicollin, and Pizzi Citation2013; Wedaïna et al. Citation2021). On the other hand, one of the limitations of using plant fibers in composite reinforcement is the wide variability of their properties (Magniont Citation2010, Ringuette Citation2011). This non-uniformity in their characteristics may be linked to their origin, the nature of the soil, and natural constraints (rainfall, climate) (Chengoue Mbouyap et al. Citation2023) the extraction method (Chengoué et al. Citation2020) plant maturity (Pickering et al. Citation2007) and experimental conditions (Dumont et al. Citation2017). Several authors have also shown that the sampling area can have an effect on the physical and mechanical properties of plant fibers (Betené et al. Citation2022; Charlet et al. Citation2007; Duval et al. Citation2011; Libog et al. Citation2023; Stawski et al. Citation2020; N. D. Yilmaz et al. Citation2016).
Grewia bicolor (GB) is a shrub or small tree that can reach 7 m to 14 m in height (Heuzé et al. Citation2015) producing shoots and branches from the base of the main trunk and belonging to the malvaceae family (Brink Citation2007). It is widespread in the dry zones of tropical Africa, throughout the Sudano-Sahelian zone and across East Africa. It is also found in Yemen, Saudi Arabia, to India (Fali et al. Citation2019). In Cameroon, the Grewia bicolor plant is found in the northern zone (Kossoumna Liba’a Citation2008; Seignobos and Tourneux Citation2002). This plant has several uses, depending on its parts: GB wood is used for building houses (poles, beams), making tools and edged weapons (tool handles, shepherd’s crooks, pike poles) (Ganaba, Ouadba, and Bognounou Citation2004) and also as firewood (Elie Citation2012). The bark is generally used to clarify muddy water and is also used to make ropes, which in some regions are used to make whips because of their strength (Heuzé et al. Citation2015).The mucilaginous leaves and leaf fibers are used as binders in sauces; fresh leaves are used to make an infusion and also as fodder for domestic animals (Augustino et al. Citation2011; Brink Citation2007). The leaves and ash from burnt leaves are sometimes used as soap and for cleaning clothes; nevertheless, the Grewia bicolor plant is a highly prized medicinal plant (Orwa et al. Citation2009). The Grewia bicolor plant has a wide range of applications in traditional African medicine (Augustino et al. Citation2011). GB fibers have yet to be exploited in the field of tannin matrix composites, yet they are available in Cameroon and have the advantage of appreciable strength due to their traditional applications. In addition, the work of (Ndiwe et al. Citation2023) has shown that GB fibers harvested in the Touboro locality (7° 46’8“North; 15° 20’ 49” East) have appreciable properties and have been used to develop polyester resin composites with good physical and mechanical properties. However, certain technical properties, such as the chemical composition, linear density, and moisture absorption of this fiber, remain unknown in the literature. What’s more, only fibers from the central part of the stems, chemically extracted in an aqueous solution containing 5% NaOH, have been used in the elaboration of said composites, due to the variability of fiber properties along the stem. The work of (Jamshaid et al. Citation2021) showed that it was possible to statistically study this variability in fiber properties at different locations on the plants for a better understanding of their behavior, and tools such as ANOVA (analysis of variance) with Tukey's post hoc test were used. It therefore seems necessary to conduct an experimental campaign in order to provide further information on this fiber and to better define the variability of fiber properties along the stem for potential more rational valorization in the development of tannin matrix composites. Furthermore, the properties of plant fibers are mainly influenced by their physical and chemical characteristics (ArunRamnath et al. Citation2023; Saba et al. Citation2016; Wambua, Ivens, and Verpoest Citation2003). The physical properties are, for example, the apparent density, the absolute density, the porosity, the linear mass, the humidity rate, the water absorption rate, and the humidity recovery (Betené et al. Citation2022; Libog et al. Citation2023; Ngoup et al. Citation2024; Soppie et al. Citation2023; Stawski et al. Citation2020; Yilmaz et al. Citation2016). The main chemical constituents of plant fibers are cellulose, hemicellulose, and lignin, which govern their mechanical properties (Ngoup et al. Citation2024). For the development of new ecological tannin matrix composites presenting the desired performance and functionality, it is necessary to have knowledge of the chemical, physical, and mechanical properties of the fibers.
The aim of this study was to determine the chemical, physical, and mechanical properties of Grewia bicolor fiber in order to assess its potential for the reinforcement of tannin matrix composites. GB stems were harvested in the NGONG locality, and the fibers were extracted in three zones of the stem (top (T), middle (M) and bottom (B)) by biological retting with stagnant water. Chemical characterization was carried out to determine the content of the main plant fiber constituents, cellulose, hemicellulose, and lignin, and FTIR analysis was used to identify functional groups. Physical characterization involved the determination of water content by gravimetry, moisture recovery, absolute density using a pycnometer, apparent density by application of the Archimedean buoyancy principle, porosity, linear density, and water absorption rate. Finally, the mechanical characterization involved determining the tensile properties of the fiber bundles, followed by an ANOVA statistical analysis.
Materials and methods
Fiber extraction
Grewia Bicolor (GB) stems, one-year-old, length ranging from 80 to 134 cm (), were harvested at Ngong (9°01’00’“North; 13°11’9”’ East), in the North Cameroon region, at a temperature of 23 ± 2°C. Inspired by the techniques of (Libog et al. Citation2023) as well as (Ndiwe et al. Citation2023), the stems were cut into three equal zones (): bottom(B), middle(T) and top(T). The diameters of the stems vary between 15 and 40 mm. They are larger at the bottom and decrease as you move toward the top. The stems from each part were stripped of their bark (), and then the bark was soaked in three containers containing 50 L of distilled water for 90 days at an ambient temperature of 25 ± 5°C (). The extraction technique used was that of biological retting with stagnant water. Once the extractives and lignins had decomposed, the bark was washed with distilled water until the fibers were obtained. Finally, the fibers obtained were dried at room temperature of 28 ± 5°C for 4 hours (), then stored in a chamber at 75% relative humidity at 25°C for the various characterizations.
Chemical characterization
Fourier transform infrared spectroscopy in attenuated total reflectance spectrometry (FTIRATR)
Fourier transform infrared (FTIR) spectroscopy was used to determine the functional groups and chemical bonds present on Grewia bicolor fiber for each part of the stem (bottom, middle, and top). The infrared spectrum was recorded using a BRUKER ALPHA spectrometer operating in attenuated total reflectance (ATR) mode and controlled by Opus Lab v 7.0 122 software. Acquisitions were made by performing 200 scans in the 4000 to 400 cm−1 frequency range, with a spectral resolution of 4 cm−1. Fiber bundles were chopped, ground and dried. A mass of 1 g was used for this purpose (Legrand et al. Citation2020; Libog et al. Citation2021).
Chemical composition
The composition of fibers such as cellulose, hemicelluloses, and lignin was determined in accordance with ASTM D 1104, ASTM D 1106 and ASTM D 1287 for fibers from the bottom, middle and top of the Grewia bicolor stem. The method used consists of successive solvent extraction of fiber components from a 2 g mass of ground and dried fibers. The first extraction was performed with an alcohol-toluene mixture, which will dissolve waxes and fats; then a second extraction was performed with hot water at 90°C, which will determine the amount of mineral salts as well as the low percentage of tannins, starch, and polysaccharides. Next, a third extraction was carried out for the separation of pectins using an aqueous ammonium oxalate extraction solution.
Lignins were then dissolved in a solution of sodium chlorite and glacial acetic acid. Finally, the hemicelluloses were solubilized with a potassium hydroxide solution, followed by a sodium hydroxide solution. By elimination, it was possible to determine the percentage of cellulose and hemicellulose. The values obtained were the average of three replicates of the fiber constituents (Mewoli et al. Citation2020).
