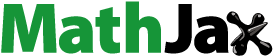
Abstract
Integrating organic light-emitting diodes (OLEDs) with light therapy can significantly advance biomedical applications by offering non-invasive, precise, and customizable treatments. Developing innovative device designs and performing cellular tests is essential to assess the safety and effect of OLED-based light therapies in dermatology. As such, a wearable OLED medical device prototype has been created for easy attachment to the body, ensuring consistent exposure to skin injuries due to its comfortable fit and user-friendly operation. Moreover, it has demonstrated effectiveness in enhancing wound healing, as indicated by a max 23% increase in fibroblast proliferation at the cellular level.
1. Introduction
Light therapy has emerged as a promising non-invasive treatment for various medical conditions, offering many benefits with minimal side effects [Citation1,Citation2]. Recent advancements in next-generation technology using micro-LEDs [Citation3,Citation4] or OLEDs [Citation5–13] have created opportunities for innovative light-based therapies that can enhance patients’ well-being and overall healthcare. Micro-LEDs are the next-generation light sources fabricated by transferring LEDs at a scale of m. They offer numerous advantages over traditional LEDs, including higher brightness, faster response time, high resolution, and longer lifespan. However, there are several obstacles to use in the field of phototherapy. These include the heat effect inherent to LEDs and the challenge of achieving uniform irradiation due to the limitations of point light sources. Additionally, there are potential hazards associated with using UV or blue LEDs required for generating specific wavelengths. Furthermore, the transfer process of micro-LEDs required for commercialization is highly complex and expensive, posing a significant challenge in bringing the technology to market.
On the other hand, OLEDs have found widespread applications beyond television and mobile devices, extending to the automotive and smart wearable sectors, owing to their exceptional advantages [Citation14–16]. Capitalizing on their inherent flexibility and wearability, OLED technology can be incorporated into diverse form factors, enhancing multiple aspects of our daily lives. OLEDs offer various unique features, such as flexibility, thinness, and customizable wavelength, that enable their integration into wearable devices. In addition, OLED has the unique ability to emit light on its own, which is its greatest merit in phototherapy, as it allows for a uniform distribution of light. As the importance of biomedical engineering is being emphasized, and interdisciplinary research between medicine and engineering is thriving, applying OLED technology in medical devices can improve patient experiences. Therefore, a patient-friendly, daily treatment, and easy-to-use manual-based wearable OLED medical device with a form factor that can be attached to the human body was needed.
2. Experiments
2.1. Prototype of body-attached wearable OLED medical device
This medical device consists of three main components: an OLED for wound healing, a printed circuit board assembly (PCBA) to drive the OLED, and a dressing film to absorb wound exudate. First, the OLED panel (Konica Minolta, A9F4C0A) was used as the light source for the medical device [Citation17,Citation18]. This panel’s electrical and optical characteristics can be seen in Figure . The electrical and optical characteristics were measured using CS-2000 (Konica Minolta). It generally emits enough radiance for photobiomodulation (PBM) for wound healing (Figure a) [Citation2,Citation6]. This device uniformly emits red light at 633 nm, which is effective for wound healing, as presented in Figure b [Citation2]. Unlike conventional lasers, LEDs, and micro-LEDs, OLED is an ideal real surface source and is flexible, allowing it to adhere closely to the skin. The light should be evenly irradiated at various angles on the wound site to ensure uniform effectiveness. Therefore, the intensity was measured at different angles, and the results showed some spectral variation depending on the angle, but the angle dependence was very low, as depicted in Figure c. Additionally, the device is close to a Lambertian light distribution (Figure d), meaning that the light can be uniformly irradiated at the same wavelength on all body parts when attached. At this time, if excessive heat is generated when the light is irradiated, the mechanism of the therapeutic effect becomes unclear due to the effects of light and heat. Therefore, thermal stability and reliability tests were conducted. The temperature change over time (measured by a thermal imaging camera, Fotric 228) and the lifespan (measured by Polaronix M6000 OLED lifetime tester) were measured at the conditions of 5 mW/cm2 used for wound healing, as demonstrated in Figure . The focus was initially adjusted, and body temperature was measured before temperature measurement to ensure accuracy and reliability of the measurements. Subsequently, the emitting area of the light source was measured at 5 min intervals for 40 min, and the maximum temperature was recorded. As a result, the generating heat was between 25–26 C, indicating that there would be no heat effects and skin burns. (Figure a) Furthermore, the device maintained approximately 95% of its initial values after over 2500 hrs (LT 95 > 2500 h), suggesting that there would be no changes in light intensity during the treatment period. (Figure b)
Figure 1. Electrical and optical characteristics of OLED (a) J-V-R; (b) EL spectra (inset: photo of emitting red light); (c) angular EL spectrum from 0 to 70 at the same current density; and (d) polar diagram compared to ideal Lambertian source.
