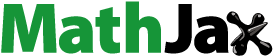
Abstract
The field of III-nitride (InGaN) nanowire micro light-emitting diode (µ-LED) displays is rapidly expanding and holds great promise, thanks to their chemical stability and outstanding performance across the entire visible spectrum. Notably, III-nitride (InGaN) nanowires, free from compositional substitutions, dislocations, and piezoelectric polarization effects associated with lateral strain relaxation with large surface-to-bulk-volume ratio, are advantage-missing in traditional planar counterparts. This comprehensive overview examines the potential landscape, associated challenges, strategies to overcome them, and opportunities for the development of advanced µ-LED displays with vibrant and accurate color representation, contributing to the advancement of next-generation display technologies. This study also covers the current obstacles faced by III-nitride (InGaN) nanowire-µ-LED displays and possible solutions to address them.
1. Introduction
Advances in display technology using micro light-emitting diodes (µ-LEDs) are continually being incorporated into modern electronic devices, necessitating high-performance smart devices for future device applications, ranging from smartphones to large-scale video walls, significantly surging in recent years and continuously growing unabated [Citation1–3]. Besides, µ-LEDs, a state-of-the-art emissive display design, are more compact (in the sub-submicron range) and with fewer optical components compared to backlit, and such favorable features have generated much interest [Citation4]. In contrast to backlit displays that rely on combined colored LED light, color filters, polarizers, and liquid crystals to create the display unit (as illustrated in Figure a), µ-LED-based emissive displays offer a much simpler system design (as depicted in Figure b). These displays directly emit light, eliminating the need for intricate layers and components, resulting in a streamlined and efficient display technology. These displays utilize red, green, blue, and occasionally white LEDs to project light directly at the viewer. Additionally, µ-LEDs outperform conventional LEDs in current spreading, self-heating, and light extraction efficiency [Citation5,Citation6]. Microdisplays, designed for a maximum brightness of 105 nits and close viewing distances, typically have fewer pixels and narrower gaps between them. However, the challenge of achieving high-pixel-density displays remains unresolved and requires further research due to pixel mode limitations [Citation7]. Given the increasing demands, researchers and engineers actively explore innovative materials and device structures to overcome their limitations. Technological implementation of emission displays began primarily with organic LED (OLED) systems due to their ease of manufacture and potential cost savings [Citation8,Citation9]. Although OLEDs have advantages for displays, they have not matched the performance and durability of their inorganic LED counterparts [Citation4,Citation10,Citation11]. Meanwhile, high-pixel density OLEDs can be achieved using evaporation and shadow masking techniques, allowing them to be employed in bright displays [Citation12,Citation13]. Table depicts the various performance matrices of LCD, OLED, and, µ-LED systems.
Figure 1. Schematic diagram of the cross-sectional view model of (a) backlit (b) emissive displays (Figures reproduced with permission from [Citation4] Copyright © 2019, John Wiley and Sons) and (c) band–gap energy versus lattice constants profile of III–nitrides (Al, In, Ga)-N (inside the dashed lines denotes that lattice-matched points of InxGa1−xN/InyAl1−yN that corresponds to blue, green and red emission wavelength, respectively). Adopted from Ref. [Citation29]. Reproduced under the terms of an open-access Creative Commons CC BY 4.0 license.
![Figure 1. Schematic diagram of the cross-sectional view model of (a) backlit (b) emissive displays (Figures reproduced with permission from [Citation4] Copyright © 2019, John Wiley and Sons) and (c) band–gap energy versus lattice constants profile of III–nitrides (Al, In, Ga)-N (inside the dashed lines denotes that lattice-matched points of InxGa1−xN/InyAl1−yN that corresponds to blue, green and red emission wavelength, respectively). Adopted from Ref. [Citation29]. Reproduced under the terms of an open-access Creative Commons CC BY 4.0 license.](/cms/asset/c0d01663-77b1-4619-abc5-6e133c491c90/tjid_a_2282937_f0001_oc.jpg)
Table 1. Performance matrix of LCD, OLED, and µ-LED systems [Citation14].