Physical characterization
Water content
In a non-forced-air oven, ten fiber samples for each part of the stem (bottom, middle, and top) with an initial undried mass m0 = 5 g ±0.1 g, previously weighed using a digital scale with a sensitivity of 0.001 g, were dried for 24 hours at a temperature of 105◦C. The mass m1 is measured after drying, and according to AFNOR B 51,004, the moisture content TH (%) was determined by EquationEquation (1)(1)
(1) (Libog et al. Citation2023; Ndiwe et al. Citation2023).
Moisture absorption rate at 75% relative humidity
Ten fiber samples for each part of the stem (bottom, middle, and top) were oven-dried at 105°C for 3 hours, then weighed using a digital scale accurate to 1/1000th in order to take the various initial masses (Mi). Samples were conditioned in a sodium chloride chamber (Solubility: 357 g/L, 8 g in 20 ml water at 75% relative humidity at 20°C) for 24 hours (Betene Ebanda Citation2012) and weighed again to collect the different final masses (Mf). The chamber saturated with sodium chloride is prepared 24 hours before the start of the test in accordance with NF G 08-001-4. EquationEquation (2)(2)
(2) was used to determine the percentage moisture absorption (RH) of the fibers.
Density
Real density
The pycnometric method using toluene (ρa = 0.866 g.cm−3 at room temperature of 24 ± 2◦C) as the immersion liquid, in accordance with ASTM D 2320–98 (2003), was used to determine the true density () of Grewia bicolor fibers on five samples for each part of the stem (bottom, middle, and top) by applying relationship (3). Prior to measurement, the fibers were cut into 5 ± 1 mm lengths, then dehumidified at 105◦C for 24 h in the non-forced air oven (Ndiwe et al. Citation2023; Obame et al. Citation2022). Measurements were taken on a digital scale with a sensitivity of 0.1 mg.
Where: m0 is the mass of the empty pycnometer; m1 is the mass of the pycnometer filled with toluene at room temperature; m2 is the mass of the pycnometer containing the fiber sample; and m3 is the mass of the pycnometer filled with toluene and fiber at room temperature.
Apparent density
Five fiber clusters for each part of the stem (bottom, middle, and top) with a mass of 5 g were dried at 105°C in an oven for 6 hours in accordance with the ISO 3344 standard (Phung Citation2018). Density determination then involved weighing the mass of the samples using a 0.001 g precision balance. These samples were soaked in kerosene and weighed again to obtain the mass
. Then they were immersed in a beaker containing a volume
of distilled water. After the water had stabilized, the new volume
was read. The density was determined using EquationEquation (4)
(4)
(4) (Libog et al. Citation2021; Mewoli et al. Citation2020; Ndiwe et al. Citation2023).
With :
Where: is the bulk density of the fiber in g/cm3;
is the initial mass of the sample in g;
is the mass of the paraffin sample in g;
is the volume of distilled water in cm3;
is the volume after immersion of the fiber in cm3;
is the volume of the kerosene in cm3,
: kerosene density in g/cm3.
Porosity
The porosity of a material expresses the proportion of voids it contains in relation to its total volume. It is therefore equal to the relative difference between the apparent () and real density
. Porosity was determined using EquationEquation (5)
(5)
(5) for the fibers of each part of the stem (bottom, middle, and top).
Linear mass
ASTM D 1577–96 was used to determine the linear mass of the fibers gravimetrically, using EquationEquation (6)(6)
(6) . Forty fiber samples for each part of the stem (bottom, middle, and top) were cut to a size of 20 cm ±0.1 cm, and dried in a non-forced air oven at a temperature of 105◦C for 24 hours (Libog et al. Citation2023). Each sample was weighed on a 0.1 mg sensitivity balance at an ambient temperature of 24 ± 2◦C.
Water absorption rate
Ten fiber clusters for each part of the stem (bottom, middle, and top) with an initial dry mass of mi = 100 mg ±5 mg were weighed using a 1 mg sensitivity balance, then immersed in distilled water at a temperature of 32 ± 2°C for 24 hours, in accordance with ASTM D 2402 (ASTM 2001). The fibers were pre-dried in an oven at 105°C. Samples were dried for 3 hours, then conditioned and left to stand for 3 hours to cool. Once removed from the water, a dry cotton cloth was used to wipe excess water from the surface. The method used in this test was gravimetric, and EquationEquation (7)(7)
(7) was used to calculate the water absorption rate (TA%).
Mechanical characterization
The determination of Young’s modulus, stress, and strain at break of the fibers was carried out on thirty samples for each part of the stem (bottom, middle, and top) using a COYON CJ-0820 tensile testing machine with a load capacity of 5 kN as recommended by ASTMD 3822 (Betene et al. Citation2022; Obame et al. Citation2022). The fibers were processed at a controlled temperature of 23°C ±1, 50% relative humidity, and a displacement speed of 1 mm/min. The fibers were separated and attached to standard-sized supports, as shown in .
The various experimental points are obtained and processed in Microsoft Excel 2019. EquationEquations (8)(8)
(8) & (Equation9
(9)
(9) ) were used to plot the stress-strain curves that gave rise to equations of the type Y=Ax+B, where A and B were respectively the slope and the ordinate at the origin of the regression line for each sample in the elastic zone. Young’s modulus (MPa) was calculated by averaging the values of A for each sample.
Statistical analysis
An analysis of variance (ANOVA) was used to assess the significant or non-significant impact of the extraction zone on fiber tensile mechanical properties using IBM SPSS Statistic 27 software. 95% confidence level was adopted, and Tukey’s test completed this statistical analysis to quantitatively assess significant differences between different fiber extraction zones on the stem (Scarpa, Amroune, and Bezazi Citation2015).
Results and discussion
Chemical characteristics
Fourier transform infrared spectroscopy in attenuated total reflectance spectrometry (FTIRATR)
The spectrum resulting from the ATR-FTIR analysis of Grewia bicolor (GB) fiber is shown in , and the identification parameters are listed in .
Table 1. Characteristic positions of the FTIR bands on Grewia Bicolor (GB) fiber and their assignments.
All three zones show spectra similar in appearance to flax, sisal, and jute fibers (Del Rio et al. Citation2007; Garside and Wyeth Citation2003; Seki et al. Citation2019). The peak located at 3334 cm−1 is attributed to hydrogen bonds (OH) in the inter- and intramolecular cellulose network of free hydroxyl groups in hemicellulose (Kılınç et al. Citation2018; Legrand et al. Citation2020). This broad absorption band is characteristic of the presence of liquid water, more or less bound to the polymeric network constituted by natural fibers (Célino et al. Citation2014). The one observed at 2919 cm−1 is associated with asymmetric CH and CH2 stretching vibration in cellulosic and hemicellulosic components (Kılınç et al. Citation2018). The absorption band centered at 1674 cm−1 corresponds to the symmetrical ester group (C=O) stretching of carbonyl groups in hemicelluloses, also present in pectins and waxes.
The absorption peak at 1619 cm−1 is located at the (O-H) bond shear in free water (Dai and Fan Citation2011). The absorbance band at 1426 cm−1 is associated with the symmetrical CH bending present in cellulose (Moonart and Utara Citation2019). The peak located at 1032 cm−1 characteristic of the vibration of OH and C=O stretches is attributed to cellulose and lignin (Mouhoubi et al. Citation2012). The symmetrical stretching of the CH and OH bonds and the band at 889 cm−1 confirmed the presence of β 1,4 glucosidic cellulose (Betene et al. Citation2020). Thus, the above analysis clearly proved that GB fibers are mainly composed of cellulose, hemicellulose, lignin, and water. We can clearly see that the spectrum of top fiber is lower in intensity than the other two, which tend to be superimposed. This level of intensity would explain why the properties of the top fiber differ significantly from those of the bottom and middle fibers. This would be due to the lengthwise maturity of the plant. This result is in line with the mechanical characterization of the fibers in the three plant zones.
We can conclude that Grewia bicolor (GB) fiber is made up of cellulose, hemicellulose, lignin, pectin and other extractives. It would therefore be interesting to know the content of each constituent.
