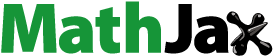
Abstract
This study examined the mechanical and chemical properties of an experimental provisional restoration containing Sr-bioactive glass nanoparticles (Sr-BGNPs) compared to commercial provisional materials. The experimental material (TempS10) contained dimethacrylate monomers with added 10 wt% Sr-BGNPs. The degree of monomer conversion (DC) of self-curing (n = 5), biaxial flexural strength (BFS)/modulus (BFM) (n = 5), and color changes (ΔE*00) of materials in red wine (n = 5) were determined. Additionally, ion release (Ca, P, and Sr) in water at 2 weeks was examined (n = 3). The commercial materials tested included polymethyl methacrylate-based provisional material (Unifast) and bis-acrylic materials (Protemp4 and Cooltemp). TempS10 exhibited a comparable degree of monomer conversion (49%) to that of Protemp4 (60%) and Cooltemp (54%) (p > 0.05). The DC of Unifast (81%) was significantly higher than that of other materials (p < 0.05). TempS10 showed a BFS (126 MPa) similar to Cooltemp (102 MPa) and Unifast (123 MPa), but lower than Protemp4 (194 MPa). The immersion time for 2 weeks exhibited no detrimental effect on the strength and modulus of all materials. The highest ΔE*00 at 24 h and 2 weeks was observed with TempS10, followed by Cooltemp, Unifast, and Protemp4. Only TempS10 showed a detectable amount of Ca (0.69 ppm), P (0.12 ppm), and Sr (3.01 ppm). The experimental provisional resin restoration containing Sr-BGNPs demonstrated polymerization and strength comparable to those of bis-acryl provisional restorations but with the added benefit of ion-releasing properties. However, the experimental material demonstrated unsatisfactory color stability.
Introduction
The temporization step with provisional dental restorations is the crucial step in fixed prosthodontic treatments. The most commonly used provisional materials can be divided according to the main resin monomers, such as auto-polymerizing polymethylmethacrylate (PMMA) and bis-acryl composites, such as bisphenol A-glycidyl dimethacrylate (Bis-GMA) and urethane di-methacrylate (UDMA) based materials [Citation1]. The major concern of PMMA is the risk of toxic monomer release and its exothermic reaction during the polymerization [Citation2–4], which could potentially induce inflammation of the pulp-dentin complex. Currently, Bis-GMA-based provisional materials are widely used due to their ease of placement and high mechanical strength. The concern with Bis-GMA based provisional materials is the risk of contamination with Bisphenol A (BPA), which could cause health concerns related to estrogenic effects [Citation5]. UDMA is commonly used as an alternative base monomer to Bis-GMA. Another potential benefit of using UDMA as the base monomer could be the increase in the degree of monomer conversion for the material [Citation6].
The placement of provisional restorations may require a long temporization period, such as for implant placement. The provisional materials that exhibited an unsatisfactory appearance upon exposure to colorants agents may also reduce the patient’s comfort [Citation7]. The roughness and low marginal seal of provisional restoration, compared to permanent restorations, may additionally promote bacterial adhesion [Citation8]. This may subsequently compromise the treatment outcome. For patients at high risk for developing periodontal diseases or secondary caries, provisional materials with remineralizing or antibacterial actions may be preferred.
Various ion-releasing fillers that could potentially enhance antibacterial and remineralizing actions for resin-based dental composites have been reported. Bioactive glass is the commonly used additives to enable both remineralizing and antibacterial actions [Citation9, Citation10]. The previous studies showed that the addition of Sr-bioactive glass promoted the release of essential elements for mineralizing actions such as Ca, P, and Sr [Citation11–13]. A study also reported that Sr inhibited the growth and reproduction, synthesis of cell walls, cell metabolism, and chromosomal replication of bacteria [Citation14]. It was demonstrated that the composites containing bioactive glass enhanced the precipitation of calcium phosphates to a demineralized dentin [Citation15]. Furthermore, the release of ions or particles could inhibit the growth of pathogenic oral microorganisms without causing serious cytotoxic effects [Citation16–18]. However, the addition of the bioactive glass at high concentration demonstrated the negative effects on the mechanical and physical properties of the materials [Citation19].
This study prepared experimental UDMA-based provisional dental restoration containing Sr-bioactive glass nanoparticles (Sr-BGNPs). The objective of this study was to compare the mechanical/physical properties of the experimental material with commercial provisional materials. The null hypothesis was that the degree of monomer conversion, biaxial flexural strength/modulus, color stability, and ion release of the experimental material should not differ significantly from the commercial materials.
