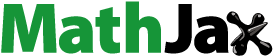
ABSTRACT
Unlike existing flexible display technologies such as foldable, rollable, or slidable displays, stretchable displays can change the size of surface areas. Furthermore, they can be transformed into a multi-curvature shape in any direction. These may be the reasons they are often called freeform displays. Stretchability, the extent displays can be stretched, is one of the most important performance factors of stretchable displays. The length ratio before and after stretching is widely utilized to represent one- or two-dimensional stretchability. However, the length ratio cannot be directly applied to three-dimensional stretching. Hence, this paper introduces new measures of three-dimensional stretchability. The pros and cons of the proposed measures are explained. In addition, experimental results to evaluate the proposed measures’ performance are presented.
1. Introduction
Various types of flexible displays have been introduced to the market, including foldable, rollable, and slidable types. Regardless of the types of flexible displays, contents are mostly displayed on a flat surface or a curved surface with a single curvature. Recently, academic institutions and display industries have developed a new type of flexible display called stretchable display [Citation1–41]. Unlike existing flexible display technologies, the size of the surface area can be changed. Furthermore, stretchable displays can have shapes with multi-curvatures in any direction. A wearable application that adheres to the back of the hand can be a good example [Citation2,Citation3]. Thus, stretchable displays are referred to as freeform displays.
Stretchable displays with island-bridge structure [Citation8–18] have a unique pixel structure. A pixel area can be divided into rigid and stretchable subregions. Light emitting devices, for example, LEDs (including mini LED and micro LED) and OLEDs, are placed in the rigid subregion that remains unchanged even if an external force is applied. The stretchable subregions contain elastic interconnectors to neighboring pixels. The types of stretching that can be applied to stretchable displays vary widely depending on applications. For instance, a stretchable display can be regarded as two-dimensional stretching when the area size is changed but the flat shape is maintained. When a stretchable display no longer has a flat shape after stretching, it can be called three-dimensional stretching [Citation4–6]. An example of three-dimensional stretching may be the case of poking a stretchable display from the bottom with a finger so that it comes out like a mountain.
For both two- and three-dimensional stretching, it is natural to ask how much a stretchable display can be stretched. In this paper, the extent to which a stretchable display device can be stretched is called stretchability. It is one of the most important performance factors of stretchable displays for all the stakeholders, such as the module makers, set makers, consumers, and testing agencies. Therefore, the measurement of stretchability should be repeatable, reproducible, and cost-effective so that it can be performed by any of the stakeholders and the results of the measurement can be communicated between the stakeholders.
In the case of two-dimensional stretching, the length ratio before and after stretching has been widely utilized to represent stretchability. For example, 10% stretching implies that 10 cm can be stretched up to 11 cm while satisfying the normal operating conditions and durability criteria specified by the manufacturer or standard. However, the ratio of length cannot be directly utilized for three-dimensional stretching.
Calculating three-dimensional stretchability requires the measurement of the surface areas after the stretching. The FEM-based or optical measurement-based methods can be applied to measure or approximate the stretched areas [Citation7]. However, these methods are computationally expensive, and it is often difficult to yield consistent results when the methods are performed by different stakeholders. Therefore, this paper aims to present a simple and practical measurement method of ‘3D stretchability.’ Three-dimensional stretchability depends on the type of stretching and apparatus used in the stretching. In addition, types of three-dimensional stretching are quite diverse. Thus, define a method and mechanism for generating a controlled three-dimensional stretching is necessary. Such a three-dimensional stretching generation should mimic the actual three-dimensional stretching to be used in industries. This paper proposes simple and practical measures of three-dimensional stretchability based on the predefined generation of three-dimensional stretching. It is assumed that stretchable displays have an island-bridge structure [Citation8–18]. The pros and cons of the proposed measures are explained. In addition, the accuracies of the proposed methods are verified by comparing them with the results from FEM-based measurement.
This paper is organized as follows: In Section 2, the stretchability of two-dimensional stretching and the controlled generation of three-dimensional stretching are described. The proposed measures of three-dimensional stretchability are also presented. The performance of the proposed measures is compared in Section 3. Finally, Section 4 concludes this paper.
2. Measurement of stretchability
2.1. Two-dimensional stretchability
Two-dimensional stretching implies that a stretchable display is located on the same plane before and after stretching, and the area size is changed. Such two-dimensional stretching can be caused by a force applied to both or one of the horizontal and vertical directions. It is assumed that the extent of stretching is isotropic when the forces applied in the direction of the horizontal and vertical axis are the same. Two-dimensional stretchability can be measured using an area ratio or length ratio. However, the measurement of length ratio has been broadly used for two-dimensional stretching. It is defined in Equation (1).