Chemical composition
The chemical composition of Grewia bicolor (GB) fibers as a function of the sampling area in the stem, compared with plant fibers from the literature, is presented in .
Table 2. Chemical composition of plant fibers.
The chemical composition of Grewia Bicolor(GB) fibers is similar to that of other plant fibers, with no significant difference in results between Middle and Bottom fibers. The amount of lignin is close to that of Triumfetta cordifolia (TC), banana and Recktophillum Camerunence (RC), while the amount of pectin and cellulose is close to that of TC. Grewia bicolor top fibers have a lower cellulose and lignin content than bottom and middle fibers, so their mechanical strength and stiffness could be lower. Previous studies on plant fibers show that increases in fiber properties such as tensile strength and Young’s modulus are closely linked to the amount of cellulose they contain (ArunRamnath et al. Citation2023). This cellulose content also has a positive influence on the reinforcement of tannin-matrix composites. Middle and bottom fibers with the highest cellulose values (52.52% and 53.67%) are more recommendable. It should be noted that these proportions of cellulose contained in GB fiber are higher than those of some fibers already used in the reinforcement of tannin matrix composites with good properties, such as Triumfetta cordifolia fiber (Mewoli et al. Citation2023), banana fiber (Konai et al. Citation2020), jute fiber (Chupin Citation2014; Kueny Citation2013), and kenaf fiber (Pizzi et al. Citation2009). This is an indicator that Grewia bicolor fiber can effectively reinforce tannin matrices. Hemicellulose has a negative effect on the tensile strength and Youngs’ modulus of the fibers (ArunRamnath et al. Citation2023); it may also compromise the mechanical strength and dimensional stability of the tannin matrix composite, as it is hygroscopic. Top fibers have a slightly higher hemicellulose content than middle and bottom fibers, making them less suitable than middle and bottom parts for reinforcing tannin matrix composites. On the other hand, the fibers of the three stem zones of Grewia bicolor have a lower hemicellulose content than Triumfetta cordifolia and banana fibers and are within the hemicellulose content range of flax, jute, and kenaf fibers already used in the reinforcement of tannin matrix composites. Nevertheless, alkaline treatment can eliminate amorphous hemicelluloses on the fiber and promote better compatibility and anchoring of GB fiber in tannin matrices, which can improve composite properties (Zhu, Abhyankar, and Njuguna Citation2013).
Physical properties
shows the results for water content, bulk density, true density, porosity, and water absorption rate of Grewia bicolor fibers, and illustrates the different behaviors.
Table 3. Physical properties of plant fibers.
Moisture content
Hemicellulose is a key factor in understanding the moisture content of a plant fiber; the higher it is, the more important it is. shows the results of the moisture content of Grewia bicolor (GB) fibers in comparison with other plant fibers. Fibers from different parts of the GB plant (bottom (B), top (T), and middle (M)) have moisture contents between 13.16% and 13.59%; these values are within the range of (Ndiwe et al. Citation2023). Middle(M) fibers from the GB have a lower moisture content than those from the other two zones, which is in agreement with the results of (Ndiwe et al. Citation2023). This finding can be explained by the fact that they have a lower hemicellulose content compared with the other two zones, as shown in . GB fibers have a higher water content than the other plants presented in , as they have a higher hemicellulose content. It would be necessary to remove this moisture from Grewia bicolor fibers before shaping tannin matrix composites, as moisture can adversely affect composite properties.
Moisture recovery at 75% relative humidity
Anhydrous fibers absorbed water in a 75% relative humidity chamber for 24 hours. Values of 11.12% ± 1.62, 11.42% ± 1.38, and 11.76% ± 0.63 were found for top, middle and bottom fibers respectively. The difference was not significant. The same applies to water content. These values are lower than those for Sida rhombifolia (21.02–21.45%) (Ngoup et al. Citation2024). This difference could be explained by the different nature of the two fibers. So Grewia bicolor fiber can be used in the same field of application as Sida rhombifolia.
Porosity
From , we can see that apparent and real densities increase as we move from Top to Bottom, and the values are respectively 0.61 g.cm−3 ±0.02 to 0.72 g.cm−3 ±0.03 and 1.25 g.cm−3 ±0.04 to 1.39 g.cm−3 ±0.07. These values are quite close to those of (Ndiwe et al. Citation2023). However, the behavior is different, which could be explained by the difference in botanical origin of the Grewia bicolor (GB) stems as well as their age and experimental conditions. This evolution according to the zone on the stem is also the opposite of the results of (Libog et al. Citation2023) who reported an increase from bottom to top for Cavendish bananas. This finding could be explained by the different nature of GB and banana. There was also no significant difference between the bottom and middle densities. The density considered in the industry is the real one, and GB densities are close to those of jute, ramie, banana, hemp, and flax fibers; GB fibers can be used in the same fields of application as the latter (Ndiwe et al. Citation2023) report that the porosity of GB fibers decreases as one moves from the head to the foot of the stem. The porosities of GB fibers in this study are 51.40%, 48.07%, and 48.37%, respectively for top, middle and bottom. These values are close to those of (Ndiwe et al. Citation2023) as well as their behavior. It was found that the porosity between the middle and bottom fibers is almost identical and lower than that of the top, which would lead us to say that the top fibers are lighter, resulting in a lower density, and will also absorb more water. These findings also suggest that bottom and middle fibers will have superior mechanical properties (stiffness, deformation, and stress) to top fibers. The bottom and middle fibers have a greater density than the jute fibers (Asim et al. Citation2015) and banana fibers (Ronald Aseer et al. Citation2013) used in tannin matrix composites. It would therefore be advisable to reinforce tannin matrix composites with GB fibers from the bottom and middle because a denser plant fiber has the capacity to support mechanical loads, which increases stiffness. Furthermore, they can contribute to the better dimensional stability of the tannin matrix composite by minimizing variations due to humidity and other environmental factors.
Linear mass
The linear density is a very important parameter for the textile application of a plant fiber. The values for Grewia bicolor (GB) for different zones are respectively 29.44 tex ± 1.66, 28.1 tex ±1.37, and 17.28 tex ±1.98 for the bottom, middle and top ().
The linear mass of GB is higher at the bottom and lower at the top. This can be explained by the porosity of the GB fiber, which is greater at the top than at the bottom. Furthermore, the more porous the fiber, the less weight it will have along its length. Regardless of the sampling area on the stem, GB fibers have a higher linear density than pineapple leaf (8–8.4 tex) (Betene et al. Citation2022) and lower than banana top, middle, and bottom (31.6–34 tex) (Libog et al. Citation2023). GB fibers can also be used in textiles. It is also observed that the linear density of the bottom and middle fibers is almost identical and higher than that of the top. These observations are made on porosity, density, and water absorption rate (Yilmaz et al. Citation2016) for corn fibers (Singh et al. Citation2020), for nettle leaf fibers, and (Libog et al. Citation2023) for banana fibers, reported instead an increase in linear density from bottom to top. This may be related to plant growth, which causes voids in mature areas to shrink in younger areas of the plant (Betene et al. Citation2022, Sikame et al. Citation2014; Libog et al. Citation2023). The fibers at the top are finer than those at the middle and bottom. Middle and bottom fibers can be an advantage, as they can lead to a more uniform distribution of loads within the tannin matrix composite, not only strengthening its overall structure but also reducing stress concentration zones that could lead to premature failure zones.