Materials and methods
Preparation of experimental provisional restoration (TempS10)
Sr-bioactive glass nanoparticles (Sr-BGNPs) were produced by the sol-gel process. The detail for preparing the particles was provided in the previous study [Citation11]. Briefly, silica nanoparticles were synthesized, followed by ion incorporation using a post-functionalization process. Then, the particles were doped with calcium (Ca) and strontium (Sr) through the addition of 3.97 g of calcium nitrate tetrahydrate (Sigma–Aldrich, St. Louis, MO, USA) and 10.67 g strontium nitrate (Sigma–Aldrich, St. Louis, MO, USA) at a nominal Si:Ca:Sr molar ratio of 1:0.5:1.5. A heat treatment process at 680 °C (holding time of 3 h) was used to dope the glass particles with Ca and Sr. The elemental composition of the obtained Sr-BGNPs was 81.0 mol% SiO2, 14.2 mol% CaO, and 4.8 mol% SrO. The diameter of the particles from the scanning electron microscope (SEM) image was approximately 200–300 nm ().
The monomer phase of the experimental provisional material () contained 70 wt% urethane dimethacrylate (UDMA, Sigma-Aldrich, St. Louis, MO, USA), 22 wt% triethylene glycol dimethacrylate (TEGDMA, Sigma–Aldrich, St. Louis, MO, USA), 3 wt% 2-hydroxyethyl methacrylate (HEMA, Sigma–Aldrich, St. Louis, MO, USA), 3 wt% 10-MDP (10-methacryloyloxydecyl dihydrogen phosphate, Watson International, Jiangsu, China), and 1 wt% CQ (camphorquinone, Sigma–Aldrich, St. Louis, MO, USA). The initiator and activator paste contained 1 wt% BPO (benzoyl peroxide, Sigma–Aldrich, St. Louis, MO, USA) and 1 wt% DMPT (N-dimethyl-p-toluidine, Sigma–Aldrich, St. Louis, MO, USA), respectively.
Table 1. Composition of commercial materials.
The powder phase contained boroaluminosilicate glass (50 nm, 0.7 µm, and 7 µm in diameter, Esstech, Essington, PA, USA) with added 10 wt% Sr-BGNPs. The decision to use 10 wt% was based on the outcomes of similar composite formulations used for preparing core build-up materials [Citation20]. The study demonstrated that adding 10 wt% Sr-BGNPs resulted in superior ion release but with acceptable strength compared to formulations containing 5 wt% Sr-BGNPs.
The initiator paste (contained BP) and activator paste (contained DMPT) were prepared using the powder-to-liquid ratio of 1.7:1 (mass ratio). They were loaded into a double-barrel syringe (medmix Switzerland AG, Haag, Switzerland). The pastes were mixed through a mixing tip with a dispenser. The commercial materials were used as the comparisons ().
Assessment of degree of monomer conversion
The degree of monomer conversion (n = 5) was determined using attenuated total reflectance Fourier transform infrared spectroscopy (ATR-FTIR, Nicolet iS5, Thermo Fisher Scientific, Waltham, MA, USA). The materials were placed on the metal ring (1 mm in thickness and 10 mm in diameter) over the ATR diamond and covered with an acetate sheet. They were covered by a metal plate to prevent exposure to light. The FTIR spectra from 400 to 400 cm−1 were recorded from the bottom surface for 10 min. The test was conducted at room temperature (25 ± 1 °C). DC was calculated using the following equation.
(1)
(1)
where
and
are the height of the peak at 1320 cm−1 (C-O of methacrylate group) [Citation21] above the background level at 1335 cm−1 before curing and at time t, respectively. The DC was then calculated through the linear extrapolation of late-time DC data versus the inverse of time as it approaches zero (as the inverse of zero is infinity) [Citation22].
Assessment of biaxial flexural strength (BFS) and modulus (BFM)
BFS and BFM of all materials (n = 5) were determined under a ball-on-ring testing jig with a mechanical testing frame (AGSX, Shimadzu, Kyoto, Japan). Firstly, disc specimens were prepared according to the protocol of the previous study. The specimens were immersed in 10 ml of deionized water for 24 and 2 weeks in an incubator (37 °C). Then, the specimens were placed on the jig under the testing frame. The load cell and crosshead speed for the test were 500 N and 1 mm/min, respectively. The fracture load was recorded (N). The BFS and BFM were calculated using the Equationequation 2(2)
(2) and Equation3
(3)
(3) , respectively.