Their promising optoelectronic features and tunable capability from far UV to near-infrared- III-nitride semiconductors, shown in Figure c, have emerged as the workhorses of LEDs and lasers, laying the foundation for modern solid-state lighting (SSL) [Citation15–19]. Moreover, these numerous advantages over their organic counterparts, such as high radiative recombination rates, long-term stability, and reliability, make them particularly appealing for real-world applications, especially in the realm of high-pixel full-color emissive displays [Citation20–22]. The extensive utilization of III-nitride semiconductors in lighting and display applications, especially highly efficient visible spectrum InGaN-based LEDs, has driven significant progress in research and development [Citation23–26]. Key innovations to achieve these objectives are large-surface, low-footprint, high-performance inexpensive substrates, and non-polar GaN, which enable integration into on-chip structures [Citation27,Citation28].
The pioneering demonstration of gallium nitride (GaN) in 1969 by Maruska and Tietjen using the hydride vapor-phase epitaxy (HVPE) approach at the Radio Corporation of America Laboratories in the United States opened up new avenues [Citation30]. Subsequently, the GaN growth methods, including molecular beam epitaxy (MBE) [Citation31–42] and metalorganic chemical vapor deposition (MOCVD), became integral in the production [Citation24,Citation28,Citation43–52]. The evolution of III-nitride LEDs can be traced back to the 1990s when researchers demonstrated efficient blue LEDs [Citation7]. Since then, substantial efforts have been devoted to research and development of GaN-based nanostructures for advancements in optoelectronic device applications [Citation53,Citation54]. The significance of this progress was further acknowledged in 2014 when Professors Isamu Akasaki, Hiroshi Amano, and Shuji Nakamura were awarded the Nobel Prize for their discovery of high-brightness GaN-based blue light-emitting diodes (LEDs) [Citation55]. Seemingly, considerable progress has been made in developing GaN-based blue and near-UV LEDs and lasers. However, their utilization in deep visible/near-infrared photonics, deep UV, and quantum optoelectronics has been restricted due to several challenges. These challenges include the presence of numerous defects, dislocations, and disorders in the materials, a significant polarization field, and inefficiencies in p-type conduction [Citation56–59].
One promising solution lies in utilizing nanowire (NW) arrays, which possess exceptional optical properties and a controllable microstructure. By manipulating physical properties like diameter, height, and pitch, NW arrays can effectively regulate and fascinate incident light. The development of III-nitride (InGaN) NW µ-LEDs represents a significant advancement in display technology, offering unparalleled brightness, efficiency, and miniaturization [Citation60,Citation61]. Moreover, elastic strain can be applied to enhance material properties and functionality, including physical, electrical, and optical properties. Furthermore, the high surface charge and saturation slip velocity of these LEDs, resulting from spontaneous and piezoelectric polarization fields, make them suitable for high-power devices operating at high voltage and temperature, surpassing traditional planar counterparts made from III-nitrides [Citation62]. By leveraging the inherent properties of InGaN NWs, these LEDs can convert electrical energy into light with remarkable efficiency, minimizing power consumption and extending battery life in portable devices. This energy-saving feature not benefits end-users and contributes to a greener and more sustainable future. Moreover, the miniaturization potential of the InGaN NW micro LED opens up new possibilities in various applications. However, structural flaws such as lattice mismatches between the substrate and epilayers, as well as within the epilayers themselves, can develop various defects, including threading dislocations [Citation63,Citation64], stacking faults [Citation63], point defects [Citation65], V-pits [Citation66], misfit dislocations [Citation67,Citation68], and trench defects [Citation46], and pose challenges that negatively impact performance. Achieving high-quality InGaN layers poses a challenge due to the immiscibility of InGaN alloys, resulting in frequent composition fluctuation and phase separation [Citation41,Citation69–71]. The addition of indium adatom to InGaN layers worsens this issue, making it even more challenging to achieve long-wavelength emission [Citation72]. Typically, InGaN quantum wells (QWs) are the most convenient emitters for short-wavelength emissions due to their low indium (15%–17%) requirements for blue emission [Citation45]. When transitioning to higher levels of emission, such as green and red (around >22%), the required proportion of indium increases significantly, dramatically dropping quantum efficiency (QE) within the QWs. This phenomenon, known as the green gap, can be attributed to crystal quality degradation and the quantum-confined Stark effect (QCSE) [Citation69]. Enhancements are required to bolster the performance of InGaN LEDs in the extended wavelength range.
