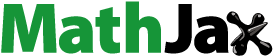
ABSTRACT
The modification method of grafting multi-walled carbon nanotubes (MWCNT, abbreviated as CNT in this paper) on the surface of flax fibers was investigated, i.e. CNT were grafted onto the surface of flax fibers by silane coupling agent under the action of ultrasonic waves to form covalent bonding. The tensile strength of CNT grafted flax fiber is 22% higher than that of untreated flax fiber monofilament. The tensile strength of FFRP composites after CNT grafting treatment increased by 14.2%; however, the tensile modulus of single fiber and composites did not show a significant increase, the interfacial shear strength of the fiber-resin is 38.3% higher than that of untreated filament. The improvement of the contact angle after grafting was investigated by observing the surface morphology, and the surface of flax filament was characterized by scanning electron microscopy and atomic force microscopy. Also the elemental changes of the flax single fiber surface before and after the treatment were analyzed using X-ray photoelectron spectroscopy. The results showed that the tensile strength of flax single fibers, its composites, and interfacial shear strength of fiber-matrix was improved after CNT grafting treatment but the increase of modulus was not obvious.
摘要
研究了在亚麻纤维表面接枝多壁碳纳米管(MWCNT,本文简称CNT)的改性方法,即在超声波作用下,用硅烷偶联剂将碳纳米管接枝到亚麻纤维表面,形成共价键. CNT接枝亚麻纤维的拉伸强度比未处理的亚麻纤维单丝高22%. CNT接枝处理后的FFRP复合材料的拉伸强度提高了14.2%,但单纤维和复合材料的抗拉模量没有显著提高,纤维树脂的界面剪切强度比未处理的长丝高38.3%. 通过观察表面形貌,研究了接枝后接触角的改善,并用扫描电子显微镜和原子力显微镜对亚麻丝表面进行了表征. 利用X射线光电子能谱分析了亚麻单纤维表面处理前后的元素变化. 结果表明,碳纳米管接枝处理后,亚麻单纤维及其复合材料的拉伸强度和纤维基体的界面剪切强度均有所提高,但模量的提高不明显.
Introduction
There has been a great increase of the application of fiber-reinforced polymer composites (FRPCs) in the past decades; the synthesis materials such as carbon, glass, and aramid fibers have been applied wildly (Xian, Guo, and Li Citation2022). However, the concern about the collection of plastic debris in the production of FRPCs, using plant-based natural fibers (eg., flax fiber) in FRP materials, has gained popularity because of their low environmental impact, simple preparation, low cost, and other advantages (Sanjay et al. Citation2019; Sharma et al. Citation2023). However, its bonding performance with resin is weak as the surface polarity manner result in ineffective load transfer between fibers through the resin; thus, the mechanical properties of flax fiber composites were compromised (Cruz and Fangueiro Citation2016).
Pretreatment can effectively improve the mechanical properties of fibers, thus the mechanical properties of composite materials will increase (Chen et al. Citation2023). Pretreatment will remove impurities from the fiber surface, change the surface morphology or chemical composition of the fibers, leading to improvement of the physical or chemical bonding properties of the fiber/resin interface (Shadhin et al. Citation2023). Currently, the commonly used treatment methods are alkali treatment, acetylation treatment, and coupling agent treatment; such methods can effectively improve the interface bond strength to a certain extent (Cui et al. Citation2023). Surface modification of natural fibers to enhance the mechanical properties of flax fiber composites has become an important direction for the current research of high performance of plant fiber composites.
Among them, multi-walled carbon nanotubes have shown extraordinary effects in plant fiber modification, which have attracted the attention of many researchers. (Anirudh et al. Citation2023) concluded that the incorporation of carbon nanotubes resulted in improved flexural properties, interlaminar shear strength, and tensile strength of carbon fiber composites. (Li et al. Citation2022) found that the fracture strength and thermal degradation temperature at 20% weight loss of OCNT/WPU composites were greatly improved over pure WPU composites. (Li et al. Citation2023) concluded that the interface between carbon fibers and matrix was enhanced after grafting MWCNTs. And the interlaminar shear strength and flexural strength of their composites were improved by 52.84% and 32.32%, respectively. (Kopsidas and Olowojoba Citation2021) proposed that the fracture energy GIC of graphene nanocomposites was increased by 182% when multi-walled carbon nanotubes were added at 1 wt %. (Mustafa and Memduh Citation2021) conducted low velocity impact tests on pure GRP and GRP composites with CNT nanomaterials added found that the damage area of the composites with CNT added was reduced.
The silane coupling agent solution can modify the surface of fiber with the help of pretreatment (Fei et al. Citation2023). Research shows that the surface of natural fiber can be functionalized by silane coupling configured by mixing anhydrous ethanol (Orue et al. Citation2016). The silane coupling agent mixed with coupling agent ethanol system undergoes hydrolysis reaction to produce active silicone hydroxyl groups, and the hydrolysis process is shown in .