(2)
(2)
(3)
(3)
where F refers to the maximum load (N), d is the specimen’s thickness (m), r is the radius of circular support (m), ν is Poisson’s ratio (0.3) [Citation23, Citation24],
is the rate of change of load with regard to central deflection (N/m), βc is the center deflection junction (0.5024) [Citation25], and q is the ratio of support radius to the radius of the disc. The method for calculating βc was provided in the previous study [Citation25]. The fracture surface of the representative specimen from each material was sputter-coated with Au (Q150R ES, Quorum Technologies, East Sussex, UK) using a current of 23 mA for 45 s. The fracture surface was then examined under SEM (JSM 7800 F, JEOL, Tokyo, Japan).
Assessment of color stability (ΔE*00)
Disc specimens (1 mm thick and 10 mm in diameter) were prepared as described above (n = 5). The color stability after immersion in red wine was determined using digital spectrophotometry (Easyshade V, VITA Zahnfabrik, Bad Säckingen, Germany) according to a previous study. The color of the specimens was determined before and after being immersed in red wine for 24 h and 2 weeks. The measurement was performed under the luminance of approximately 1,000 Lux over the grey background. The CIELAB coordinates (CIE L*, a*, b*, C*, and ho) were recorded. L*, a*, and b* are lightness and values on the red–green and yellow–blue axes, respectively. C* and ho are the chroma and hue angle, respectively. The color change or color stability (ΔE*00) upon immersion before and after immersion in red wine was calculated using the CIEDE2000 formula (Equationequation 4(4)
(4) ). The complete formulae were provided in a previous study [Citation26].
(4)
(4)
where
and
represent the differences in lightness, chroma, and hue, respectively, before and after immersion.
represents a rotation function related to the interaction between the chroma and hue differences in the blue region. Additionally,
and
are weighting functions, and
are the correction terms for experimental conditions.
Assessment of ion release
Disc specimens were prepared (n = 3). They were immersed in 5 ml of deionized water for 4 weeks. Then, the storage solution was collected and mixed with 2 vol% nitric acid. Inductively coupled plasma-optical emission spectrometry (ICP-OES; Optima 8300, PerkinElmer, Singapore) was used to analyze the elemental composition in the solution. The standards for Ca (317.9 nm), P (213.6 nm), and Sr (460.7 nm) were performed using the Environmental standard 26 components (CPAchem, Bogomilovo, Bulgaria). The detection range of Ca, P, and Sr were 0.1–10 ppm, 0.1–10 ppm, and 0.1–50 ppm, respectively.
Statistical analysis
Data were analyzed with Prism 10 for macOS (GraphPad Software, Boston, MA, USA). Values present in the current study are mean and 95% confidence interval (CI). The normality of data was determined using the Shapiro-Wilk test. Then, data were compared using one-way ANOVA followed by Tukey’s test. The significance level was set at p = 0.05. Power analysis was performed using G*Power version 3.1.9.6 (Heinrich-Heine-Universität Düsseldorf, Dusseldorf, Denmark) to estimate the sample size required by each test. It was estimated that a sample size of n = 5 should exhibit power > 0.95 at alpha = 0.05 for One-way ANOVA.
Results
Degree of monomer conversion
The rapid increase in DC was observed with Unifast, followed by Protemp 4, Cooltemp, and TempS10 (). The calculated DC at the late time of TempS10 (48.9 ± 9.2), Protemp4 (60.0 ± 9.9), and Cooltemp (53.4 ± 5.4) were comparable (p > 0.05) (). Unifast exhibited the highest DC (80.8 ± 0.2%), which was significantly higher than TempS10, Protemp4, and Cooltemp (p < 0.05).
Biaxial flexural strength (BFS) and modulus of elasticity (BFM)
The highest BFS at 24 h was detected from Protemp4 (194 ± 17 MPa) (), which was significantly higher than that of TempS10 (126 ± 6 MPa) (p < 0.01), Cooltemp (102 ± 12 MPa) (p < 0.01), and Unifast (123 ± 15 MPa) (p < 0.01). The BFS of TempS10 was comparable to that of Cooltemp and Unifast (p > 0.05). The BFS at 2 weeks of all materials was comparable to the value at 24 h (p > 0.05) (). The higher BFS after immersion in water for 2 weeks was obtained from Protemp4 (196 ± 14 MPa) followed by Unifast (122 ± 12 MPa), ProtempS10 (104 ± 9 MPa), and Cooltemp (89 ± 12 MPa), respectively.
Figure 3. Biaxial flexural strength (BFS) of all Materials after immersion in deionized water for (A) 24 h or (B) 2 weeks. Error bars are 95% CI (n = 5). Lines indicate p < 0.05.