(1)
(1) The length of the stretchable display in the direction of the force is measured before and after the maximum stretching that satisfies durability conditions specified by the manufacturer. The advantage of the length ratio is that the measurement method is well-established and simple. However, it cannot be directly utilized for three-dimensional stretching.
2.2. Controlled generation of three-dimensional stretching
Three-dimensional stretchability depends on the type of stretching and apparatus used in the stretching. Thus, identifying a method and mechanism for generating a controlled three-dimensional stretching first so that three-dimensional stretchability can be measured independently by the module manufacturers, final product makers, testing agencies, and/or consumer organizations is essential. Such a three-dimensional stretching generation should mimic the actual three-dimensional stretching to be used in industries. Controlled generation of three-dimensional stretching should result in repeatable and identically reproducible results.
Figure illustrates an example of generating a controlled three-dimensional stretching. Figure represents a cross-sectional view. Figure (a) and (b) denote the positions of the apparatus before and after the three-dimensional stretching, respectively. An external object to generate a three-dimensional stretching is a sphere attached to a cylinder in Figure . The role of the cylinder is for ease of an up and down movement of the sphere. Here, it is assumed that the sphere is moved upward by an electric motor.
Figure 1. Example of controlled generation of three-dimensional stretching (a) before stretching and (b) after stretching.

The fixture guide sits on the top of the stretchable display and remains unchanged during the generation of three-dimensional stretching. it is a thin but rigid plate with a circular opening. However, it is represented as two separated rectangles in the cross-sectional view in Figure . The fixture guide serves to limit the range of three-dimensional stretching. The diameter of the circular hole of the fixture guide is bigger than that of the sphere to generate three-dimensional stretching. The use of the fixture guide can be optional. When the fixture guide is not utilized, the shape of three-dimensional stretching appears less steep. However, the stretchable display may stretch in the same way as lifting the center of a camping tent with a pole. In other words, the entire area of the stretchable display may be stretched. In this paper, 3D stretching can be specified by the radius and curvature of the rounded cylinder, the radius of the fixture guide hole, and the speed of the cylinder. In addition, the durability of the stretched panel may be affected by the shape of the fixture guide and the size of the gap between the fixture guide and the sphere to generate three-dimensional stretching.
2.3. Measures of three-dimensional stretching
In this paper, new measurement methods of three-dimensional stretchability can be explained using the schematic drawing illustrated in Figure . The horizontal line in Figure represents a stretchable display device before stretching. The fixture guide with a circular hole sits on the stretchable display device. In Figure , the grey-shaded circle represents the cross-section of the sphere to generate three-dimensional stretching. The red arc, , represents the boundary of the portion of the sphere moved above the fixture guide to create a controlled three-dimensional stretching. The blue curved line denotes the portion of the stretched display by the sphere. The stretchable display reaches the maximum height predetermined by the manufacturer. It is assumed that the maximum height is less than the radius of the sphere.
Figure 2. Schematic cross-sectional view to explain the proposed measures of three-dimensional stretchability.

The larger circle in Figure denotes the boundary of the cross-section of an imaginary sphere. It is assumed that the imaginary sphere passes through the three points, A’, B’, and the top of the stretched display. The green arc, , represents the boundary of the cross-section of the imaginary sphere above the plane defined by the fixture guide. In Figure , h represents the maximum height.
is the radius of the base of the sphere cap. It is also half the length of the chord corresponding to the red arc.
,
, and
are the radius of the sphere, the circular hole of the fixture guide, and the imaginary sphere, respectively.
is the difference between the radius of the circular hole of the fixture guide and the radius of the sphere. It represents a gap between the fixture and the sphere.
The first measure of the three-dimensional stretchability is defined by Equation (2) using the ratio of the red arc to its corresponding chord
.
(2)
(2) where
and are
are calculated by Equations (3) and (4), respectively.
(3)
(3)
(4)
(4)
in Equation (3) is defined in Figure . In Equation (2), the surface of the stretchable display after stretching exactly matches with the surface of the sphere that generates the stretching. This assumption may deteriorate the accuracy of the calculated stretchability.
An alternative measure of stretchability is defined in Equation (5) using the ratio of the green arc to its corresponding chord
.