The condensation reaction occurs within the silane coupling agent itself, and the condensation reaction is shown in (Xu and Yang Citation2008). P. Muruganandhan prepared three various levels of intracellular adhesiveness with silane modification; the UV aging was investigated and the fracture stresses of the composites are reduced by a maximum of 35.2% (Muruganandhan et al. Citation2023).
In order to further improve the load transfer efficiency at the fiber/resin interface, this paper proposes a multi-walled carbon nanotube grafting treatment on the surface of flax fibers to improve the mechanical properties of flax fiber composites and extend the application range of plant fiber composites. The mechanical properties of single fiber and its composites will be studied and micro-debonding test will be performed. The interface properties of flax filament and resin will be tested in contact angle, and the morphology and elemental analysis of treated and untreated fiber will be performed with SEM, AFM, and XPS. Based on current research, the possibility of modified natural fiber as a substitute of basalt or glass fiber in reinforcement and retrofit of existing building will be discussed. The composite production is less carbon emission, and the cost of flax and silane agent is moderate; they are potential for both environmental and economic benefits of the application of flax fiber in FRP.
Materials and methods
Materials
Flax fiber
The flax fiber bundle used in this paper is a kind of twisted wet-spun yarn produced by Harbin Linen Textile Co., Ltd. in China, with a bulk density of 1.6 g/cm3 and an average linear density of 0.0667 g/m. The spinning process is ring spinning.
Multi-walled carbon nanotubes
In this paper, NMC20-OH hydroxylated multi-walled carbon nanotubes produced by Beijing Boyu Hi-Tech New Material Technology Co., Ltd. in China were selected for grafting studies on the surface of flax filament, and the main technical parameters of multi-walled carbon nanotubes are listed in .
Table 1. Technical parameters of hydroxylated MWCNTs.
Silane coupling agent
The silane coupling agent used in this paper is γ-aminopropyltriethoxysilane (APS, KH-550), which is purchased from Nanjing Tengong Organosilicon Materials Co. The technical parameters of SCA is listed in .
Table 2. Technical parameters of SCA KH-550.
Epoxy resin, curing agent, and accelerator
In this paper, NPEF-170 bisphenol F diglycidyl ether epoxy resin produced by Nanya Plastics Industry Co., Ltd. of Taiwan, China, and Methyl Hexahydrophthalic Anhydride (MeHHPA) produced by Jiaxing Qingyang Chemical Co., Ltd. of China as curing agent. The accelerator used in this paper is 2,4,6-tris (dimethylaminomethyl) phenol (DMP-30) produced by China Jiaxing Qingyang Chemical Co., Ltd. with a relative molecular mass of 265.4 and a density of 0.972 g/cm3 ~ 0.978 g/cm3, with a light yellow viscous liquid appearance and a configuration ratio of 2.0% of the epoxy resin mass.
Preparation of carbon nanotubes grafted on the surface of flax fiber
Silane coupling agent KH-550 and distilled water are in the ratio of 40:4:1 by mass. The 0.5 wt.% multi-walled carbon nanotubes were added into the silane coupling agent solution and stirred for 30 min using a low-speed gear under the action of ultrasonic waves, and the silicon hydroxyl groups were chemically bonded with a large number of active hydroxyl groups on the surface of carbon nanotube molecules to produce carbon nanotube suspensions. The specimen preparation process for grafting carbon nanotubes on the surface of flax fibers was as follows:
Cutting the aluminum sheet as shown in for fixing the flax filament;
From the flax fiber yarn to extract the appropriate length of flax single fiber; the flax single fiber ends straightened with conductive adhesive on the aluminum sheet to be completely cured adhesive;
The reaction was carried out in a magnetic heating stirrer with two clamps on the top of the pasted flax filament aluminum sheet, which was immersed in the configured CNT suspension, as shown in .
The treated flax filament specimens will be left for 11 h to dry naturally, you can complete the production of CNT graft specimens.
Preparation of specimen
Flax filament tensile specimen production
In this paper, the test length of flax single fiber is 20 mm, the CNT grafting treatment of flax single fiber filaments glued to the 50 × 20 mm2 paper with a 20 × 10 mm2 rectangular opening in middle, flax filament placed along the long side of the paper frame, the part through the opening of the fiber as a test area. After determining the location of the fiber, the filament is fixed on the paper sheet with a prefabricated resin. After the specimen is prepared, it is maintained at room temperature for 12 h, and the test is started after it is cured.
Preparation of flax fiber composite
The unidirectional composites were prepared using a 4FW500 × 2000 winding machine produced by Harbin FRP Research Institute. The winding rate was 0.15 m/s and the winding spacing of fiber bundles was 0.4 mm. The composites were prepared by unidirectional winding with 12 layers and the fiber content was about 33%. The dimensions of winding plate were 15 × 250 mm2, and the winding angle was 89.6º. The thickness of FFRP will be measured and used to calculate the tensile properties, and the average thickness was 2.6 (±0.1) mm. The winding process is shown in .
Figure 4. Schematic diagram of unidirectional flax fiber composite material prepared by winding method.