For BFM at 24 h, the highest modulus was observed with TempS10 (2.7 ± 0.2 GPa), which was significantly higher than that of Protemp4 (1.9 ± 0.4 GPa), Cooltemp (1.3 ± 0.1 GPa), and Unifast (1.3 ± 0.4 GPa) (p < 0.05) (). Furthermore, no significant difference between the BFM of each material at 24 h and 2 weeks was detected (p > 0.05). The highest BFM at 2 weeks was observed with TempS10 (2.4 ± 0.2 GPa), followed by Protemp4 (1.9 ± 0.5 GPa), Unifast (1.8 ± 0.4 GPa), and Cooltemp (1.1 ± 0.3 GPa), respectively (). The fracture surface of all TempS10, Protemp4, and Cooltemp revealed fillers with polymer matrix. Voids or dislodgement of glass fillers were detected with Cooltemp (). Additionally, the agglomeration of Sr-BGNPs was observed on the fracture surface of TempS10.
Color stability (ΔE*00)
The ΔE*00 at 24 h was detected with TempS10 (3.8 ± 0.4), whilst the lowest value was detected from Unifast (0.6 ± 0.2) (). The ΔE*00 of TempS10 was significantly higher than Unifast, Protemp4 (0.9 ± 0.2), and Cooltemp (2.0 ± 0.5) (p < 0.05). A significant increase of ΔE*00 at 2 weeks was detected with all materials (p < 0.05). The highest color change at 2 weeks was obtained from TempS10 (26 ± 5), which was significantly higher than Protemp4 (2.8 ± 0.1), Cooltemp (1.7 ± 1.5), and Unifast (4.3 ± 0.9) (p < 0.05) (). The color change of Unifast was comparable to that of Protemp4 (p = 0.837), but these values were significantly lower than that of Cooltemp (p < 0.05).
Ion release
The concentrations of Ca, P, and Sr observed with PT, CT, and UF were below than that of the working range of the instrument. For TempS10, the Ca, P, and Sr concentrations in water at 2 weeks were 0.69 ± 0.10 ppm, 0.12 ± 0.02 ppm, and 3.01 ± 1.09 ppm, respectively.
Discussion
It was expected that the addition of Sr-bioactive glass nanoparticles may promote the release of essential ions, which could potentially enhance the remineralizing actions of the materials [Citation11, Citation27]. This study, therefore, examined the physical and mechanical properties of experimental resin composites containing Sr-bioactive glass nanoparticles for provisional restorations (TempS10). The experimental material demonstrated a degree of monomer conversion (DC) and strength comparable to those of commercial bis-acryl provisional materials, but with the added benefit of ion release. However, the color stability of TempS10 was significantly lower than that of other commercial materials. Hence, the null hypothesis was partially rejected.
The DC results obtained from the commercial materials were similar to those reported in the previous study [Citation28]. DC observed for each material was primarily influenced by the composition of the monomer system present in the material. The high DC, as observed with Unifast, is usually associated with a high exothermic reaction, which could potentially affect the dentin-pulp complex. A study reported that the peak temperature recorded with at the center and peripheral areas of PMMA disc specimen were 73.9 ± 8.6 °C and 59.2 ± 5.3 °C, respectively [Citation29]. It was also demonstrated that PMMA polymerization induces a significant temperature increase (4–5 °C) in dentine, which was higher than that (1–3 °C) observed with bis-acryl materials [Citation3].
It should be mentioned that the actual composition and concentration of each commercial product were not obtained from suppliers, thus limiting the direct comparison with the experimental material. A high DC for resin composite-based materials may be partially correlated with a low risk of toxic monomer release. A study indicated a strong inverse correlation between the increase in DC (from 22.4 to 46.5%) and the amount of monomers eluted from the resin composites [Citation30]. Given that the DC of composite provisional materials in the current study was approximately higher than 50%, this may suggest the low risk of toxic monomer release. However, elution studies and cytotoxicity tests should be employed in future studies.
The strength observed in commercial materials was slightly higher than the reported value in the published study (Protemp4 = 113 MPa, Unifast = 64 MPa) [Citation31, Citation32]. This discrepancy might be attributed to variations in testing protocols used across different studies. For instance, the specimens in the current study were tested at the initial time point and did not undergo thermally accelerated aging. This may not represent the long-term service simulation, which was considered as the limitation of the current study. Additionally, the current study allowed the specimen to be set for 24 h before immersion. This could subsequently increase the degree of polymerization and the observed mechanical strength of the specimen.