Water absorption rate
Plant fibers with a water absorption rate equal to or greater than 100% have a hydrophilic character due to the presence of hemicellulose in their structure (Mewoli et al. Citation2020). It’s clear to see that Grewia bicolor (GB) fibers are hydrophilic (absorption rate between 121.86% and 153.59%), and the sampling area influences the water absorption rate (). This ability to absorb water reduces the material’s rigidity in composite construction (Libog et al. Citation2021; Mewoli et al. Citation2020). This characteristic can be corrected by using a surface treatment or a coupling agent (Betene et al. Citation2020). The absorption rate values in this study are well above those of (Ndiwe et al. Citation2023). This may be explained by the difference in plant harvesting location and maturity. The porosity and higher hemicellulose content of the top zone explain why it has a higher absorption rate than the other zones (153.59%). An increase in the rate of water absorption from the bottom to the top was also observed. This could be explained by the more efficient capillary effect from the bottom to the top of the GB plant. Such a finding was made by (Libog et al. Citation2023) for Cavendish banana, (Sikame et al.,2014) for raffia, and (Yilmaz et al. Citation2016) for maize. Grewia bicolor fibers have a lower absorption rate than banana fibers (347–517%) (Libog et al. Citation2023), sisal fibers (1982.2%) (Asim et al. Citation2015; Béakou et al. Citation2008), and Triumfeta cordifolia fibers (342.3%) (Mewoli et al. Citation2023). These fibers have already been used to reinforce a tannin matrix composite, so given the low absorption rate of Grewia bicolor fibers, their adhesion to a tannin matrix will be more favorable.
Mechanical properties and statistical analysis
shows that Grewia bicolor (GB) fibers have a type 3 brittle mechanical behavior also encountered in the case of Triumfetta cordifolia fibers (Soppie et al. Citation2023) This is justified by the fact that the deformations of the GB are of the order of less than 10%; moreover, the first linear zone marks the alignment of the microfibrils on the tensile axis, and the second linear zone is the elastic zone for the determination of Young’s modulus (Soppie et al. Citation2023). shows the mechanical properties of GB fibers in relation to other plant fibers, depending on the extraction area.
Table 4. Mechanical properties of study fibers compared with other plant fibers.
The Young’s modulus, stress, and strain at break of GB fibers change with the extraction zone (). Young’s modulus and stress at break decrease when moving from bottom to top, while strain at break increases (). This observation was made by (Ndiwe et al. Citation2023) for Grewia bicolor extracted at Touboro and also by (Libog et al. Citation2023; Subramanya, Satyanarayana, and Shetty Pilar Citation2017) for the case of banana stem. This evolution of mechanical properties on the GB stem could be explained by the fact that the degree of maturity of the plant decreases from bottom to top, as in the case of bananas (Subramanya, Satyanarayana, and Shetty Pilar Citation2017). The amount of cellulose in many plant fibers also depends on their degree of maturity, which could indicate or justify their high stress and deformation. In addition, the mechanical behavior of GB fibers is in agreement with the chemical composition values in . The richer the cellulose, the greater the mechanical strength; the less rich the lignin, the less rigid (lower Young’s modulus). The evolution of the mechanical behavior of GB fibers differs from that of pineapple leaves (Betene et al. Citation2022) and flax (Charlet et al. Citation2007). This possible difference can be attributed to the origin of the plant.
Compared to the mechanical properties of (Ndiwe et al. Citation2023) shows that GB fibers have weaker properties. This could be explained by the difference in age, which affects the chemical composition of the fiber (Yilmaz et al. Citation2017) as well as experimental parameters (Obame et al. Citation2022, Citation2022).
The descriptive statistics in indicate that there is a difference between the mean values of Young’s modulus, stress, and strain at break depending on the location of the fiber on the GB rod. However, dispersions around the mean have been neglected for such an assertion; an ANOVA statistical analysis test () will enable us to tell with a 95% confidence level whether there is a significant difference between the values of the mechanical parameters (Mishra et al. Citation2022).
Table 5. Results of analysis of variance (ANOVA) test for mechanical properties of study fibers.
Here, we observe () that there is a significant difference depending on the location of the fiber on the rod for Young’s modulus (p = .013 < 0.05), stress at break (p = .018 < 0.05) and strain at break (p = .018 < 0.05). Unfortunately, the ANOVA test does not allow us to say how this significant difference exists between the different zones for each parameter. For this, a Tukey test with a rejection threshold of 5% (Libog et al. Citation2023) can clearly solve this problem ().
Table 6. Results of Tukey’s analysis test for mechanical properties of studied fibers.
When the significant value is less than 0.05, there is a significant difference between the data; otherwise, the difference is not significant at 95% confidence. Whatever the pair (A, B), the significant value is different. This result was also found for bananas (Libog et al. Citation2023). In the case of stress at break, we find that the difference (A-B) is negative between top-middle and top-bottom, and the significant value is less than 0.05; we can conclude that the stress at break of Top fibers has a significant difference with Bottom and Middle, and is lower than the latter. There is no significant difference between the Bottom and Middle fibers (p = .8 > 0.05). There is no significant difference between bottom and middle fibers in the case of Young’s modulus (p = .37 > 0.05). However, there was a significant difference between top-middle and top-bottom. The greater the density of the fibers, the more it is observed that the Young’s modulus and the breaking stress also increase, which corroborates with the evolution of the porosity. The denser the fiber, the less porous it is and the better mechanical properties it has. It can be concluded that the middle and bottom fibers are identical in terms of mechanical properties.
Conclusion
Investigation into the understanding of the chemical, physical, and mechanical properties of Grewia bicolor (GB) fibers according to the location of the parts on the stem points to their possible use in the elaboration of tannin matrix composites. Statistical analysis (ANOVA) using Tukey’s 95% confidence test shows that the mechanical properties of the bottom and middle zones do not vary significantly from those of the top. Indiscriminate sampling of fibers in the bottom and middle parts of the GB stem is more advisable for the development of tannin matrix composites. It would be interesting to continue this study by carrying out a chemical treatment to further improve the properties of the GB fiber, which could improve fiber-tannin matrix adhesion.
Author’s contribution
Mbere Taoga Michel: Interpretation of results, drafting and reading of manuscript; Betene Ebanda Fabien: Supervision of work, validation of tests and reading of manuscript; Pizzi Antonio : Methodology, reading of manuscript; Ndiwe Benoit: Supervision of work, validation of tests and reading of manuscript, Noah Pierre Marcel Anicet: Supervision of work, validation of tests and reading of manuscript; Essome Mbang Jonas Peequeur: Interpretation of results, drafting and reading of manuscript; Kibong Kimbi Kevin and Etinge Tabi Akwo :Data collection, César Segovia: Investigation into methodology, reading of manuscript
Conflicts and interests
The authors declare no conflict of interest regarding the publication of this article.
Ethical approval
The authors confirm that this work has no ethical problems.
The authors declare no conflict of interest regarding the publication of this article.
Major points
-The plant fibers come from NGONG in the North Cameroon region;
-The influence of the physical, chemical and mechanical properties was determined according to the location of the fiber on the Grewia bicolor(GB) stem;
-Statistical analysis by ANOVA was used;
-The study fiber is a major potential for tannin matrix composites.
Acknowledgments
The authors would like to thank Mr. Ngue Alain (Second Grade Technical High School Teacher in Mechanical Manufacturing), Mr. Mansassou Claude (Second Grade Technical High School Teacher in Mechanical Manufacturing) and Mr. Temwa Jean Marc (Engineering student at Ecole Nationale Polytechnique de Douala) for their help during the experimental campaign. We would also like to thank Dr. Mewoli Edwige Armel and Mr. Ndounakon Bissiliken Alphonse (PhD student in mechanical engineering) for their support and practical advice in this work. The authors would like to thank Ms. Biloa Otiti Sandrine (PhD student in mechanical engineering) and Ms. Nga Liliane (PhD student in forestry engineering) for their material contributions. Finally, we thank the reviewers of this manuscript for their critical contributions.
Disclosure statement
No potential conflict of interest was reported by the author(s).
Additional information
Funding
References
- Amiri, A., Z. Triplett, A. Moreira, N. Brezinka, M. Alcock, and C. A. Ulven. 2017. “Standard Density Measurement Method Development for Flax Fiber.” Industrial Crops and Products 96:196–21. https://doi.org/10.1016/j.indcrop.2016.11.060.
- Anderson, J., and J. Thornback. 2012. “A Guide to Understanding the Embodied Impacts of Construction Products.” Construction Products Association 12:2013.