To address these challenges and advance the field, a comprehensive study is needed to understand the causes of the ‘green gap’ and its related issues. Investments in research and development in this sector highlight the significance of this field in consumer markets [Citation73]. The integration of InGaN NW µ-LEDs into compact devices, wearable electronics, augmented reality (AR) and virtual reality (VR) headsets, and medical implants can bring about lifelike images and seamless merging of virtual and real-world data [Citation19,Citation41,Citation74]. The constant evolution of display technology and the InGaN NW µ-LED breakthrough will shape the future. Further optimization and research aim to reduce defect concentrations in (In)GaN-based NW semiconductors, meeting the demand for full-spectrum RGB light output, smaller footprints, and more efficient light emission [Citation75–81]. Amidst this landscape, a groundbreaking innovation has emerged—the III nitrides (InGaN) NW µ-LED —which can revolutionize industries ranging from consumer electronics to healthcare and others [Citation82].
This renewed interest is evident in the increasing number of patents, publications, and studies focused on the performance of GaN-based micro-displays to achieve full (∼100%) luminance efficiency in submicron displays. To achieve this efficiency level, it is necessary to establish a reliable method for producing µ-LEDs with optimum efficiency and consistent optical emission spectra. This requires a comprehensive understanding of how to effectively localize and arrange different atomic species while fabricating the wire and its multiple layers. The objective was to investigate the elements that influence the containment or spreading of a well as the structure develops. Numerous studies on III-nitride NW optoelectronic devices, including micro LEDs [Citation83–85], lasers [Citation86,Citation87], photodetectors [Citation36,Citation88], solar cells [Citation89–91], and solar fuel devices [Citation92,Citation93], have been developed in previous works. In this overview, we synthesize the advancements and challenges in InGaN-based LED displays, fabrication techniques, optical and electrical characteristics, and display applications. Section 1 briefly introduces the topic. Section 2 explores the growth mechanism of III-nitride NWs, which serves as a widely adopted fabrication process. Section 3 focuses on the application of III-nitride NWs in µ-LEDs and highlights the latest breakthroughs achieved in developing NW-based LEDs operating within the visible spectral regions. Section 4 concludes with a summary of the findings and offers insights into prospects in this field.
2. III-Nitride nanowires- growth mechanism
This section aims to explore different commonly employed methods for developing III-nitride NWs. Our focus is on the fundamental core characteristics and recent developments in the NW-in-structure of III-nitride, encompassing polarity, surface charge properties, and recent advancements in dopant coordination.
2.1 Development of III-nitride NWs
III-nitride NW development can be categorized into two main approaches: top down and bottom up [Citation94,Citation95]. Typically, the top-down strategy involves nanoscale designs followed by an etching procedure. To mitigate surface damage and flaws, wet chemical treatment, post-etch annealing, and/or annealing are frequently employed. On the other hand, the bottom-up approach serves as the foundation for a significant portion of research on III-nitride NWs, offering more freedom in managing device topologies [Citation49,Citation94]. The growth of III-nitride NWs using a bottom-up approach underlines the concept of vapor–liquid-solid (or) diffusion-driven growth, which involves several key steps to achieve the desired properties and performance.
2.1.1. Fabrication on gaN-Based nanowires: a top-down approach
III-nitride materials, characterized by their wurtzite crystal structures, exhibit a natural polarization field stemming from the absence of inversion symmetry. This inherent polarization effect is compounded by the lattice mismatch between the InGaN QW and GaN barrier layers. Consequently, piezoelectric polarization is induced, a phenomenon often harnessed to achieve a spectral blue shift by nanostructuring the InGaN/GaN QWs within a grown LED wafer. In many display applications, especially those requiring high-resolution augmented displays, miniaturizing LED chip sizes to the micrometer or even submicrometer scale is imperative [Citation96,Citation97]. Despite the significantly lower surface recombination velocity in InGaN-based materials than other III–V semiconductors, microscale InGaN LEDs are susceptible to the impact of Shockley–Read–Hall (SRH) recombination at surface defects [Citation5,Citation98,Citation99]. Top-down fabrication is a prominent technique to achieve monolithic multicolor emission in display technology, particularly utilizing GaN-based nanowires [Citation100]. The typical nanostructuring for LEDs or micro LEDs involves transferring a specific pattern using nanolithography techniques (such as