The addition of filler particles with a diameter in micron size (7 µm) and sub-micron sizes (0.7 µm, 500 nm) could lead to the high stiffness observed with TempS10. Furthermore, the estimated filler load of TempS10 (∼63 wt%) was also higher than that of commercial materials such as Protemp4 (24–45 wt%) [Citation33]. The combination of glass of various sizes and nanoparticles was also expected to enhance the filler packing [Citation34]. The agglomeration of Sr-BGNPs in some areas was observed with TempS10, which could negatively affect the strength of the material. It was speculated that the smooth fracture surface of Protemp4 may be attributed to the effective dispersion of small glass fillers (50 nm) [Citation35], leading to efficient stress transfer and increased mechanical strength in comparison to other materials [Citation36, Citation37]. However, the minimum flexural strength required by ISO 10477-2018 (Polymer-based crown and veneering materials) was 50 MPa [Citation38]. The results from the current study suggest that the experimental material still meets the requirement of the standard. Future work should employ a longer immersion time or use an accelerating protocol to compare the long-term mechanical performance of the materials. This may ensure the durability of the material when a long-term temporization period is needed.
The major limitation of experimental material in the current study is its low color stability. It was reported that viewers can detect color differences as small as ΔE*00=0.8, while the acceptable threshold is ΔE*00=1.8 [Citation39]. The results from the current study indicated that Protemp4 and Unifast exhibited color stability in an acceptable range after immersion in red wine at 24 h immersion. The monomer and fillers systems contained in materials influenced the color stability of resin-based materials [Citation40]. Furthermore, it may be possible that the color stability of materials is associated with the surface roughness, which leads to the increase in the accumulation of pigments. A previous study showed that the Protemp4, which contained only nanosized filler, exhibited the lowest surface roughness compared with other materials [Citation41]. This could explain the low ΔE*00 observed in Protemp4 in the current study. The low color stability of TempS10 and Cooltemp could be due to the containing of large glass fillers, which may increase the surface irregularities that could promote the deposition of pigments. It is also possible that the release of Sr-BGNPs from the surface of experimental provisional material may enhance surface irregularities. The use of large fillers may additionally result in poor wear resistance. The crack propagation at the interface of filler and matrix leads to the loss of filler particles [Citation42]. This should be examined using surface roughness studies such as atomic force microscopy or profilometry in future work. A previous study also suggested that the chemical reaction between ions due to the dissolution of bioactive glass and components in beverages could contribute to the increase in color changes of composites containing bioactive glass [Citation43]. The elution of components such as fillers and monomers also affected color stability of the materials, this should be further examined using HPLC in future study.
The release of essential elements such as Ca, P, and Sr from the experimental material was expected to encourage remineralization for the demineralized dentin [Citation10, Citation12]. The previous study proposed that Sr may enhance the acid resistance of dental hard tissue via the transformation of hydroxyapatite to Sr-substitute apatite, which may help promote antibacterial action [Citation44]. The greater strontium release than other ions could be because they dissolve from the doped area, while the release of calcium and phosphorous involves dissolving from the glass network. The limitation of this study was the absence of an experimental control (i.e. the formulation without any additive), which could influence the accurate determination of the beneficial effects of Sr-BGNPs.
The concentration of calcium released from TempS10 was still lower than that of commercially available hybrid resin composite used for restoration (∼1–2 ppm, ACTIVA BioActive-Restorative, Pulpdent, Watertown, MA, USA) [Citation45, Citation46]. The concentration of all ions released was also lower than that observed with resin-based composite used in orthodontic adhesives in the previous studies [Citation11, Citation47]. An explanation could be that the materials prepared in the earlier studies contained a highly soluble monocalcium phosphate monohydrate (MCPM, solubility = 783 g/L, Ca/P ratio = 0.5) [Citation48, Citation49]. Combining MCPM with bioactive glass nanoparticles may enhance the water sorption [Citation50] and accelerate glass degradation, but this could also reduce the color stability and strength of the materials. It should be emphasized that the current study is an in vitro study. The need for further assessment of dentin remineralization using the suggested methods [Citation10], such as XRD, FTIR, or Raman spectroscopy, should be confirmed in future work prior to assessment in in vivo studies.
Conclusion
Considering the limitations imposed by the study methods, it could be concluded that experimental material containing Sr-BGNPs showed a comparable degree of monomer conversion and mechanical strength to commercial resin composite-based provisional materials. The experimental materials showed lower color stability but could provide the release of essential ions for mineralization, such as Ca, P, and Sr. This was expected to promote remineralizing actions for the material.
Author contribution
Conception: PP, CP, and PN; methodology: PP, CP, and PN; data analysis: NS, HC; manuscript preparation: NS, HC, CP, PN, and PP; review and editing: PN and PP.