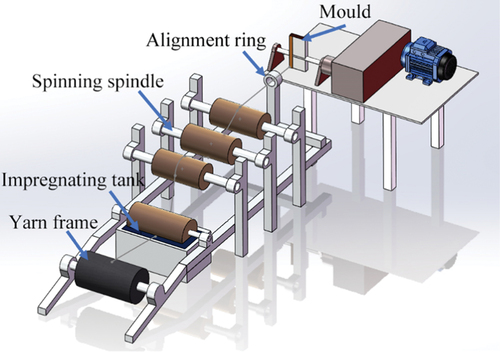
The FFRP sheets prepared by winding were cured with the mold at 120°C for 60 min and then at 150°C for 60 min, the demolded specimens are shown in . After demolding, the specimens were further cut to the proper size for further tests.
Before preparing a continuous unidirectional flax fiber composite (Flax Fiber Reinforced Polymer, FFRP), the nominal thickness of the flax fiber is first confirmed to facilitate the analysis of the properties of the composite. The nominal thickness is calculated as follows: the ratio of mass per unit area to the bulk density. The mass per unit area is obtained by calculating the linear density and winding spacing. The fiber volume content is calculated using Equation 1 (Guo et al. Citation2022).
Where
tf —— The total nominal thickness of the fibers;
tfrp —— The thickness of the material is measured as a whole.
After calculation, the nominal thickness of flax fiber is 0.104 mm. The tensile strength and modulus of flax fiber composites in this paper are based on the nominal thickness, and the analysis part will not be detailed.
Test methods
Single fiber tensile test
The tensile test of flax fiber filaments was performed by the single fiber strength tester, model JQ03A, produced by Shanghai Zhongchen Digital Technology Equipment Co. According to ASTMD3379–75 standard, before and after the treatment of flax single fiber tensile test spacing of 20 mm, stretching rate of 0.00125 mm/s, the distance between the chucks is 40 mm. The diameter of each fiber filament was measured and recorded before the test started, then the specimen was clamped and tested, the load-displacement curve was recorded. Due to the discrete properties of fiber filaments, each parameter was calculated by 25 filaments, the formula for calculating the tensile strength of monofilaments is shown in Equation 2 (Harzallah, Benzina, and Drean Citation2010):
Where
σs —— Tensile strength of flax fiber monofilaments (MPa);
Fs —— Tensile strength value at breakage of fiber monofilament (N);
d —— The diameter of flax fiber monofilament (mm).
Micro debonding test
In this paper, micro-debonding method was applied to determine the interfacial shear strength of flax fiber monofilaments and epoxy resin, based on which the interfacial bonding properties of flax fiber surface-grafted carbon nanotubes and epoxy resin were investigated. Firstly, the diameter of each flax fiber was measured, then the ends of filament were fixed on a concave metal sheet similar as the treatment. Then, resin matrix is applied to the surface of the fixed monofilament, and resin balls of various sizes are formed under surface tension. The microdroplet should be selected between 50 µm and 200 µm to apply the micro-debonding test.
The micro-debonding test utilizes the FA-620 micro-debonding tester manufactured by Toei Corporation, Japan, with a maximum load of 500 mN and a chucking speed of 0.06 mm/min. The fiber/resin is debonded by moving the fiber under a microscope using a clamping device to clamp the droplet, and the schematic diagram of the micro-debonding test is shown in .
The interfacial shear strength between flax monofilament fiber and resin was calculated according to Equation 3 (Samper Madrigal et al. Citation2015); the average value of 20 valid data from treated and untreated fibers was compared.
where
τs—— Tensile strength of flax fiber monofilaments (MPa);
Fmax—— The value of the tensile strength of the fiber monofilament at breakage (N);
d —— Diameter of flax fiber monofilament (mm);
l —— Length of fiber encapsulation in the resin (mm).
Tensile properties of fiber composites
In this paper, the American Society for Testing and Materials tensile property test standard for resin-based composites (ASTMD3039/D3039M) was used, and the prepared tensile test specimens of flax fiber composites were cut into 180 mm × 15 mm. The tensile properties were tested with an electronic universal material testing machine (WDW-100E, Jinan Times Testing and Metallurgy Testing Machine Co., Ltd.) with a loading speed of 2 mm/min, and at least five specimens were tested for each same specimen.
Contact angle
The interfacial performance between the fiber and the resin matrix is measured by the contact angle between the resin and fiber. A certain size of epoxy resin was wrapped on the flax filament, and then the droplets were measured by microscope after on the surface of the filament. The droplet and surface of the single fiber are shown in .