electron-beam lithography or nanosphere lithography), followed by inductively coupled plasma dry etching, to expose the sidewalls of the quantum wells, often accompanied by surface passivation [Citation101]. While manufacturing μLEDs, processes like etching generate surface defects, impurities, and dangling bonds, which act as non-radiative recombination centers. This process allows for strain relaxation, reducing polarization fields and minimizing the extent of QCSE [Citation102,Citation103]. The resultant QW band profile becomes less triangular, leading to higher effective bandgap energy, resulting in blue-shifted emission spectra and increased IQE due to enhanced electron–hole wavefunction overlap [Citation104]. Moreover, as nanostructure sizes decrease, a larger portion of the nanostructures experiences strong strain relaxation, causing a greater spectral blue shift [Citation105,Citation106]. Consequently, the EQE of blue InGaN LEDs decreases significantly as the lateral dimension shrinks, underscoring the need to minimize surface defects during the production of micro or sub-micrometer-sized LEDs and protect the surface with a robust passivation layer [Citation96,Citation97,Citation99]. For instance, Smith et al. [Citation99], observed a reduced external quantum efficiency (EQE) of blue InGaN LEDs, from around 10% at a lateral dimension of 10 µm to approximately 2%–3% at 1 µm. The sidewalls of InGaN/GaN MQW nanorods exhibit various structural imperfections, including surface dangling bonds, defects from strain relaxation, and lingering damage from dry etching. Many research reports have shown that surface passivation can effectively mitigate these defect states, enhancing EQE. However, conventional passivation methods, such as chemical vapor deposition or atomic layer deposition (ALD), expose the nanoscale LEDs to thermal energy, plasma, and atomic reactions, potentially leading to structural damage on delicate sidewall surfaces. The combined polarization charges result in the QCSE, decreasing the effective bandgap energy and hence, a blue-shifted emission spectrum. This observation of size-dependent strain relaxation in the quantum wells led to the proposition that nanostructures patterned using techniques like nanosphere lithography could be employed to attain monolithic multicolor emission [Citation103,Citation107]. The recent study of the interaction between the GaN surface and the sidewall passivation layer was thoroughly explored using a range of techniques, including dry etching, wet etching, plasma SiO2, and sol–gel SiO2 (Mihyang Sheen et al.). Figure illustrates the progression of the morphology and atomic structure of the sidewall in the multiple quantum well (MQW) region after each fabrication step, elucidated through high-angle annular dark-field scanning transmission electron microscopy (HAADF-STEM) imaging and respective schematic representation of atomic structure formation. In dry etching of the epi-layer, the nanorod assumed a trapezoidal shape due to differences in impact energy on the surface. The white arrows emphasize the sidewall’s amorphous region, resulting from successive ion bombardments. In contrast, after KOH wet etching, the nanorod exhibited an inverted trapezoidal shape due to the slower etch rate of p-GaN compared to n-GaN. Upon depositioning the SiO2 layer through plasma-enhanced ALD, the plasma introduced point defects like nitrogen vacancies, nitrogen interstitials (Ni), nitrogen split interstitials (N–N) N, and vacancies of Ga complexes, respectively. This amorphized the nanorod surface observed across the entire GaN nanorod. Notably, this amorphous region was more pronounced on the semi-polar facet of the InGaN QWs, likely due to the higher density of dangling bonds. As the SiO2 layer thickness increased from 2 nm to 60 nm, the plasma-induced amorphization penetrated to a depth of 4 nm at the InGaN QWs. Conversely, when utilizing sol–gel SiO2 deposition on the nanorods, no amorphous region was observed at the interface, in addition to the deposited SiO2. Challenges such as plasma-induced damage, achieving current injection without shorting the device, and strain relaxation in long-wavelength-emitting wafers are addressed during top-down fabrication. A novel approach involves inducing strain in quantum wells through specific nanostructure configurations, resulting in spectral red-shifting. By strategically fabricating nanostructures above the electron-blocking layer and multiple quantum wells, strain can be induced, allowing for longer-wavelength emission without significantly altering the indium content in the quantum wells. This approach shows promise in achieving high IQE and broadening emission spectra, essential for realizing monolithic RGB displays via top-down nanostructuring.
Figure 2. STEM-HAAF images illustrating the side wall appearance of InGaN MQW with different fabrication techniques: (a) dry etch, (b) wet etch, (c) sol–gel SiO2 deposition, and plasma-enhanced ALD SiO2 deposition of thicknesses measuring (d) 2 nm and (e) 60 nm. Figures reproduced with permission from [Citation84]. Copyright © 2022.