Acknowledgments
This study was supported by Faculty of Dentistry Thammasat University Research Fund (Contract no. 4/2565) and Thammasat University Research Unit in Dental and Bone Substitute Biomaterials, Thammasat University, Thailand. The author would like to sincerely thank medmix Switzerland AG for their kind support in the mixing apparatus for preparing the experimental provisional material.
Data availability statement
The data that support the findings of this study are available from the corresponding author upon reasonable request.
Disclosure statement
No potential conflict of interest was reported by the authors.
Correction Statement
This article has been corrected with minor changes. These changes do not impact the academic content of the article.
Additional information
Funding
References
- Sadek HMA, El-Banna A. Biaxial flexural strength of different provisional restorative materials under chemo-mechanical aging: an in vitro study. J Prosthodont. 2023;1-8. doi: 10.1111/jopr.13662.
- Pituru SM, Greabu M, Totan A, et al. A review on the biocompatibility of PMMA-Based dental materials for interim prosthetic restorations with a glimpse into their modern manufacturing techniques. Materials (Basel). 2020;13(13):2894. doi: 10.3390/ma13132894.
- Altintas SH, Yondem I, Tak O, et al. Temperature rise during polymerization of three different provisional materials. Clin Oral Investig. 2008;12(3):283–286. doi: 10.1007/s00784-007-0163-7.
- Gautam R, Singh RD, Sharma VP, et al. Biocompatibility of polymethylmethacrylate resins used in dentistry. J Biomed Mater Res B Appl Biomater. 2012;100(5):1444–1450. doi: 10.1002/jbm.b.32673.
- Jun SK, Cha JR, Knowles JC, et al. Development of Bis-GMA-free biopolymer to avoid estrogenicity. Dent Mater. 2020;36(1):157–166. doi: 10.1016/j.dental.2019.11.016.
- Floyd CJ, Dickens SH. Network structure of Bis-GMA- and UDMA-based resin systems. Dent Mater. 2006;22(12):1143–1149. doi: 10.1016/j.dental.2005.10.009.
- Macedo M, Volpato CAM, Henriques B, et al. Color stability of a bis-acryl composite resin subjected to polishing, thermocycling, intercalated baths, and immersion in different beverages. J Esthet Restor Dent. 2018;30(5):449–456. Epub 20180908 doi: 10.1111/jerd.12404.
- Ozel GS, Guneser MB, Inan O, et al. Evaluation of C. Albicans and S. Mutans adherence on different provisional crown materials. J Adv Prosthodont. 2017;9(5):335–340. Epub 20171016 doi: 10.4047/jap.2017.9.5.335.
- Dai LL, Mei ML, Chu CH, et al. Mechanisms of bioactive glass on caries management: a review. Materials (Basel). 2019;12(24):4183. Epub 20191212. doi: 10.3390/ma12244183.
- Fernando D, Attik N, Pradelle-Plasse N, et al. Bioactive glass for dentin remineralization: a systematic review. Mater Sci Eng C Mater Biol Appl. 2017;76:1369–1377. Epub 20170314 doi: 10.1016/j.msec.2017.03.083.
- Chaichana W, Insee K, Chanachai S, et al. Physical/mechanical and antibacterial properties of orthodontic adhesives containing Sr-bioactive glass nanoparticles, calcium phosphate, and andrographolide. Sci Rep. 2022;12(1):6635. doi: 10.1038/s41598-022-10654-6.
- Dai LL, Mei ML, Chu CH, et al. Remineralizing effect of a new strontium-doped bioactive glass and fluoride on demineralized enamel and dentine. J Dent. 2021;108:103633. Epub 20210311. doi: 10.1016/j.jdent.2021.103633.
- Sasaki JI, Kiba W, Abe GL, et al. Fabrication of strontium-releasable inorganic cement by incorporation of bioactive glass. Dent Mater. 2019;35(5):780–788. Epub 20190301 doi: 10.1016/j.dental.2019.02.019.
- Baheiraei N, Eyni H, Bakhshi B, et al. Effects of strontium ions with potential antibacterial activity on in vivo bone regeneration. Sci Rep. 2021;11(1):8745. doi: 10.1038/s41598-021-88058-1.
- Jang JH, Lee MG, Ferracane JL, et al. Effect of bioactive glass-containing resin composite on dentin remineralization. J Dent. 2018;75:58–64. Epub 20180525 doi: 10.1016/j.jdent.2018.05.017.
- Potiprapanpong W, Naruphontjirakul P, Khamsuk C, et al. Assessment of mechanical/chemical properties and cytotoxicity of resin-modified glass ionomer cements containing Sr/F-bioactive glass nanoparticles and methacrylate functionalized polyacids. Int J Mol Sci. 2023;24(12):10231. doi: 10.3390/ijms241210231.