Figure 2. (a) Graph of generating heat depending on the time from OLED. (inset: photo of measuring temperature using a thermal camera) and (b) operating lifetime at target radiant emittance (5 mW/cm2).
Figure illustrates a specially designed PCBA for driving the OLED. The PCBA receives power from a 12 V 2A adapter and includes an on/off switch for the system. Upon powering on, the display indicates the current and time, which can be modified using the setup button. The current is adjustable in 1 mA increments from 0 to 35 mA, while the timer allows for min and sec settings. When the desired values are input, and the process begins, a green LED indicator illuminates, the OLED starts operating, and the system shuts down automatically when the predetermined treatment duration concludes. The design prioritizes maximum usability, ensuring a seamless and user-friendly experience for users.
Lastly, the OLED medical device prototype for wound healing should be used with a dressing film for exudate absorption. When exudate forms from a wound, directly attaching an OLED may cause damage to the OLED due to the exudate and may even reduce the wound-healing effect. Therefore, using a dressing film that can prevent primary contamination along with the OLED can produce a synergistic effect, taking the benefits of light therapy. However, as various types of dressing films are available on the market, it is important to choose the appropriate one. Gauze, a widely known traditional dressing method, may be inexpensive; however, it has the drawback of allowing the wound to dry out easily. Hydrocolloid and Mepiform dressing films, which are popular among consumers, also provide a moist environment and protect the wound from contamination, but they share the disadvantage of being opaque. In contrast, 3M Tegaderm film-type dressings are extremely thin and transparent, offering a dual benefit when combined with OLEDs, as shown in Figure . Figure a shows the transmittance of various types of dressing film, such as gauze, hydrocolloid products, Mepiform, and 3M Tegaderm film, to check synergistic effects with OLED and whether light can pass through sufficiently or not. While most dressing films have a transmittance of less than 30% in the visible light spectrum, the 3M Tegaderm film exhibits a high transmittance of nearly 80%, which allows for the expectation of a positive synergy effect with OLEDs. Actually, when 3M Tegaderm dressing film and OLED light sources were attached, there was no loss of light intensity, as exhibited in Figure b. The results showed that regardless of the presence of the dressing film, the change in light intensity was negligible, allowing the dressing to serve its purpose while also benefiting from the phototherapy. OLEDs are susceptible to moisture and oxygen, which can cause damage when exposed to bodily fluids such as wound exudate. However, this medical device employs a dressing film as the primary barrier to prevent damage to the OLED. Additionally, the OLED is protected from external damage through encapsulation on the top and bottom surfaces.
Figure 4. (a) Transmittance of various dressing films including glass (reference) in the visible region (400 to 750 nm) and (b) changes in radiance depending on the presence or absence of Tegaderm film.
Figure presents the final prototype of the medical device. It is attachable to the human body, very thin, flexible, and can be combined with a transparent dressing film for wound dressing and phototherapy, making it a wearable OLED medical device, as denoted in Figure a,b. Moreover, the appearance of the medical device, created based on the components, can be seen in Figure c,d. It has been designed as a product that users can comfortably wear and enables daily treatment. In this study, we aimed to verify the cell proliferation of the OLED for actual therapeutic effects.
2.2. Process of cell culture
Cell experiments were performed to validate the therapeutic effects. Human fibroblasts were isolated from foreskin samples collected during circumcisions, with informed consent from all participants. All procedures were conducted in accordance with relevant guidelines and regulations, and the study received approval from the Seoul National University Bundang Hospital Institutional Review Board (IRB Approval No. B-1603/340-309). Fibroblasts were cultured in Dulbecco's Modified Eagle's Medium (DMEM, LM001-05, WelGENE, Daegu, South Korea), supplemented with 10% fetal bovine serum (FBS, SH30919.03, HyClone by Cytiva, Logan, UT), and 1 × antibiotic-antimycotic (15240, Gibco by Life Technologies, Grand Island, NY).