![Figure 2. STEM-HAAF images illustrating the side wall appearance of InGaN MQW with different fabrication techniques: (a) dry etch, (b) wet etch, (c) sol–gel SiO2 deposition, and plasma-enhanced ALD SiO2 deposition of thicknesses measuring (d) 2 nm and (e) 60 nm. Figures reproduced with permission from [Citation84]. Copyright © 2022.](/cms/asset/d243807b-26cb-4b87-9ef7-b0af322084a7/tjid_a_2282937_f0002_oc.jpg)
2.1.2. Bottom-up approach
2.1.2.1 Self-organized growth of GaN-based NWs
Catalyst-mediated growth of III-nitride NWs: GaN nanorods exhibit self-organized formation through the combined mechanisms of vapor–liquid-solid (VLS) and vapor–solid (VS) growth principles, as demonstrated in Figure . Vapor–liquid–solid (VLS) growth, introduced by Wagner and Ellis [Citation108], later on, is a commonly used technique for synthesizing III-nitride NWs [Citation108]. Johar et al. made an initial announcement regarding the successful fabrication of GaN/InGaN multi-quantum well (MQW) co-axial NWs on an n-type Si (111) substrate [Citation48]. This significant achievement was undertaken to enable the study of ultrafast carrier dynamics. In this method, III-nitride NW growth is initiated by a metal catalyst, typically Au [Citation109] or Ni [Citation110], and a mixture of both [Citation111], as well as Ta, in the presence of nitrogen and a gaseous mixture [Citation112]. The growth process involves adatom adsorption, diffusion, and precipitation. Initially, the metal catalyst serves as a nucleation site for III-nitride NW growth. As gaseous precursors contact with the heated substrate, they decompose and react with the catalyst, forming a liquid alloy droplet. Concurrently, nitrogen reacts with the absorbed material (Al, Ga, and In) in the catalyst, forming III-nitride NWs. These NWs grow vertically from the liquid alloy droplets with the Si (111), with their size and density determined by factors such as growth temperature, precursor flow rates, and catalyst size [Citation109]. Previous studies on materials such as GaAs, Si, and GaN claimed that catalyst patterning can effectively facilitate the controlled growth of nanorods through catalyst-induced mechanisms [Citation111,Citation113]. The lateral patterning of the metal catalyst, fashioned via lithography, regulates the development of GaN nanorods. Nevertheless, certain limitations still need to be addressed, specifically regarding defining the orientation and quantity of GaN nanorods produced on a single metal catalyst site [Citation111,Citation114]. Despite VLS’s capability to produce GaN nanorods, their crystal orientations exhibit considerable variations, leading to tendencies of tilting, twisting, intersecting, and branching during their growth process. Focus ion beam etching has been demonstrated as an effective method for contact preparation on VLS-grown single GaN nanorod-based LEDs [Citation115]. Nevertheless, this proposed method for a GaN NW LED may not be feasible for large-scale applications, as variations in the height and orientation of GaN nanorods pose challenges during device processing for solid-state lighting applications.
Catalyst-free self-assembled III-nitride NW growth: Catalysts are commonly utilized to overcome the high activation energy barrier for NW nucleation and growth. However, the presence of catalysts can introduce impurities and defects into the NW structure, which can adversely affect their properties and performance. To promote catalyst-free growth, careful control of the growth parameters is crucial and discussed to realize the formation of III-nitride NWs [Citation116]. The conceptualization of self-assembled GaN nanorods began at MBE, where various groups found that the V/III ratio was a crucial factor [Citation116,Citation118–120]. The choice of substrate, growth temperature, precursor composition, and gas flow rates play vital roles in determining the NW morphology, crystal structure, and optical properties. Figure presents the variance in developing GaN nanostructures in SEM images under N-rich and Ga-rich environments. This study found that a high V/III ratio promotes GaN nanorod growth, whereas a low V/III ratio (Ga-rich) promotes film growth. It was discovered that a high V/III ratio controls the atomic density and suppresses atomic site aggregation by reducing the diffusion length of Ga atoms in the N-rich state [Citation118]. Under the specified growth circumstances, the AlN buffer layer is critical for the regular formation of the nanorods and matrix layers [Citation33]. Additionally, surface treatments and the introduction of suitable growth templates can further enhance the NW growth and alignment. For instance, Jang et al. [Citation117] studied temperature-based self-assembly growth of GaN NW formation on Au-Si (111) using the MOCVD process. This study demonstrated that the density of the NWs increased as the growth temperature increased up to 950°C. After which, it decreased as the growth temperature increased further, as shown in Figure . This tendency was attributed to enhanced adatom evaporation at high temperatures, which caused the growth process to reverse direction. The diameter of the grown GaN NWs ranged from 80 nm to 250 nm at a growth temperature of 950°C, with an average length of 3 mm. Bertness et al. [Citation33] demonstrated that the NW density increased with an increase in the AlN buffer layer thickness from 60 nm to 80 nm–100 nm on Si (111), as reflected in Figure . The easy plastic relaxation of GaN is attributed to the granular structure of the thin AlN buffer layer. This finding suggests that two essential conditions for NW formation are the three-dimensional islanding of GaN and its subsequent plastic strain relaxation [Citation121], which also regulate the growth orientation [Citation122]. Similarly, the importance of buffer layer thickness has been recognized when using sapphire as a substrate [Citation123]. Lande et al., for instance, found that the initial formation of GaN nano-islands on AlN/Si (111) is fully relaxed, providing critical fuel for the growth of NWs [Citation32,Citation121]. Consonni et al. [Citation124] conducted a detailed investigation using a comprehensive surface-free energy model to elucidate the transformation of epitaxial GaN growth.