- Khvostenko D, Hilton TJ, Ferracane JL, et al. Bioactive glass fillers reduce bacterial penetration into marginal gaps for composite restorations. Dent Mater. 2016;32(1):73–81. doi: 10.1016/j.dental.2015.10.007.
- Salehi S, Gwinner F, Mitchell JC, et al. Cytotoxicity of resin composites containing bioactive glass fillers. Dent Mater. 2015;31(2):195–203. doi: 10.1016/j.dental.2014.12.004.
- Korkut E, Torlak E, Altunsoy M. Antimicrobial and mechanical properties of dental resin composite containing bioactive glass. J Appl Biomater Funct Mater. 2016;14(3):e296-301–e301. Epub 20160726 doi: 10.5301/jabfm.5000271.
- Mirchandani B, Padunglappisit C, Toneluck A, et al. Effects of Sr/F-Bioactive glass nanoparticles and calcium phosphate on monomer conversion, biaxial flexural strength, surface microhardness, mass/volume changes, and color stability of Dual-Cured dental composites for core Build-Up materials. Nanomaterials (Basel). 2022;12(11):1897. doi: 10.3390/nano12111897.
- Delgado AHS, Young AM. Methacrylate peak determination and selection recommendations using ATR-FTIR to investigate polymerisation of dental methacrylate mixtures. PLoS One. 2021;16(6):e0252999. doi: 10.1371/journal.pone.0252999.
- Panpisut P, Khan MA, Main K, et al. Polymerization kinetics stability, volumetric changes, apatite precipitation, strontium release and fatigue of novel bone composites for vertebroplasty. PLoS One. 2019;14(3):e0207965. doi: 10.1371/journal.pone.0207965.
- Akinmade AO, Nicholson JW. Poisson’s ratio of glass-polyalkenoate (glass-ionomer) cements determined by an ultrasonic pulse method. J Mater Sci: mater Med. 1995;6(8):483–485. doi: 10.1007/BF00123374.
- Chung SM, Yap AU, Koh WK, et al. Measurement of Poisson’s ratio of dental composite restorative materials. Biomaterials. 2004;25(13):2455–2460. doi: 10.1016/j.biomaterials.2003.09.029.
- Higgs WA, Lucksanasombool P, Higgs RJ, et al. A simple method of determining the modulus of orthopedic bone cement. J Biomed Mater Res. 2001;58(2):188–195. doi: https://doi.org/10.1002/1097-4636(2001)58:2<188::AID-JBM1006>3.0.CO;2-v.
- Ardu S, Duc O, Di Bella E, et al. Color stability of recent composite resins. Odontology. 2017;105(1):29–35. Epub 20160218 doi: 10.1007/s10266-016-0234-9.
- Panpisut P, Praesuwatsilp N, Bawornworatham P, et al. Assessment of physical/mechanical performance of dental resin sealants containing Sr-Bioactive glass nanoparticles and calcium phosphate. Polymers (Basel). 2022;14(24):5436. doi: 10.3390/polym14245436.
- Padunglappisit C, Posaya-Anuwat S, Sompoch V, et al. Effects of different amine activators on the monomer conversion, biaxial flexural strength, and color stability of experimental provisional dental restorations. Eur J Dent. 2021;15(3):488–494. doi: 10.1055/s-0040-1721908.
- Fang J, Shen J, Jiang W, et al. Cytotoxicity of polymethyl methacrylate cement on primary cultured metastatic spinal cells. Mol Cell Toxicol. 2016;12(2):125–132. doi: 10.1007/s13273-016-0016-z.
- Durner J, Obermaier J, Draenert M, et al. Correlation of the degree of conversion with the amount of elutable substances in nano-hybrid dental composites. Dent Mater. 2012;28(11):1146–1153. Epub 20120831 doi: 10.1016/j.dental.2012.08.006.
- Mehrpour H, Farjood E, Giti R, et al. Evaluation of the flexural strength of interim restorative materials in fixed prosthodontics. J Dent (Shiraz). 2016;17(3):201–206.
- Yao J, Li J, Wang Y, et al. Comparison of the flexural strength and marginal accuracy of traditional and CAD/CAM interim materials before and after thermal cycling. J Prosthet Dent. 2014;112(3):649–657. Epub 20140412 doi: 10.1016/j.prosdent.2014.01.012.