According to the computational model proposed by Carroll, the contact angle between the thickness of the droplet and the sum of the single fiber radius T, the resin sphere length L and the single fiber radius R and the contact angle θ were satisfied by Equation 4, and then the contact angle between the liquid and the flax single fiber was calculated by the method proposed by (Carroll Citation1976).
where
l —— The ratio of the droplet length L to the single fiber radius R;
a —— As a function of t and θ, the expression;
t —— The ratio of the sum of droplet thickness and monofilament radius, T, to the monofilament radius, R;
F(ϕ,k) —— The Legendre form of the first type of incomplete elliptic integral with the expression:
E(ϕ,k) —— The Legendre form of the second type of incomplete elliptic integral with the expression:
where the parameters ϕ and k are functions with respect to a and θ. The expressions are as follows:
Surface morphology characterization of flax single fibers
Scanning Electron Microscope (SEM) is a field emission environment SEM (Quanta200FEG) manufactured by FEG, USA, with acceleration voltage 200–30 kV, high vacuum mode, and electron image resolution of 1.2 × 3.0 nm. The surface of the specimen is sprayed with gold (Ag). The gold spraying time was 10 min.
In this paper, a Dimension Icon Atomic Force Microscope (AFM) from Bruker, Germany, was used to observe the surface morphology of the fibers with a scanning size of 1 m, a scanning speed of 10 Hz, and a scanning mode set to Tapping Mode.
XPS test analysis
In this paper, X-ray photoelectron spectroscopy (XPS) was used to analyze the surface functional groups and elemental changes of flax fiber bundles before and after grafting treatment. A K-Alpha X-ray photoelectron spectrometer from Thermofisher Scientific Company was used with an aluminum target X-ray source (Al Kα, h = 1000 eV) with a monochromator, an X-ray beam emission current of 6 mA, a power of 200W, a sensitivity of 350kcps, a passage energy PE = 50 eV, a scan range of 0- 1350 eV, and scan step size 0.1 eV.
Experimental results and analysis
Analysis of the tensile properties of single fibers
The typical tensile stress-strain curve of untreated flax filament is shown in . The nonlinear behavior is consistent with the conclusion of (Chokshi et al. Citation2020) who defined “inflection points” in the tensile stress-strain curve of natural fibers.
In this paper, the two-stage characteristics of the tensile stress-strain curves of flax filament were used with the same results as those of existing studies on plant fibers (Charleta et al. Citation2009; Placet Citation2009). In the initial stage of stretching, the stress-strain relationship is linear and this section is the elastic stage. In the elastic phase, the applied tensile stress is low and the crystalline zone macromolecular polymeric chains are first displaced under tension, while the non-crystalline zone does not cause displacement due to the low force provided (Zhu and Goda Citation2008). The greater the tensile modulus of the fiber, the better the stiffness of the fiber, the size of the tensile modulus of the fiber and the orientation of the molecular chain and the composition of the macromolecule.
Flax fiber stress-strain curve in the second stage of the modulus decreased significantly the fiber is about to break when the molecular chain segments in the fiber irreversible displacement, while the fiber chain segments between the misalignment and slip. Meredith defines the inflection point between elastic and plastic deformation of the plant fiber stretching curve: the tangent line of the curve at the inflection point is parallel to the line connecting the starting point and the limit point, and the fiber is mainly stressed by the crystals in the crystalline region before the inflection point, and mainly by the polymer chains in the non-crystalline region after the inflection point. Before the inflection point, the fiber is mainly stressed by the crystals in the crystalline region, and after the inflection point, the polymer chains are mainly stressed in the non-crystalline region (Bunsell Citation2009). The initial tensile modulus, i.e. the elastic modulus, is determined by the properties of the macromolecules in the fiber. In the elastic phase, the macromolecular chains in the fiber do not change under stress. In this paper, the change in modulus during fiber stretching is considered to be related to the change in the number of macromolecular chains contained in the fiber. The comparison of tensile stress-strain curves of flax single fibers before and after CNT grafting treatment is shown in .
The filament tensile modulus of flax filament treated with carbon nanotube suspension did not show obvious changes like untreated flax filament, the reason may be due to the modulus and the composition of the material molecular orientation and macromolecular weight. Considering the discrete mechanical properties of flax fiber filaments, the enhancement effect of carbon nanotubes on the tensile modulus of filaments was small, mainly because the grafting reaction of carbon nanotubes mainly occurred on the surface of flax fiber, and the structural changes of β-glucose monomer inside the fiber did not occur, indicating that the molecular orientation and the number of molecular chains in the fiber did not change significantly before and after carbon nanotube grafting treatment.
The tensile strength and modulus of untreated and carbon nanotube grafted flax fibers are shown in . The tensile strength of flax fiber filaments was significantly improved after the carbon nanotube grafting on the surface, while improvement of modulus is slight.
Table 3. Tensile strength and modulus of modified single flax fiber.
CNT graft modified flax fiber than the untreated flax fiber filament strength increased by 22%, achieved a significant strengthening effect, the reason is that the treatment process cleaned the impurities and gums, thus the ration of cellulose with better mechanical properties increased, so the tensile strength can be enhanced. Compared with untreated flax filament, the tensile modulus of treated filament increased by only 1.09%, which is because the modulus of the fiber is mainly determined by the molecular chain of macromolecules and molecular composition, while the current modification process cannot cause the reconstruction of the internal elements of the fiber, thus the modulus is not significantly improved.