- Arias, A., S. González-García, G. Feijoo, and M. T. Moreira. 2022. “Tannin-Based Bio-Adhesives for the Wood Panel Industry As Sustainable Alternatives to Petrochemical Resins.” Journal of Industrial Ecology 26 (2): 627–642. https://doi.org/10.1111/jiec.13210.
- ArunRamnath, R., S. Murugan, M. Sanjay, A. Vinod, S. Indran, A. Y. Elnaggar, A. M. Fallatah, and S. Siengchin. 2023. “Characterization of Novel Natural Cellulosic Fibers from Abutilon Indicum for Potential Reinforcement in Polymer Composites.” Polymer Composites 44 (1): 340–355. https://doi.org/10.1002/pc.27100.
- Asim, M., K. Abdan, M. Jawaid, M. Nasir, Z. Dashtizadeh, M. Ishak, and M. E. Hoque. 2015. “A Review on Pineapple Leaves Fibre and Its Composites.” International Journal of Polymer Science 2015:1–16. https://doi.org/10.1155/2015/950567.
- Augustino, S., J. B. Hall, F. B. Makonda, and R. C. Ishengoma, 2011. Medicinal Resources of the Miombo Woodlands of Urumwa, Tanzania: Plants and Its Uses.
- Béakou, A., R. Ntenga, J. Lepetit, J. A. Atéba, and L. O. Ayina. 2008. “Physico-Chemical and Microstructural Characterization of “Rhectophyllum camerunense” Plant Fiber.” Composites Part A, Applied Science and Manufacturing 39 (1): 67–74. https://doi.org/10.1016/j.compositesa.2007.09.002.
- Beldjoud, N., and F. Ghadbane. 2020. “L’impact du traitement écologique à base du bicarbonate de sodiumsur les propriétés des fibre végétales.” PhD Thesis, Univ M’sila.
- Betene, A. D. O., F. E. Betene, F. Martoïa, P. J. Dumont, A. Atangana, and P. M. A. Noah. 2020. “Physico-Chemical and Thermal Characterization of Some Lignocellulosic Fibres: Ananas Comosus (AC), Neuropeltis Acuminatas (NA) and Rhecktophyllum Camerunense (RC).” Journal of Minerals and Materials Characterization and Engineering 8 (4): 205–222. https://doi.org/10.4236/jmmce.2020.84014.
- Betené, A. D. O., F. E. Betené, F. E. Ngali, P. M. A. Noah, B. Ndiwé, A. G. Soppie, A. Atangana, and R. Moukené. 2022. “Influence of Sampling Area and Extraction Method on the Thermal, Physical and Mechanical Properties of Cameroonian Ananas Comosus Leaf Fibers.” Heliyon 8 (8): e10127. https://doi.org/10.1016/j.heliyon.2022.e10127.
- Betene Ebanda, F. 2012. “Study of the Mechanical and Thermal Properties of Plaster Reinforced with Tropical Plant Fibers.” PhD Thesis, Clermont-Ferrand 2.
- Brink, M., ed. 2007. Grewia bicolor juss, in: Ressources végétales de l’Afrique tropicale 7(1), Bois D’oeuvre 1, 335–337. Geningen, Netherlands: PROTA Foundation.
- Bureaux, H. 2011. “Availability and Accessibility of Plant Fibers for Material Uses in France 84”.
- Célino, A., O. Gonçalves, F. Jacquemin, and S. Fréour. 2014. “Qualitative and Quantitative Assessment of Water Sorption in Natural Fibers Using ATR-FTIR Spectroscopy.” Carbohydrate Polymers 101:163–170. https://doi.org/10.1016/j.carbpol.2013.09.023.
- Charlet, K., C. Baley, C. Morvan, J. P. Jernot, M. Gomina, and J. Bréard. 2007. “Characteristics of Hermès Flax Fibres As a Function of Their Location in the Stem and Properties of the Derived Unidirectional Composites.” Composites Part A, Applied Science and Manufacturing 38 (8): 1912–1921. https://doi.org/10.1016/j.compositesa.2007.03.006.
- Chengoue Mbouyap, A., T. Tchotang, C. Fokam Bopda, H. L. Assonfack, J. B. Saha Tchinda, and M. A. Cheumani Yona. 2023. “Impact of Cultivation Area on the Physical, Chemical, and Mechanical Properties of Banana Pseudo-Stems Fibers in Cameroon.” Journal of Natural Fibers 20 (2): 2198274. https://doi.org/10.1080/15440478.2023.2198274.
- Chengoué, A. M., T. Tchotang, C. B. Fokam, and B. Kenmeugne. 2020. “Influence of Extractions Techniques on the Physico-Mechanical Properties of Banana Pseudo-Stem Fibers.” Journal of Materials and Environmental Science 11:1121–1128. https://www.jmaterenvironsci.com/Document/vol11/vol11_N7/JMES-2020-1198-Chengoue.pdf.
- Chupin, L. 2014. “Study of the Extraction of Tannins from Maritime Pine Bark for the Development of Tannin-Lignosulfonate Glues.” PhD Thesis, Pau.
- Dai, D., and M. Fan. 2011. “Investigation of the Dislocation of Natural Fibres by Fourier-Transform Infrared Spectroscopy.” Vibrational Spectroscopy 55 (2): 300–306. https://doi.org/10.1016/j.vibspec.2010.12.009.
- Dallel, M. 2012. “Evaluation of the Textile Potential of Alfa Fibers (Stipa Tenacissima L.): Physicochemical Characterization from Fiber to Yarn.” PhD Thesis, Université de Haute AlsaceMulhouse.
- Del Rio, J. C., A. Gutiérrez, I. M. Rodríguez, D. Ibarra, and A. T. Martinez. 2007. “Composition of Nonwoody Plant Lignins and Cinnamic Acids by Py-GC/MS, Py/TMAH and FT-IR.” Journal of Analytical and Applied Pyrolysis 79 (1–2): 39–46. https://doi.org/10.1016/j.jaap.2006.09.003.
- Djebloun, Y. 2018. “Contribution à la caractérisation des matériaux composites renforcés de fibres végétales.” doctoral, UNIVERSITE MOHAMED KHIDER BISKRA.
- Dumont, P., L. OrgéOrgéAs, F. Martoïa, T. Budtova, and M. Vincent. 2017. “Polymer Composites and Lignocellulosic Fibers-Properties, Processing and Characterization.” Fr Berzin 160–201.
- Duval, A., A. Bourmaud, L. Augier, and C. Baley. 2011. “Influence of the Sampling Area of the Stem on the Mechanical Properties of Hemp Fibers.” Materials letters 65 (4): 797–800. https://doi.org/10.1016/j.matlet.2010.11.053.
- Elie, A. 2012. “Firewood Potential Production of Three Sahelian Woody Species (Grewia Bicolor, Pterocarpus Lucens and Combretum Glutinosum) in Ferlo (Northern Senegal).” Advances in Environmental Biology 6:2329–2334. https://www.researchgate.net/profile/Kindomihou-Valentin/publication/259502642_Firewood_potential_production_of_three_sahelian_woody_species_Grewia_bicolor_Pterocarpus_lucens_and_Combretum_glutinosum_in_Ferlo_Northern_Senegal/links/0deec52c56e50d30dd000000/Firewood-potential-production-of-three-sahelian-woody-species-Grewia-bicolor-Pterocarpus-lucens-and-Combretum-glutinosum-in-Ferlo-Northern-Senegal.pdf.
- Essabir, H., R. Boujmal, M. O. Bensalah, D. Rodrigue, R. Bouhfid, and A. E. K. Qaiss. 2016. “Mechanical and Thermal Properties of Hybrid Composites: Oil-Palm Fiber/Clay Reinforced High Density Polyethylene.” Mechanics of Materials 98:36–43. https://doi.org/10.1016/j.mechmat.2016.04.008.