(5)
(5)
in Equation (5) can be calculated by substituting
in place of
and
in place of
in Equation (3). Similarly,
in Equation (5) can be determined by substituting
in place of
and
in place of
in Equation (4). The new measure in Equation (5) better mimics the shape of stretching caused by the sphere and the fixture guide. It is likely that the measure in Equation (5) provides better accuracy than the measure in Equation (2). The advantage of the measures based on the length ratio in Equations (2) and (5) is that the two-dimensional stretchability in Equation (1) is also determined based on the length ratio. Thus, values of two and three-dimensional stretchability measures can be compared.
Unlike new measures in Equations (2) and (5), three-dimensional stretchability can be measured based on area ratio. A new measure based on the area ratio can be defined by Equation (6).
(6)
(6)
The nominator inside the parenthesis in Equation (6) represents the area after three-dimensional stretching. More specifically, it is the surface area of the sphere cap in Figure . The sphere cap is a portion of the sphere to generate the controlled three-dimensional stretching. The denominator inside the parenthesis in Equation (6) represents the area before the stretching. It is the base area of the sphere cap. The base of the sphere cap is a circle whose radius is .
The last measure of three-dimensional stretchability is also based on the area ratio. It can be defined in Equation (7) using the imaginary sphere defined in Figure .
(7)
(7) The nominator inside the parenthesis in Equation (7) is the surface area of the sphere cap corresponding to the green arc of the imaginary sphere in Figure . The imaginary sphere is assumed to be cut by the plane of the fixture guide. The denominator inside the parenthesis in Equation (7) is the area of the base of the sphere cap of the imaginary sphere. The base of the sphere cap is a circle whose radius is
.
Unlike the stretchability measures in Equations (2) and (5), new measures in Equations (6) and (7) are defined based on the area ratio.
3. Performance evaluation of three-dimensional stretchability measures
3.1. Reference data from simulation based on FEM
Before stretching, pixels of stretchable display can be represented by squares or rectangles placed on the same plane. A pixel position can be specified by two-dimensional coordinates. After a controlled stretching by the sphere illustrated in Figure , a stretched pixel appears as a quadrilateral in three-dimensional space. Three-dimensional coordinates of the vertices of the stretched pixels are needed to specify the pixel positions. They can be determined by a simulation using FEM (Finite Element Method). The details of the simulation utilized in this paper will be reported separately.
To evaluate the accuracies of the proposed stretchability measures, ground truth or reference data are needed. The reference measures for comparison are determined based on the three-dimensional coordinates of vertices of stretched pixels from the FEM-based simulations. The reference measure based on the length ratio is defined as in Equation (8).
(8)
(8) In Equation (8), the ‘Length of blue curved line’ represents the length after the controlled three-dimensional stretching in Figure . It is determined by the data from the simulation based on FEM. The denominator inside the parenthesis in Equation (8) represents the length,
before the stretching.
in Equation (8) serves as the reference data to evaluate the accuracies of the proposed measures based on the length ratio in Equations (2) and (5).
A new area ratio-based measure of three-dimensional stretchability is defined by Equation (9).
(9)
(9) In Equation (9),
represents the set of stretched pixels. Whether or not it is stretched can be determined by checking the z-coordinate in the three-dimensional coordinates of the vertices of the pixels determined by the simulation.
denotes the index of the pixel location. ‘pixel_area_before(i,j)’ and ‘pixel_areas_after(i,j)’ represents the area of the pixel at the (i,j)th location before and after the three-dimensional stretching, respectively. The shape of the pixel before stretching is square or rectangular. Thus, sum of ‘pixel_area_before(i,j)’ can be calculated by multiplying the number of stretched pixels by the area of a pixel. However, ‘pixel_areas_after(i,j)’ needs to be calculated using the three-dimensional coordinates of the vertices of the quadrilateral determined by the simulation with FEM.
The stretchability measures based on the data from the simulation serve as the reference values of the measures. However, they may not be practical stretchability measures because of the following reasons: Computer simulation based on FEM is computationally expensive. The data required to run the simulation program, such as the Young’s modulus and Poisson ratio, may not be publicly available to all the stakeholders.