Analysis of microdebonding results
In this paper, the micro-debonding shear strength of untreated filaments with that of carbon nanotube grafted filaments is compared. The results of the comparison tests are shown in .
Table 4. Micro debonding test results of plant fiber and epoxy resin system.
The interfacial shear strength of carbon nanotubes grafted flax fibers and epoxy resin was improved significantly, presumably due to the grafting of carbon nanotubes on the surface of flax fibers through covalent bonds. On the one hand, carbon nanotube grafting can improve the roughness of plant fiber surface, enhance the mechanical occlusal force, and strengthen the effective bonding area between resin and fiber; on the other hand, in the process of interfacial debonding, carbon nanotubes with excellent performance can effectively retard the occurrence of stress concentration by changing the direction of microcrack expansion in the interfacial layer, thus improving the interfacial shear strength. The specific surface area of carbon nanotubes is 50 ~ 1300 m2/g, and grafting on the surface of flax fibers can greatly increase the specific surface area of fibers, that is, the nano-scale reinforcing phase is created on the surface of fibers, which can effectively improve the contact area between the reinforcing phase and resin matrix and increase the strength of their effective interfacial bonding.
The specimens after the micro-debonding test were observed by SEM. As shown in , after debonding of the untreated flax filament-epoxy resin system, the fiber surface was relatively smooth, indicating that the bonding performance of the untreated linen fiber and resin interface was poor. As shown in , after the carbon nanotube grafting treatment, the presence of resin adhesion on the fiber surface with the phenomenon of fiber surface peeling was observed. This phenomenon of fiber surface peeling is related to the multi-scale structure of plant fibers, and the indentation modulus of other natural fiber cross-section was tested, and the test results showed that the indentation modulus of the primary wall of fiber cells is significantly lower than the modulus of the secondary wall due to the different growth patterns of cell walls during the growth of plant fibers (Arnould et al. Citation2017), and the outer cell wall mechanical properties of plant fibers are weaker.
In the micro-debonding test, the modified fiber/resin interface peeled the primary cell wall of the plant fiber surface from the fiber under the effect of stronger interfacial shear because of the increased bond strength of the modified fiber/resin interface. In addition to the damage pattern where peeling occurred at the fiber surface, a small amount of resin also adhered to the fiber surface, which indicated that the bond strength at the interface was also higher than the shear strength inside the resin. By comparing the results of SEM analysis, it was concluded that the damage behavior in the flax fiber/resin system after CNT grafting treatment could be represented by the following two main modes, as shown in .
The modified linen fiber resin interface layer is no longer the weak link inside the composite, and the damage of the composite occurs inside the resin matrix or the surface of fiber.
Analysis of tensile properties of unidirectional flax fiber composites
It has been found that the tensile stress-strain curves of synthetic fiber-reinforced polymer composites are linear, while the stress-strain curves of flax or other natural fiber composites are nonlinear. Therefore, this paper studies the tensile stress-strain curves of flax fiber composite sheets, and the test results of their flax fiber composites are shown in .
As seen in , the tensile stress-strain curves of the flax fiber composites exhibit a two-stage characteristic, which is in agreement with the available results (Coroller et al. Citation2013; Madsen, Hoffmeyer, and Lilholt Citation2007). In this paper, the elastic modulus of FFRP is calculated with reference to ASTM D3039, taking the tangential modulus for material strains between 0 and 0.01 (ASTM. Citation2017). It is worth noting that the elastic modulus of the flax fiber composites, during the stretching process, the elastic modulus E2 decreases by more than 50% compared to the initial elastic modulus E1. The reason for this may be due to the fact that during the stretching process, the flax fiber bundles are pulled and untwisted resulting in a weaker bond between the fibers and the resin and thus a substantial decrease in the modulus.
The following analysis will compare the tensile properties of untreated, CNT-grafted flax fiber composite specimens, the test results are shown in .
Table 5. The tensile strength and modulus of CNT-grafted modified flax fiber composite material.
The tensile strength was increased by 14.2%, which was due to the improved properties of the single fibers, followed by the enhanced mechanical occlusal connection between the fiber and resin interfaces by CNT, and the improved interfacial properties of the composites. The tensile modulus was increased by 7.6%, which is consistent with the experimental findings of (Wang, Xian, and Li Citation2015). The use of multi-walled carbon nanotubes modified flax filament achieved a significant effect on improving the tensile strength of flax fiber composites, but the enhancement effect on tensile modulus was relatively small, and it was inferred that the tensile modulus enhancement effect of multi-walled carbon nanotubes modified flax fibers on composites was not obvious. There is an unexpected result: the standard deviation of tensile properties was decreased, that might be because the weak fibers have a premature failure during treatment.
Surface characterization and interfacial property analysis
Contact angle analysis
Through fiber contact angle analysis of the flax fiber-epoxy resin system, it can be observed that the contact angle between the untreated flax fiber and the resin matrix is 24.45°, as shown in . After CNT grafting treatment, the contact angle between the flax fiber and the resin is 19.46°, as shown in .