- Fali, A., S. T. White, T. G. Folland, M. He, N. A. Aghamiri, S. Liu, J. H. Edgar, J. D. Caldwell, R. F. Haglund, and Y. Abate. 2019. “Refractive Index-Based Control of Hyperbolic Phonon-Polariton Propagation.” Nano Letters 19 (11): 7725–7734. https://doi.org/10.1021/acs.nanolett.9b02651.
- Faruk, O., A. K. Bledzki, H.-P. Fink, and M. Sain. 2012. “Biocomposites Reinforced with Natural Fibers: 2000–2010.” Progress in Polymer Science 37 (11): 1552–1596. https://doi.org/10.1016/j.progpolymsci.2012.04.003.
- Ganaba, S., J. M. Ouadba, and O. Bognounou. 2004. “House-Building Plants in the Sahelian Region.” Bois Forets Trop 282:11–17.
- Garside, P., and P. Wyeth. 2003. “Identification of Cellulosic Fibres by FTIR Spectroscopy-Thread and Single Fibre Analysis by Attenuated Total Reflectance.” Studies in Conservation 48 (4): 269–275. https://doi.org/10.1179/sic.2003.48.4.269.
- Gurunathan, T., S. Mohanty, and S. K. Nayak. 2015. “A Review of the Recent Developments in Biocomposites Based on Natural Fibres and Their Application Perspectives.” Composites Part A: Applied Science and Manufacturing 77:1–25. https://doi.org/10.1016/j.compositesa.2015.06.007.
- Heuzé, V., G. Tran, R. Delagarde, D. Bastianelli, and F. Lebas. 2015. False Brandy Bush (Grewia Bicolor).
- Hicham, A., and S. Abdessalam. 2019. “Caractérisation et élaboration d’un matériaux composites Jute (déchets)/Polyester.” Thesis, Université de MOHAMED BOUDIAF - M’SILA.
- Jamshaid, H., U. Hussain, R. Mishra, M. Tichy, and M. Muller. 2021. “Turning Textile Waste into Valuable Yarn.” Cleaner Engineering and Technology 5:100341. https://doi.org/10.1016/j.clet.2021.100341.
- Jeyapragash, R., V. Srinivasan, and S. Sathiyamurthy. 2020. “Mechanical Properties of Natural Fiber/Particulate Reinforced Epoxy Composites–A Review of the Literature.” Materials Today: Proceedings 22:1223–1227. https://doi.org/10.1016/j.matpr.2019.12.146.
- Kılınç, A. Ç., S. Köktaş, Y. Seki, M. Atagür, R. Dalmış, Ü. H. Erdoğan, A. A. Göktaş, and M. Ö. Seydibeyoğlu. 2018. “Extraction and Investigation of Lightweight and Porous Natural Fiber from Conium Maculatum As a Potential Reinforcement for Composite Materials in Transportation.” Composites Part B Engineering 140:1–8. https://doi.org/10.1016/j.compositesb.2017.11.059.
- Konai, N., A. Pizzi, R. Danwe, M. Lucien, and K. Tapsia. 2020. “Thermomechanical Analysis of African Tannins Resins and Biocomposite Characterization.” Journal of Adhesion Science and Technology 35 (14): 1492–1499. https://doi.org/10.1080/01694243.2020.1850611.
- Konai, N., A. Pizzi, D. Raidandi, M. Lagel, C. L’Hostis, C. Saidou, A. Hamido, S. Abdalla, F. Bahabri, and A. Ganash. 2015. “Aningre (Aningeria Spp.) Tannin Extract Characterization and Performance As an Adhesive Resin.” Industrial Crops and Products 77:225–231. https://doi.org/10.1016/j.indcrop.2015.08.053.
- Kossoumna Liba’a, N. 2008. “De la mobilité à la sédentarisation : gestion des ressources naturelles et des territoires par les éleveurs Mbororo au Nord du Cameroun.” PhD Thesis, UPV.
- Kueny, R. 2013. “Biocomposites: High-Tech Composites with Natural Fiber Reinforcement and Natural Resin Matrix.” PhD Thesis, University of Lorraine.
- Legrand, N. B. R., O. Fabien, B. E. Pierre, N. P. Marcel, and A. A. Jean. 2020. “Physico-Chemical and Thermal Characterization of a Lignocellulosic Fiber, Extracted from the Bast of Cola Lepidota Stem.” Journal of Minerals and Materials Characterization and Engineering 8 (5): 377–392. https://doi.org/10.4236/jmmce.2020.85024.
- Libog, L., J. Aime, N. Joseph, B. Ndiwe, L. Meva’a, A. Ateba, and L. Laurent. 2021. “Physico-Chemical and Thermal Characterization of the Banana Pseudo-Stem Fibers (BF).” European Journal of Experimental Biology 9:33–52. https://www.researchgate.net/publication/353347593_PHYSICO-CHEMICAL_AND_THERMAL_CHARACTERIZATION_OF_THE_BANANA_PSEUDO-STEM_FIBERS_BF?_tp=eyJjb250ZXh0Ijp7ImZpcnN0UGFnZSI6ImhvbWUiLCJwYWdlIjoic2VhcmNoIiwicG9zaXRpb24iOiJwYWdlSGVhZGVyIn19.
- Libog, L., F. Biyeme, A. Betené, A. Biwolé, B. Ndiwe, J. P. Essome Mbang, C. Takoumbe, J. Aimé Mbey, and J. Meva’a. 2023. “Influence of the Extraction Location on the Physical and Mechanical Properties of the Pseudo-Trunk Banana Fibers.” Journal of Natural Fibers 20 (2). https://doi.org/10.1080/15440478.2023.2204451.
- Li, X., L. G. Tabil, and S. Panigrahi. 2007. “Chemical Treatments of Natural Fiber for Use in Natural Fiber-Reinforced Composites: A Review.” Journal of Polymers and the Environment 15 (1): 25–33. https://doi.org/10.1007/s10924-006-0042-3.
- Magniont, C. 2010. “Contribution à la formulation et à la caractérisation d’un écomatériau de construction à base d’agroressources.” PhD Thesis, Toulouse 3.
- Mansouri, N.-E.-E., A. Pizzi, and J. Salvado. 2007. “Lignin-Based Polycondensation Resins for Wood Adhesives.” Journal of Applied Polymer Science 103 (3): 1690–1699. https://doi.org/10.1002/app.25098.
- Marimuthu, K. P., S. M. Kumar, V. R. Kumar, and H. K. Govindaraju. 2019. “Characterization of Mechanical Properties of Epoxy Reinforced with Glass Fiber and Coconut Fiber.” Materials Today: Proceedings 16:661–667. https://doi.org/10.1016/j.matpr.2019.05.143.
- Mbang, J. P. E., P. M. A. Noah, L. Libog, T. Ngoup, M. M. Taoga, and F. B. Ebanda. 2023. “State of Knowledge on Starch As an Alternative Solution to Petrochemical Resources—A Review.” Journal of Minerals and Materials Characterization and Engineering 11 (2): 49–62. https://doi.org/10.4236/jmmce.2023.112005.
- Mewoli, A. E., C. Segovia, F. B. Ebanda, A. Ateba, P. M. A. Noah, B. Ndiwe, and A. E. Njom. 2020. “Physical-Chemical and Mechanical Characterization of the Bast Fibers of Triumfetta Cordifolia A.Rich. from the Equatorial Region of Cameroon.” Journal of Minerals and Materials Characterization and Engineering 8 (4): 163–176. https://doi.org/10.4236/jmmce.2020.84011.
- Mewoli, A. E., C. Segovia, A. E. Njom, F. B. Ebanda, J. J. Eyinga Biwôlé, C. Xinyi, A. Ateba, P. Girods, A. Pizzi, and N. Brosse. 2023. “Characterization of Tannin Extracted from Aningeria Altissima Bark and Formulation of Bioresins for the Manufacture of Triumfetta Cordifolia Needle-Punched Nonwovens Fiberboards: Novel Green Composite Panels for Sustainability.” Industrial Crops and Products 206:117734. https://doi.org/10.1016/j.indcrop.2023.117734.