3.2. Experimental results of performance evaluations
Even with the controlled stretching shown in Figure , values of three-dimensional stretchability depend on the values of the parameters such as the radius of the sphere, the radius of the circular hole in the fixture guide, and the maximum height. Table lists 21 different combinations of these parameters to cover various three-dimensional stretching cases. For each of the 21 cases, FEM-based simulation is carried out. In addition, the reference measures in Equations (8) and (9) are calculated. In addition, the values of the length ratio measures in Equations (2) and (5) are compared with the values of the measure in Equation (8). Similarly, the values of the area ratio measures in Equations (6) and (7) are compared with the values of the measure in Equation (9).
Table 1. Twenty-one configurations of controlled generation of three-dimensional stretching.
Figure illustrates graphs of the length-based measures, and
, versus the reference measure
in Equation (8). Figure illustrates the graphs of the area-based measures,
and
, versus the reference measure
in Equation (9). All the measures are sorted in ascending order of the reference measures. If the values of the proposed measures match those of reference measures, the graphs will appear as straight lines.
and
defined using an imaginary sphere yielded graphs close to the straight lines whereas
and
generated the graphs far from straight lines.
In addition, an average percent of errors is calculated by Equation (10)
(10)
(10) In Equation (10),
is the number of different configurations of three-dimensional stretching. In this case, N = 21, as listed in Table .
represents the value of the reference measures with the ith configuration.
represents the value of the proposed measures with the ith configuration.
in Equation (8) serves as the reference data for
and
. Similarly,
is utilized as the reference data for
and
.
Figure details the graph of the percent errors of and
versus
. When the values of the measure under evaluation are the same as those of the reference measure, the percent error will have a value of 0. It can be noticed from Figure that
yields quite different error values for the twenty-one cases of three-dimensional stretching. However, the percent errors of
are relatively consistent for all twenty-one cases. The average percent errors for
is 4.5%. Figure illustrates the graph of the percent errors for
and
. The horizontal axis represents
sorted in ascending order.
generates much larger errors than
. The percent errors of
is insensitive for variations in the values of parameters of the three-dimensional stretching. However, the average percent errors for the
is much larger than that of
.
The effects of the gap between the fixture and the sphere on the are examined. Figure explains how changing the values of the gap affects the values of
when the radius of the sphere
and the maximum height are fixed. There are three graphs of
illustrated in Figure . Three graphs in Figure represent the cases of the different maximum heights
respectively. Each graph includes the measurements for three different values of the gap
. The values of the gap are written on the graphs. It can be gleaned from Figure that the value of
decreases as the gap increases when the radius of the sphere and the maximum height are fixed.
Next, the effects of the radius of the sphere are examined with examples where the gap and the maximum heights
are fixed. Figure depicts the values of
when the radius of the sphere changes from 25 to 65 mm. The values of the radius of the sphere
are written on the graphs. The value of
decreases as the radius of the sphere increases when the gap and the maximum height are fixed, as illustrated in Figure .
4. Conclusion
Stretchability is one of the most important performance factors of stretchable displays. The length ratio before and after stretching has been extensively used to represent two-dimensional stretchability. However, the ratio of length cannot be directly applied to three-dimensional stretching. In this paper, a controlled configuration of the generation of three-dimensional stretching is proposed. In addition, new measures of three-dimensional stretchability are introduced. The advantages and disadvantages of the proposed measures are explained. The accuracy of the proposed measures is evaluated using the reference measures determined based on the FEM-based simulation. The length-based measure based on an imaginary sphere yields small and consistent errors in twenty-one cases of three-dimensional stretching utilized in the experiments. It can be a good candidate as a standard measure of three-dimensional stretching.
Acknowledgments
This research was supported by the Material and Component Industrial Future Growth Engine Program (20010744) funded By the Korea Ministry of Trade, Industry & Energy (MOTIE).
Disclosure statement
No potential conflict of interest was reported by the author(s).
Additional information
Funding
Notes on contributors

Choon-Woo Kim
Choon-Woo Kim received a B.S. degree in control and instrumentation engineering from Seoul National University, Korea, in 1983. He received his M.S. and Ph.D. degrees in electrical engineering from Purdue University in 1985 and 1989, respectively. From 1989 to 1994, he was with 3M, St. Paul, MN, U.S.A. In 1994, he joined the Department of Information and Communication Engineering, Inha University, Incheon, Korea. He was an associate editor of the Journal of Electronic Imaging from 2007 to 2019 and the symposium chair for Electronic Imaging in 2016. He is a convenor of IEC TC110 WG13. His research interests include color image processing for digital imaging systems.

Gi Won Kim
Gi Won Kim is an undergraduate student in the Departments of Information and Communication engineering, Inha University, Korea. His research interests include next-generation displays and image quality on stretchable displays.