The contact angle of flax fiber and epoxy resin matrix becomes smaller after CNT grafting treatment, indicating the treatment can improve the wettability of flax fiber and epoxy resin interface. CNT grafting treatment can form a layer of carbon nanotube film on the surface of flax fiber, which increases the roughness and surface energy of flax fiber surface and improves the interfacial interaction between flax fiber and epoxy resin. This improvement reduces the surface tension of the material, making it easier to contact with liquids and helps to enhance the wetting properties of liquids on the material surface. Thus, the CNT grafting treatment improves the wetting properties of the flax fiber and epoxy resin interface.
Surface morphology analysis of flax single fiber
In order to verify the effect of carbon nanotube grafting on the surface morphology of the fiber, SEM and AFM observations were performed on untreated and CNT-grafted flax single fibers, respectively. SEM analysis tests were able to characterize the dispersion of CNT on the surface of flax fibers, and AFM analysis tests were able to characterize the binding and dispersion of CNT on the surface of flax fibers. As shown in , the length of one side of AFM picture was one nanometer.
is the surface of untreated flax fiber, it is flat, smooth, and attached to a small amount of low-molecular material; as shown in , CNT-grafting treatment of flax fiber, there were obvious grooves and increased roughness on the surface of the fiber after CNT grafting, that is CNT uniformly dispersed on the surface of the fiber, and the formation of a net-like film on the surface of the linen single fiber, which is conducive to improving the bonding properties of the fiber and resin surface.
In order to further characterize the effect of carbon nanotubes acting on the surface of flax fibers, the morphology of the flax single fiber surface was observed and analyzed by AFM. It can be clearly be observed that shows a relatively smooth and flat surface of untreated flax single fiber, which is not conducive to the combination with the resin matrix; while in , the surface of flax fiber after CNT-grafting contains many grooves, and the roughness of the fiber surface is significantly increased. These grooves will provide an effective mechanical connection of the interface between the fiber and the matrix and therefore can effectively improve the shear strength of the interface.
Analysis of the surface composition of flax fiber
To investigate the effect of CNT-grafting treatment on the surface composition of flax fibers, this section analyzes the surface elemental composition of flax fibers using XPS. It has been shown that the oxygen to carbon ratio (O/C) of different components in the same fiber is different, for example, the O/C of cellulose is about 0.83, hemicellulose and pectin are also about 0.83, and the O/C of lignin is about 0.35, and the O/C of flax fiber is reduced after carbon nanotube grafting treatment, which indicates that carbon nanotube grafting has a positive effect on the fiber surface.
The O/C ratios in this chapter are taken as the corresponding ratios of various elements after the XPS test and then compared. The test curves are shown in , and the results of the elemental ratios are shown in .
Table 6. The ratio of oxygen to carbon on the surface of flax fiber after CNT grafting.
In the CNT-grafting treatment before the flax single fiber surface oxygen to carbon ratio is high, which is due to the flax fiber surface, there would have been a certain number of functional groups, such as hydroxyl groups, ester groups, carbon groups, etc., these groups react with oxygen molecules in the air, the formation of oxides, resulting in a high surface oxygen content. The CNT-grafting treatment will introduce carbon elements, which will replace part of the oxygen atoms, thus leading to a decrease in the ratio of oxygen to carbon on the surface, indicating that after the treatment, CNT formed an effective coverage on the surface of the fiber.
Conclusion
In this paper, the effects of carbon nanotube grafting treatment on the tensile properties, surface morphology, and elemental composition of flax fibers were studied, the effects of surface carbon nanotube grafting on the interfacial bonding of flax fiber composites and the mechanical properties of the composites were characterized by microscopic analysis and mechanical tests, and the following conclusions were obtained:
The modulus of the single flax fiber is not significantly improved after CNT modification process, as it is mainly determined by the molecular chain of macromolecules and molecular composition; the treatment is not enough to cause the reconstruction of the internal elements of the fiber.
The tensile strength of the flax fiber composite was improved, which is due to the improved tensile strength of single fibers and the improved interfacial properties. The modulus of the composites was mainly related to the modulus of the single fibers, which did not change after treatment.
After CNT-grafting treatment of flax fiber and micro-debonding damage morphology indicated that the modified flax fiber resin interfacial properties were significantly improved. The contact angle between flax fiber and epoxy resin was reduced, indicating that CNT grafting improved the interfacial infiltration properties.
The surface morphological characterization of flax single fiber shows that the surface of the fiber formed a net-like film after treatment, which helped to improve the bonding performance of the fiber and resin, so CNT-grafting could effectively improve the interfacial strength of the fiber matrix.
The elemental composition of the fiber surface was found that the oxygen-to-carbon ratio (O/C) decreased after CNT-grafting treatment compared with untreated fibers, indicating that CNT introduced carbon to form an effective coverage on the surface.
Hightlights
Flax fiber was modified with grafted multi-walled carbon nanotube (MWCNT).
The tensile strength was improved but the modulus barely changed.
Test results show the interfacial properties of fiber and resin were improved effectively.