- Mishra, S. S., T. Mohapatra, S. S. Sahoo, and P. Mishra. 2022. “Overall Performance Investigation and Optimization of a Multi-Fuel Operated Compression Ignition Engine Using Coupled Taguchi and Grey Relational Analysis.” American Chemical Society Omega 7 (37): 33216–33232. https://doi.org/10.1021/acsomega.2c03566.
- Moonart, U., and S. Utara. 2019. “Effect of Surface Treatments and Filler Loading on the Properties of Hemp Fiber/Natural Rubber Composites.” Cellulose 26 (12): 7271–7295. https://doi.org/10.1007/s10570-019-02611-w.
- Morgenstern, O., K. A. Stone, R. Schofield, H. Akiyoshi, Y. Yamashita, D. E. Kinnison, R. R. Garcia, et al. 2018. “Ozone Sensitivity to Varying Greenhouse Gases and Ozone-Depleting Substances in CCMI-1 Simulations.” Atmospheric Chemistry & Physics 18 (2): 1091–1114. https://doi.org/10.5194/acp-18-1091-2018.
- Mouhoubi, S., H. Osmani, T. Bali, and S. Abdeslam. 2012. “Elaboration and Properties Study of Treated and Untreated Polyester-Alfa Composites.”
- Nam, S., and A. N. Netravali. 2006. “Green Composites. I. Physical Properties of Ramie Fibers for Environment-Friendly Green Composites.” Fibers and Polymers 7 (4): 372–379. https://doi.org/10.1007/BF02875769.
- Ndiwe, B., N. Konai, A. D. O. Betené, A. Pizzi, A. G. Wedaïna, F. Nzogning, A. Mewoli, et al. 2023. “Characterization of Grewia Bicolor Fibre and Its Use in the Development of Composites.” International Wood Products Journal 14 (2): 1–13. https://doi.org/10.1080/20426445.2023.2223936.
- Ndiwe, B., A. Pizzi, B. Tibi, R. Danwe, N. Konai, and S. Amirou. 2019. “African Tree Bark Exudate Extracts As Biohardeners of Fully Biosourced Thermoset Tannin Adhesives for Wood Panels.” Industrial Crops and Products 132:253–268. https://doi.org/10.1016/j.indcrop.2019.02.023.
- Nenonene, A. 2009. “Development and Mechanical Characterization of Kenaf Stem Particleboards and Bioadhesives Based on Bone Glue, Tannin or Mucilage.” PhD Thesis.
- Ngoup, T., N. D. Efeze, T. Kanaa, J. P. E. Mbang, S. César, and N. Nnanga, et N. Ebénézer. 2024. “Physical, Chemical and Mechanical Characterization of Sida Rhombifolia Fibers from the Center Region of Cameroon for Their Potential Use in Textiles and Composites.” Journal of Natural Fibers 21 (janvier). https://doi.org/10.1080/15440478.2023.2294478.
- Nicollin, A., R. Kueny, L. Toniazzo, and A. Pizzi. 2012. “High Density Biocomposite from Natural Fibers and Tannin Resin.” Journal of Adhesion Science and Technology 26 (10–11): 1537–1545. https://doi.org/10.1163/156856111X618399.
- Noah, P. M. A., E. F. Betene, S. V. Obame, B. Allasra, M. C. Bindjeme, and A. Atangana. 2020. “Elaboration and Characterization of a Hybrid Composite Material with Two Particles of the Same Size: Coco Shells and Palm Shells.” Open Journal of Composite Materials 10 (4): 77–91. https://doi.org/10.4236/ojcm.2020.104006.
- Obame, S. V., A. D. O. Betené, P. M. Naoh, F. E. Betené, and A. Atangana. 2022. “Characterization of the Neuropeltis Acuminatas Liana Fiber Treated As Composite Reinforcement.” Results in Materials 16:100327. https://doi.org/10.1016/j.rinma.2022.100327.
- Orwa, C., A. Mutua, R. Kindt, R. Jamnadass, and A. Simons. 2009. Agroforestree Database: A Tree Reference and Selection Guide. Version 4.
- Pereira, P. H. F., M. D. F. Rosa, M. O. H. Cioffi, K. C. C. D. C. Benini, A. C. Milanese, H. J. C. Voorwald, and D. R. Mulinari. 2015. “Vegetal Fibers in Polymeric Composites: A Review.” Polímeros 25 (1): 9–22. https://doi.org/10.1590/0104-1428.1722.
- Phung, T. A. 2018. “Formulation and Characterization of an Earth-Plant Fiber Composite: Bauge.” PhD Thesis, Normandie Université.
- Pickering, K. L., G. W. Beckermann, S. N. Alam, and N. J. Foreman. 2007. “Optimising Industrial Hemp Fibre for Composites.” Composites Part A, Applied Science and Manufacturing 38 (2): 461–468. https://doi.org/10.1016/j.compositesa.2006.02.020.
- Ping, L., F. Gambier, A. Pizzi, Z. D. Guo, and N. Brosse. 2012. “Wood Adhesives from Agricultural Byproducts: Lignins and Tannins for the Elaboration of Particleboards.” Cellulose Chemistry and Technology 46:457–462. https://scholar.google.com/scholar?hl=fr&as_sdt=0%2C5&q=Wood+Adhesives+from+Agricultural+Byproducts%3A+Lignins+and+Tannins+for+the+Elaboration+of+Particleboard&btnG=.
- Ping, L., A. Pizzi, Z. D. Guo, and N. Brosse. 2012. “Condensed Tannins from Grape Pomace: Characterization by FTIR and MALDI TOF and Production of Environment Friendly Wood Adhesive.” Industrial Crops and Products 40:13–20. https://doi.org/10.1016/j.indcrop.2012.02.039.
- Pizzi, A. 2006. “Recent Developments in Eco-Efficient Bio-Based Adhesives for Wood Bonding: Opportunities and Issues.” Journal of Adhesion Science and Technology 20 (8): 829–846. https://doi.org/10.1163/156856106777638635.
- Pizzi, A., R. Kueny, F. Lecoanet, B. Massetau, D. Carpentier, A. Krebs, F. Loiseau, S. Molina, and M. Ragoubi. 2009. “High resin content natural matrix–natural fibre biocomposites.” Industrial Crops and Products 30 (2): 235–240. https://doi.org/10.1016/j.indcrop.2009.03.013.
- Ramesh, M., L. RajeshKumar, and V. Bhuvaneshwari. 2021. “Bamboo Fiber Reinforced Composites.” In Bamboo Fiber Composites, edited by M. Jawaid, S. Mavinkere Rangappa, and S. Siengchin, 1–13. Singapore: Composites Science and Technology. Springer. https://doi.org/10.1007/978-981-15-8489-3_1.
- Rhazi, N. 2015. “Development of Eco-Friendly Sticky Mixes from Acacia Mollissima Bark from Morocco.” PhD Thesis, Pau.
- Ringuette, B. 2011. “Matériaux composites à base de fibres de chanvre.” PhD Thesis, Laval.
- Rohen, L. A., A. C. Neves, D. D. P. Mantovani, F. C. Maurício, J. da Silva Vieira, L. D. A. Pontes, F. M. Margem, and S. Monteiro. 2017. “Hemp Fiber Density Using the Pycnometry Technique.” In Characterization of Minerals, Metals, and Materials 2017, edited by S. Ikhmayies, 423–428. Springer. https://doi.org/10.1007/978-3-319-51382-9_46.
- Ronald Aseer, J., K. Sankaranarayanasamy, P. Jayabalan, R. Natarajan, and K. Priya Dasan. 2013. “Morphological, Physical, and Thermal Properties of Chemically Treated Banana Fiber.” Journal of Natural Fibers 10 (4): 365–380. https://doi.org/10.1080/15440478.2013.824848.