2.3. Cell-based in-vitro experiments
Once the cell culture preparation is complete, OLED irradiation is performed, and cell toxicity and proliferation are observed using the cell counting kit-8 (CCK-8, Dojindo, Kumamoto, Japan) assay method. CCK-8 is a widely used kit for the rapid and highly sensitive detection of cell proliferation and toxicity, based on WST-8 (2-(2-Methoxy-4-nitrophenyl)-3-(4-nitrophenyl)-5-5(2,4-disulfophenyl)-2H-tetrazolium sodium salt). WST-8 can be reduced to a highly water-soluble yellow formazan product by dehydrogenases within mitochondria. The intensity of the yellow color is directly proportional to cell proliferation and inversely proportional to cell toxicity.
The non-toxic nature of OLEDs has been extensively reported in our research group [Citation5,Citation6]. To measure cell proliferation, standard human fibroblasts were seeded in 96-well plates at a density of 2,000 cells per well and cultured for 24 h. Subsequently, cells underwent serum deprivation for an additional 4-8 h, followed by OLED irradiation at the recommended dosage. After 72 h, the CCK-8 solution was introduced to each well and allowed to incubate for 2 h. Absorbance at a wavelength of 450 nm was quantified utilizing a microplate spectrophotometer (Epoch2, BioTek Instruments, Inc., Winooski, VT).
First, the RED OLED was exposed through a 96-well plate connected with PCBA. Areas covered with Al foil were set as control groups unaffected by light, and cells exposed to the outside were irradiated with the RED OLED, as indicated in Figure . Figure a illustrates that among the 96 wells, the black squares (4 wells) covered with foil were set as the control group (CTL), and the proliferation of cells in the red squares (4 wells) directly exposed to the light source was analyzed. During this process, the examination conditions were set at 5 mW/cm2, with a 10-30 min exposure to observe the proliferation rate of human fibroblasts. The actual exposure setup can be seen in Figure b. As a result, it was confirmed that a maximum of 23% cell proliferation occurred in the group exposed to light for 30 min compared to the control group without light exposure, as shown in Figure . This result appears consistent with the cell proliferation tendency reported by our group for the 630 nm OLED [Citation5,Citation6]. However, it is not simply that continuous exposure to light results in better healing effects, but rather that there is an optimal range where the healing effects are maximized, and beyond a certain level, the effects begin to decrease. This phenomenon is typically seen in low-level light therapy and is known as a biphasic response [Citation19]. Therefore, additional research is needed to find the optimal energy dose. Nevertheless, through this cell experiment, OLEDs can effectively contribute to the proliferation phase of the wound healing process, and leveraging the unique advantages of OLEDs could open up new markets in medical devices.
3. Conclusion
In conclusion, this study presents a novel body-attached wearable OLED medical device for cutaneous wound healing, offering effective and non-invasive treatment. Combining the unique features of OLED technology with a user-friendly design and a transparent dressing film provides uniform light irradiation, promoting wound healing without compromising patient comfort. This innovative approach has the potential to significantly enhance patient care and revolutionize the field of light-based therapies in dermatology. Beginning with this prototype, research and development of OLED medical devices will flourish, ultimately providing patients with a unique and special experience in their treatment.
Disclosure statement
No potential conflict of interest was reported by the author(s).
Additional information
Funding
Notes on contributors

Yongjin Park
Yongjin Park received his BS in Electronic and Electrical Engineering from the Sungkyunkwan University, South Korea, in 2018. He obtained his MS and Ph.D degree from Korea Advanced Institute of Science and Technology (KAIST), South Korea, in 2020 and 2023, respectively. His main research topic involves biomedical applications using wearable OLED.

Hye-Ryung Choi
Hye-Ryung Choi received her PhD degree from Seoul National University in 2006. She is currently a research professor at the Seoul National University Bundang Hospital, South Korea. Her main research subject is the in vitro & in vivo evaluation of medical devices such as LED, OLED, or 3D bio-printers, considering suitable evaluation methods, as well as wound healing, keloids, fine dust, and artificial skin models.

Jung Won Shin
Jung-Won Shin received her MD degree in 2006 and PhD degree in 2017 at Seoul National University. She is currently an associate professor in the Department of Dermatology, Seoul National University Bundang Hospital. Her main clinical and research topics include hair and wound biology.