Disclosure statement
No potential conflict of interest was reported by the author(s).
Additional information
Funding
References
- Anirudh, S., K. A. Prabha, S. B. Murthy, and B. C. Rao. 2023. “Enhancement of Mechanical Properties of Matrix by Functionalized Multi-Wall Carbon Nanotubes (MWCNT) in Composite Material.” Materials Today: Proceedings 92 (2): 1186–18. https://doi.org/10.1016/j.matpr.2023.05.308.
- Arnould, O., D. Siniscalco, A. Bourmaud, A. L. Duigou, and C. Baley. 2017. “Better Insight into the Nano-Mechanical Properties of Flax Fibre Cell Walls.” Industrial Crops and Products 97:224–228. https://doi.org/10.1016/j.indcrop.2016.12.020.
- ASTM. 2017. D3039/D3039M-17. 105–116.
- Bunsell, A. R. 2009. Handbook of Tensile Properties of Textile and Technical Fibres. Cambridge: Elsevier.
- Carroll, B. J. 1976. “The Accurate Measurement of Contact Angle, Phase Contact Areas, Drop Volume, and Laplace Excess Pressure in Drop-On-Fiber Systems.” Journal of Colloid and Interface Science 57 (3): 488–495. https://doi.org/10.1016/0021-9797(76)90227-7.
- Charleta, K., S. Evea, J. P. Jernota, M. Gominaa, and J. Breard. 2009. “Tensile Deformation of a Flax Fiber.” Procedia Engineering 1 (1): 233–236. https://doi.org/10.1016/j.proeng.2009.06.055.
- Chen, Z., K. Du, H. Yin, D. Zhang, J. Gao, W. Song, and S. Zhang. 2023. “Surface Modification of Bamboo Fiber with Dopamine Associated by Laccase for Poly(3-Hydroxylbutyrate) Biocomposites.” Journal of Cleaner Production 389 (20): 135996. https://doi.org/10.1016/j.jclepro.2023.135996.
- Chokshi, S., V. Parmar, P. Gohil, and V. Chaudhary. 2020. “Chemical Composition and Mechanical Properties of Natural Fibers.” Journal of Natural Fibers 19 (10): 3942–3953. https://doi.org/10.1080/15440478.2020.1848738.
- Coroller, G., A. Lefeuvre, A. L. Duigou, A. Bourmaud, G. Ausias, T. Gaudry, and C. Baley. 2013. “Effect of Flax Fibres Individualisation on Tensile Failure of Flax/Epoxy Unidirectional Composite.” Composites Part A, Applied Science and Manufacturing 51:62–70. https://doi.org/10.1016/j.compositesa.2013.03.018.
- Cruz, J., and R. Fangueiro. 2016. “Surface Modification of Natural Fibers: A Review.” Procedia Engineering 155:285–288. https://doi.org/10.1016/j.proeng.2016.08.030.
- Cui, H., C. Wang, X. Liu, J. Yuan, and Y. Liu. 2023. “Dynamic Simulation of Fluid-Structure Interactions Between Leaves and Airflow During Air-Assisted Spraying: A Case Study of Cotton.” Computers and Electronics in Agriculture 209:107817. https://doi.org/10.1016/j.compag.2023.107817.
- Fei, B., D. Wang, N. AlMasoud, H. Yang, J. Yang, T. S. Alomar, B. Puangsin d, et al. 2023. “Bamboo Fiber Strengthened Poly(lactic Acid) Composites with Enhanced Interfacial Compatibility Through a Multi-Layered Coating of Synergistic Treatment Strategy.” International Journal of Biological Macromolecules 249 (30): 126018. https://doi.org/10.1016/j.ijbiomac.2023.126018.
- Guo, R., G. Xian, C. Li, X. Hunag, and M. Xin. 2022. “Effect of Fiber Hybridization Types on the Mechanical Properties of Carbon/Glass Fiber Reinforced Polymer Composite Rod.” Mechanics of Advanced Materials and Structures 29 (27): 6288–6300. https://doi.org/10.1080/15376494.2021.1974620.
- Harzallah, O., H. Benzina, and J.-Y. Drean. 2010. “Physical and Mechanical Properties of Cotton Fibers: Single-Fiber Failure.” Textile Research Journal 80 (11): 1093–1102. https://doi.org/10.1177/0040517509352525.
- Kopsidas, S., and G. B. Olowojoba. 2021. “Multifunctional Epoxy Composites Modified with a Graphene Nanoplatelet/Carbon Nanotube Hybrid.” Journal of Applied Polymer Science 138 (35): 50890. https://doi.org/10.1002/app.50890.
- Li, C., Dong, Y., Yuan, X., Qiu, Z., Zhang, Y., Yan, S., Gao, X., and Zhu, B. “Two-step method to realize continuous multi-wall carbon nanotube grafted on the fibers to improve the interface of carbon fibers/epoxy resin composites based on the Diels-Alder reaction.” Carbon 212: 118131. https://doi.org/10.1016/j.carbon.2023.118131
- Li, Y., Y. Shang, J. He, M. Li, and M. Yang. 2022. “Low-Loading Oxidized Multi-Walled Carbon Nanotube Grafted Waterborne Polyurethane Composites with Ultrahigh Mechanical Properties Improvement.” Diamond and Related Materials 130:109427. https://doi.org/10.1016/j.diamond.2022.109427.