Gyeong Gyun Kang
Gyeong Gyun Kang is an undergraduate student in the Dept. of Information and Communication Engineering at Inha University, Korea. His research interests are the calibration method and efficiency of next-generation displays.
References
- T. Sekitani, et al., Nature Mat 8 (6), 494–499 (2009).
- Y. Hong, et al., J. Inf. Display 33 (4), 6–38 (2017).
- Y. Lee, et al., Sci Adv 7 (23), (2021). doi:10.1126/sciadv.abg9180.
- J. Hong, et al., J. of SID 25 (3), 194–199 (2017).
- Y. Huang, et al., ACS App. Mat. 11 (51), 48321–48330 (2019).
- H. Sun, Z. Han, and N. Willenbacher, ACS App. Mat 11 (41), 38092–38102 (2019).
- S. Han, J. Shin, S. Choi, et al., IMID 2021 (2021).
- J. Byun, et al., Scienti. Reports 7 (2017). doi:10.1038/srep45328.
- Y. Lee, et al., Chem. Eng. J. 427 (2022). doi:10.1016/j.cej.2021.130858.
- K. Han, et al., ACS App. Elec. Mat 3 (11), 5037–5047 (2021).
- D. Kim, et al., Chem. Eng. J 429 (2022). doi:10.1016/j.cej.2021.132289.
- Y. Lee, et al., Mat. Today 53, 51–57 (2022).
- H. Cho, et al., Mat. Hori. 9 (8), 2053–275 (2022).
- M. Miyakawa, H. Tsuji, and M. Nakata, J. of SID 30 (9), 699–705 (2022).
- Y. Hong, et al., SID Int. Symp. Digest Tech. Papers 49 (1), 483–485 (2018).
- Q. Yang, et al., SID Int. Symp. Digest Tech. Papers 51 (1), 1142–1144 (2020).
- X. Jinxiang, et al., SID Int. Symp. Digest Tech. Papers 52 (11), 2092–2103 (2022).
- C. Wang, et al., SID Int. Symp. Digest Tech. Papers 53 (1), 521–523 (2022).
- J. Rogers, T. Someya, and Y. Huang, Science 327 (5973), 1603–1607 (2010).
- M. Kaltenbrunner, et al., Nature 499 (7459), 458–463 (2013).
- T. Sekitani, et al., Nature Mat 9 (12), 1015–1022 (2010).
- Y. Van De Burgt, et al., Nature Mat 16 (4), 414–418 (2017).
- S. Park, M. Vosguerichian, and Z. Bao, Nanoscale 5 (5), 1727–1752 (2013).
- C. Larson, et al., Science 351 (6277), 1071–1074(2016).
- M. White, et al., Nature Pho 7 (10), 811–816 (2013).
- M. Park, et al., Nature Nano 7 (12), 803–809 (2012).
- Y. Kim, et al., Nature 500 (7460), 59–63 (2013).
- N. Matuhisa, et al., Nature Comm 6 (2015). doi:10.1038/ncomms8461.
- M. Lee, et al., Nano Letters 13 (6), 2814–2821 (2013).
- D. Kim, and J. Rogers, Adv. Mat 20 (24), 4887–4892 (2008).
- S. Benight, et al., Progress in Ploy. Sci 38 (12), 1961–1977 (2013).
- B. Wang, et al., Chem. Review 118 (11), 5690–5754 (2018).
- J. Boley, et al., Adv. Func. Mat 24 (23), 3501–3507 (2014).
- T. Cheng, et al., Adv. Mat 27 (22), 3349–3376 (2015).
- C. Yan, et al., ACS Nano 8 (1), 316–322 (2014).
- S. Lacour, et al., Proceed. of IEEE 93 (8), 1459–1467 (2005). J. Koo, et al., Adv. Fun. Mat., 28 (35) (2018).
- J. Yoon, H. Kim, and Y. Hong, Inf. Display 38 (6), 14–18 (2022).
- H. Cho, et al., J. Inf. Display 21 (1), 41–47 (2020).
- S. Kim, et al., SID Int. Symp. Digest Tech. Papers 50 (2), 1194–1197 (2019).
- P. Wang, et al., SID Int. Symp. Digest Tech. Papers 53 (1), 524–525 (2022).
- Y. Hong, et al., SID Int. Symp. Digest Tech. Papers 48 (1), 253–256 (2017).