- Saad, H. 2013. “Development of Bio-Composites Based on Plant Fibers and Ecological Glues.” These de doctorat, Pau.
- Saba, N., M. Jawaid, O. Y. Alothman, M. Paridah, and A. Hassan. 2016. “Recent Advances in Epoxy Resin, Natural Fiber-Reinforced Epoxy Composites and Their Applications.” Journal of Reinforced Plastics & Composites 35 (6): 447–470. https://doi.org/10.1177/0731684415618459.
- Sango, T., A. M. C. Yona, L. Duchatel, A. Marin, M. K. Ndikontar, N. Joly, and J.-M. Lefebvre. 2018. “Step–Wise Multi–Scale Deconstruction of Banana Pseudo–Stem (Musa Acuminata) Biomass and Morpho–Mechanical Characterization of Extracted Long Fibres for Sustainable Applications.” Industrial Crops and Products 122:657–668. https://doi.org/10.1016/j.indcrop.2018.06.050.
- Santos, J., G. Antorrena, M. S. Freire, A. Pizzi, and J. Gonzalez-Alvarez. 2017. “Environmentally Friendly Wood Adhesives Based on Chestnut (Castanea Sativa) Shell Tannins.” European Journal of Wood and Wood Products 75 (1): 89–100. https://doi.org/10.1007/s00107-016-1054-x.
- Sauget, A., A. Nicollin, and A. Pizzi. 2013. “Fabrication and Mechanical Analysis of Mimosa Tannin and Commercial Flax Fibers Biocomposites.” Journal of Adhesion Science and Technology 27 (20): 2204–2218. https://doi.org/10.1080/01694243.2013.767151.
- Scarpa, F., S. Amroune, and A. Bezazi. 2015. “Analyse Statistique Et Effet des Traitements Chimique Sur leComportement Physico - Mécanique des Fibres des Bras de Grappe desPalmiers Dattier = Statistical Analysis and Effect of Chemical Treatment on the Physicomechanical Behavior of Fibres from Date-Palm Fruit Branches.” Synthèse : Revue des Sciences et de la Technologie 31:108–120. https://doi.org/10.12816/0027867.
- Sealy-Fisher, V. J., and A. Pizzi. 1992. “Increased Pine Tannins Extraction and Wood Adhesives Development by Phlobaphenes Minimization.” European Journal of Wood and Wood Products 50 (5): 212–220. https://doi.org/10.1007/BF02663290.
- Seignobos, C., and H. Tourneux. 2002. Le Nord-Cameroun à travers ses mots: dictionnaire de termes anciens et modernes: province de l’extrême-nord. KARTHALA Editions. https://scholar.google.com/scholar?hl=fr&as_sdt=0%2C5&q=Seignobos%2C+C.%2C+and+H.+Tourneux.+2002.+Le+Nord-Cameroun+%C3%A0+travers+ses+mots%3A+dictionnaire+de+termes+anciens+et+modernes%3A+province+de+l%E2%80%99extr%C3%AAme-nord.+KARTHALA+Editions.&btnG=.
- Seki, Y., A. Ç. Kıilıinç, R. Dalmıiş, S. Köktaş, and E. Çelik. 2019. “Characterization of Flax, Jute, and Sisal Fibers After Sodium Perborate Modification.” AATCC Journal of Research 6 (6): 25–31. https://doi.org/10.14504/ajr.6.6.4.
- Sikame Tagne, N. R., E. Njeugna, M. Fogue, J.-Y. Drean, A. Nzeukou, and D. Fokwa. 2014. “Study of Water Absorption in Raffia Vinifera Fibres from Bandjoun, Cameroon.” Scientific World Journal 2014.
- Singh, G., S. Jose, D. Kaur, and B. Soun. 2020. “Extraction and Characterization of Corn Leaf Fiber.” Journal of Natural Fibers 19 (5): 1581–1591. https://doi.org/10.1080/15440478.2020.1787914.
- Soppie, A. G., A. D. O. Betene, P. M. A. Noah, A. E. Njom, F. B. Ebanda, A. Ateba, A. Mewoli, D. N. Efeze, and R. Moukene. 2023. “Chemical Extraction and Its Effect on the Properties of Cordleaf Burbark (Triumphetta Cordifolia A. Rich) Fibres for the Manufacture of Textile Yarns.” Heliyon 9 (6): e17581. https://doi.org/10.1016/j.heliyon.2023.e17581.
- Stawski, D., E. Çalişkan, N. D. Yilmaz, and I. Krucińska. 2020. “Thermal and Mechanical Characteristics of Okra (Abelmoschus Esculentus) Fibers Obtained via Water-And Dew-Retting.” Applied Sciences 10 (15): 5113. https://doi.org/10.3390/app10155113.
- Subramanya, R., K. G. Satyanarayana, and B. Shetty Pilar. 2017. “Evaluation of Structural, Tensile and Thermal Properties of Banana Fibers.” Journal of Natural Fibers 14 (4): 485–497. https://doi.org/10.1080/15440478.2016.1212771.
- Thébault, M., A. Pizzi, S. Dumarçay, P. Gerardin, E. Fredon, and L. Delmotte. 2014. “Polyurethanes from Hydrolysable Tannins Obtained without Using Isocyanates.” Industrial Crops and Products 59:329–336. https://doi.org/10.1016/j.indcrop.2014.05.036.
- Trosa, A., and A. Pizzi. 2001. “A No-Aldehyde Emission Hardener for Tannin-Based Wood Adhesives for Exterior Panels.” Holz als Roh- und Werkstoff 59 (4): 266–271. https://doi.org/10.1007/s001070100200.
- Vidil, L. 2019. “Study of 2D Natural Materials - Potential for Use As Reinforcement in 307P Composite Materials.”
- Wambua, P., J. Ivens, and I. Verpoest. 2003. “Natural Fibres: Can They Replace Glass in Fibre Reinforced Plastics?” Composites Science and Technology 63 (9): 1259–1264. https://doi.org/10.1016/S0266-3538(03)00096-4.
- Wedaïna, A. G., A. Pizzi, W. Nzie, R. Danwe, N. Konaï, S. Amirou, C. Segovia, and R. Kueny. 2021. “Performance of Unidirectional Biocomposite Developed with Piptadeniastrum Africanum Tannin Resin and Urena Lobata Fibers As Reinforcement.” Journal of Renewable Materials 9:477–493. https://doi.org/10.32604/jrm.2020.012782.
- Xi, X., A. Pizzi, C. Gerardin, X. Chen, and S. Amirou. 2020. “Soy Protein Isolate-Based Polyamides As Wood Adhesives.” Wood Science and Technology 54 (1): 89–102. https://doi.org/10.1007/s00226-019-01141-9.
- Yilmaz, N. D., M. Sulak, K. Yilmaz, and F. Kalin. 2016. “Physical and Chemical Properties of Water-Retted Fibers Extracted from Different Locations in Corn Husks.” Journal of Natural Fibers 13 (4): 397–409. https://doi.org/10.1080/15440478.2015.1029201.
- Yilmaz, N., M. Sulak, K. Yılmaz, and G. M. Khan. 2017. “Effect of Chemical Treatments on Physicochemical Properties of Fibres from Banana Fruit and Bunch Stems.” Indian Journal of Fibre & Textile Research 42:111–117. https://doi.org/10.56042/ijftr.v42i1.8684.
- Youmssi, D., Y. D. Bampel, J. Njankouo, J.-B. Saha Tchinda, and M. Ndikontar. 2017. “Chemical Composition of Some Plantation Wood Species (Eucalyptus Saligna, Cupressus Lusitanica and Eucalyptus Paniculata) and Assessment of Compatibility with Plaster.” Journal of the Indian Academy of Wood Science 14 (2): 146–153. https://doi.org/10.1007/s13196-017-0200-3.
- Zhu, J., H., Abhyankar, and J. Njuguna. 2013. “Effect of Fibre Treatment on Water Absorption and Tensile Properties of Flax/Tannin Composites.” Proceedings of the ICMR.