- Madsen, B., P. Hoffmeyer, and H. Lilholt. 2007. “Hemp Yarn Reinforced Composites – II. Tensile Properties. Composites Part a.” Composites Part A, Applied Science and Manufacturing 38 (10): 2204–2215. https://doi.org/10.1016/j.compositesa.2007.06.002.
- Muruganandhan, P., S. Jothilakshmi, R. Vivek, S. Nanthakumar, S. Sakthi, S. Mayakannan, and R. Girimurugan. 2023. “Investigation on Silane Modification and Interfacial UV Aging of Flax Fibre Reinforced with Polystyrene Composite.” Materialstoday Proceedings. https://doi.org/10.1016/j.matpr.2023.03.272.
- Mustafa, T., and K. Memduh. 2021. “Low-Velocity Impact Response of Pre-Stressed Glass Fiber/Nanotube Filled Epoxy Composite Tubes.” Journal of Composite Materials 55 (7): 915–926. https://doi.org/10.1177/0021998320961552.
- Orue, A., A. Jauregi, U. Unsuain, J. Labidi, A. Eceiza, and A. Arbelaiz. 2016. “The Effect of Alkaline and Silane Treatments on Mechanical Properties and Breakage of Sisal Fibers and Poly(lactic Acid)/Sisal Fiber Composites.” Composites Part A, Applied Science and Manufacturing 84:186–195. https://doi.org/10.1016/j.compositesa.2016.01.021.
- Placet, V. 2009. “Characterization of the Thermo-Mechanical Behaviour of Hemp Fibres Intended for the Manufacturing of High Performance Composites. Composites Part a.” Composites Part A, Applied Science and Manufacturing 40 (8): 1111–1118. https://doi.org/10.1016/j.compositesa.2009.04.031.
- Samper Madrigal, M., R. Petrucci, L. Sánchez Nacher, R. Balart Gimeno, and J. Kenny. 2015. “Effect of Silane Coupling Agents on Basalt Fiber-Epoxidized Vegetable Oil Matrix Composite Materials Analyzed by the Single Fiber Fragmentation Technique.” Polymer Composites 36 (7): 1205–1212. https://doi.org/10.1002/pc.23023.
- Sanjay, M. R., S. Siengchin, J. Parameswaranpillai, M. Jawai, C. Iulian Pruncu, and A. Khan. 2019. “A Comprehensive Review of Techniques for Natural Fibers As Reinforcement in Composites: Preparation, Processing and Characterization.” Carbohydrate Polymers 207:108–201. https://doi.org/10.1016/j.carbpol.2018.11.083.
- Shadhin, M., M. Rahman, R. Jayaraman, Y. Chen, D. Mann, and W. Zhong. 2023. “Natural Biomass & Waste Biomass Fibers – Structures, Environmental Footprints, Sustainability, Degumming Methods, & Surface Modifications.” Industrial Crops and Products 204 (15): 117252. https://doi.org/10.1016/j.indcrop.2023.117252.
- Sharma, H., A. Kumar, S. Rana, N. Gopal Sahoo, M. Jamil, R. Kumar, S. Sharma, et al. 2023. “Critical Review on Advancements on the Fiber Reinforced Composites: Role of Fiber/Matrix Modification on the Performance of the Fibrous.” Journal of Materials Research and Technology 26:2975–3002. https://doi.org/10.1016/j.jmrt.2023.08.036.
- Wang, H., G. Xian, and H. Li. 2015. “Grafting of Nano-TiO2 Onto Flax Fibers and the Enhancement of the Mechanical Properties of the Flax Fiber and Flax Fiber/Epoxy Composite.” Composites Part A, Applied Science and Manufacturing 76:172–180. https://doi.org/10.1016/j.compositesa.2015.05.027.
- Xian, G., R. Guo, and C. Li. 2022. “Combined Effects of Sustained Bending Loading, Water Immersion and Fiber Hybrid Mode on the Mechanical Properties of Carbon/Glass Fiber Reinforced Polymer Composite.” Composite Structures 281 (1): 115060. https://doi.org/10.1016/j.compstruct.2021.115060.
- Xu, L., and M. Yang. 2008. “In situ Compatibilization of Polypropylene and Polystyrene-Grafted Nano-Sized TiO2 in the Presence of Friedel–Crafts Catalyst.” Materials Letters 62 (17–18): 2607–2610. https://doi.org/10.1016/j.matlet.2007.12.064.
- Zhu, L., and K. Goda. 2008. “Strengthening and Stiffening of Ramie Yarns by Applying Cyclic Load Treatment.” Journal of Applied Polymer Science 109 (2): 889–896. https://doi.org/10.1002/app.28146.