Chang-Hun Huh
Chang-Hun Huh received his MD degree in 1995 and PhD degree in 2007 at Seoul National University. He is currently a full clinical professor and chairman of the Department of Dermatology at Seoul National University Bundang Hospital. He is a clinical dermatologist, dermatologic surgeon, an actively working scientist, and a consultant for the government and companies on medical devices, cosmetics, and cell treatment.

Kyung Cheol Choi
Kyung Cheol Choi received his PhD degree from Seoul National University in 1993. He is currently a full professor in the School of Electrical Engineering, KAIST, and an IEEE fellow, South Korea. Prof. Choi and his research group, Advanced Display and Nano Convergence Lab (ADNC) are focusing on wearable/stretchable displays and their application to bio/medical devices.
References
- M.R. Hamblin, Photochem. Photobiol 94, 199 (2018).
- H. Chung, T. Dai, S.K. Sharma, Y.Y. Huang, J.D. Carroll, and M.R. Hamblin, Ann. Biomed. Eng 40, 516 (2012).
- S.Y. Lee, S. Jeon, Y.W. Kwon, M. Kwon, M.S. Kang, K.Y. Seong, T.E. Park, S.Y. Yang, D.W. Han, S.W. Hong, and K.S. Kim, Sci. Adv 8, 44 (2022).
- J.H. Lee, Y. Ahn, H.E. Lee, Y.N. Jang, A.Y. Park, S. Kim, Y.H. Jung, S.H. Sung, J.H. Shin, S.H. Lee, S.H. Park, K.S. Kim, M.S. Jang, B.J. Kim, S.H. Oh, and K.J. Lee, Adv. Healthc. Mater 12, 1 (2023).
- Y. Park, H.R. Choi, Y. Jeon, H. Kim, J.W. Shin, C.H. Huh, K.C. Park, and K.C. Choi, Sci. Rep 12, 1 (2022).
- Y. Jeon, H.R. Choi, M. Lim, S. Choi, H. Kim, J.H. Kwon, K.C. Park, and K.C. Choi, Adv. Mater. Technol 3, 1 (2018).
- Y. Jeon, H.R. Choi, J.H. Kwon, S. Choi, K.M. Nam, K.C. Park, and K.C. Choi, Light Sci. Appl 8, 1 (2019).
- Y. Jeon, I. Noh, Y.C. Seo, J.H. Han, Y. Park, E.H. Cho, and K.C. Choi, ACS Nano (2020). doi:10.1021/acsnano.0c06649.
- Y.G. Park, E. Cha, H.S. An, K.P. Lee, M.H. Song, H.K. Kim, and J.U. Park, Nano Res 13, 1347 (2020).
- S. Choi, Y. Jeon, J.H. Kwon, C. Ihm, S.Y. Kim, and K.C. Choi, Adv. Sci 9, 1 (2022).
- H. Kim, H.R. Choi, Y. Park, Y. Jeon, H.S. Lee, E.G. Jeong, K.C. Park, and K.C. Choi, Adv. Mater. Interfaces 8, 1 (2021).
- Y. Jeon, H.R. Choi, K.C. Park, and K.C. Choi, J. Soc. Inf. Disp 28, 324 (2020).
- Y. Park, G.S. Lee, H. Choi, C. Huh, Y. Kim, K.C. Choi, and R. Korea, MRS (2022).
- J. Song, H. Lee, E.G. Jeong, K.C. Choi, and S. Yoo, Adv. Mater 32, 1 (2020).
- S. Choi, Y. Na, J. Lee, and K.C. Choi, Dig. Tech. Pap. - SID Int. Symp 52, 279 (2021).
- Y. Park, B. Noh, and K. C. Choi, IMID Invited talk (2022).
- Y. Miyata, R. Nakabayashi, K. Tabata, N. Ueda, T. Yamada, S. Kon, and T. Tsujimura, Infrared Down-Conversion of Organic Light Emitting Diode Emission for Medical Use and Vein Authentication (2020).
- T. Tsujimura, J. Fukawa, K. Endoh, Y. Suzuki, K. Hirabayashi, and T. Mori, J. Soc. Inf. Disp 22, 412 (2014).
- M.R. Hamblin, Y.Y. Huang, S.K. Sharma, and J. Carroll, Dose-Response 9, 602 (2011).