- Wuersching SN, Hickel R, Edelhoff D, et al. Initial biocompatibility of novel resins for 3D printed fixed dental prostheses. Dent Mater. 2022;38(10):1587–1597. Epub 20220822 doi: 10.1016/j.dental.2022.08.001.
- Wang R, Habib E, Zhu XX. Evaluation of the filler packing structures in dental resin composites: from theory to practice. Dent Mater. 2018;34(7):1014–1023. Epub 20180416 doi: 10.1016/j.dental.2018.03.022.
- Santing HJ, Kleverlaan CJ, Werner A, et al. Occlusal wear of provisional implant-supported restorations. Clin Implant Dent Relat Res. 2015;17(1):179–185. Epub 20130417 doi: 10.1111/cid.12072.
- Glaskova T, Zarrelli M, Borisova A, et al. Method of quantitative analysis of filler dispersion in composite systems with spherical inclusions. Compos Sci Technol. 2011;71(13):1543–1549. doi: 10.1016/j.compscitech.2011.06.009.
- Aminoroaya A, Neisiany RE, Khorasani SN, et al. A review of dental composites: challenges, chemistry aspects, filler influences, and future insights. Compos. B: Eng. 2021;216:108852. doi: 10.1016/j.compositesb.2021.108852.
- British Standard. Dentistry polymer-based crown and veneering materials (ISO 10477:2018). Switzerland: BSI Standards; 2018.
- Paravina RD, Ghinea R, Herrera LJ, et al. Color difference thresholds in dentistry. J Esthet Restor Dent. 2015;27 Suppl 1(S1): s 1–9. Epub 20150417 doi: 10.1111/jerd.12149.
- Fonseca AS, Labruna Moreira AD, de Albuquerque PP, et al. Effect of monomer type on the CC degree of conversion, water sorption and solubility, and color stability of model dental composites. Dent Mater. 2017;33(4):394–401. Epub 20170227 doi: 10.1016/j.dental.2017.01.010.
- Gantz L, Fauxpoint G, Arntz Y, et al. In vitro comparison of the surface roughness of polymethyl methacrylate and bis-acrylic resins for interim restorations before and after polishing. J Prosthet Dent. 2021;125(5):833 e1–e10. Epub 20210306 doi: 10.1016/j.prosdent.2021.02.009.
- Elfakhri F, Alkahtani R, Li C, et al. Influence of filler characteristics on the performance of dental composites: a comprehensive review. Ceram Int. 2022;48(19):27280–27294. doi: 10.1016/j.ceramint.2022.06.314.
- Tuncdemir MT, Gulbahce N. Addition of antibacterial agent effect on color stability of composites after immersion of different beverages. J Esthet Restor Dent. 2019;31(5):508–513. Epub 20190718 doi: 10.1111/jerd.12513.
- Saghiri MA, Vakhnovetsky J, Vakhnovetsky A, et al. Functional role of inorganic trace elements in dentin apatite tissue-Part 1: Mg, Sr, Zn, and Fe. J Trace Elem Med Biol. 2022;71:126932. Epub 20220115. doi: 10.1016/j.jtemb.2022.126932.
- Jun SK, Lee JH, Lee HH. The biomineralization of a bioactive glass-incorporated light-curable pulp capping material using human dental pulp stem cells. Biomed Res Int. 2017;2017:2495282–2495289. doi: 10.1155/2017/2495282.
- Bhatia K, Nayak R, Ginjupalli K. Comparative evaluation of a bioactive restorative material with resin modified glass ionomer for calcium-ion release and shear bond strength to dentin of primary teeth-an in vitro study. J Clin Pediatr Dent. 2022;46(6):25–32. Epub 20221101 doi: 10.22514/jocpd.2022.022.
- Chanachai S, Chaichana W, Insee K, et al. Physical/mechanical and antibacterial properties of orthodontic adhesives containing calcium phosphate and nisin. J Funct Biomater. 2021;12(4):73. doi: 10.3390/jfb12040073.
- Jeong J, Kim JH, Shim JH, et al. Bioactive calcium phosphate materials and applications in bone regeneration. Biomater Res. 2019;23(1):4. doi: 10.1186/s40824-018-0149-3.
- Nasri K, El Feki H, Sharrock P, et al. Spray-Dried monocalcium phosphate monohydrate for soluble phosphate fertilizer. Ind Eng Chem Res. 2015;54(33):8043–8047. doi: 10.1021/acs.iecr.5b02100.
- Par M, Spanovic N, Bjelovucic R, et al. Long-term water sorption and solubility of experimental bioactive composites based on amorphous calcium phosphate and bioactive glass. Dent Mater J. 2019;38(4):555–564. Epub 20190201 doi: 10.4012/dmj.2018-145.