Figure 3. Schematic representation of VLS metal catalyst assisted III-nitride NW growth sequence process.

Figure 4. SEM images of (a) N-rich and (b) Ga-rich flux conditions, respectively. Figures reprinted with permission from Ref. [Citation116], Copyright © 2016, Elsevier.
![Figure 4. SEM images of (a) N-rich and (b) Ga-rich flux conditions, respectively. Figures reprinted with permission from Ref. [Citation116], Copyright © 2016, Elsevier.](/cms/asset/f44d5f37-b69c-4dbf-9a4b-9f0da34a40e3/tjid_a_2282937_f0004_ob.jpg)
Figure 5. Tilt-view FE-SEM images of GaN NWs grown on Au-Si (111) at growth temperatures of (a) 800, (b) 850, (c) 900, (d) 950, and (e) 1000 °C. Figures reprinted with permission from Ref. [Citation117], Copyright © 2016, IOP Publishing, Ltd.
![Figure 5. Tilt-view FE-SEM images of GaN NWs grown on Au-Si (111) at growth temperatures of (a) 800, (b) 850, (c) 900, (d) 950, and (e) 1000 °C. Figures reprinted with permission from Ref. [Citation117], Copyright © 2016, IOP Publishing, Ltd.](/cms/asset/e9d16fee-7d10-4a7f-8089-9a5f7a128ca3/tjid_a_2282937_f0005_ob.jpg)
Figure 6. FE-SEM characteristic images GaN NWs (a) AlN buffer layer thickness 60 nm, (wire density 5 numbers/mm2) (b) AlN buffer layer thickness 80 nm–100 nm, (wire density 160 numbers/mm2). Figures reproduced with permission from Ref. [Citation33], Copyright © 2016, Elsevier.
![Figure 6. FE-SEM characteristic images GaN NWs (a) AlN buffer layer thickness 60 nm, (wire density 5 numbers/mm2) (b) AlN buffer layer thickness 80 nm–100 nm, (wire density 160 numbers/mm2). Figures reproduced with permission from Ref. [Citation33], Copyright © 2016, Elsevier.](/cms/asset/b68c485b-e9fd-4504-9f28-879a4a3cf306/tjid_a_2282937_f0006_ob.jpg)
They focused on the evolution from nanotruncated pyramidal structures to vertically oriented nanorods, combining in situ RHEED measurements with ex situ HRTEM imaging. During nucleation, they observed a series of sequential shape changes, starting from spherical caps and progressing to truncated and finally full-pyramid-shaped islands. Based on their discoveries, it has the islands can decrease their overall free energy per unit volume by elastically accommodating the strain resulting from lattice mismatches with newly developed facets. Upon reaching a risky size, a misfit dislocation initiates at the edges of the entire pyramids, facilitating plastic relaxation. This misfit dislocation effectively reduces the lattice-mismatch-induced strain near the center of a pyramid. Once this condition is achieved within a complete pyramid, the transformation to GaN nanorods takes place.
Jalena et al. [Citation118] conducted a study on the spontaneous development of GaN NWs on Si(111) using MBE and demonstrated that the formation of nanocolumns on Ga droplet-patterned surfaces does not require any seeding or catalyst on the substrate surface. The presence of Ga droplets, however, acts as a reservoir, providing Ga ad-atoms to nearby nucleation sites and promoting the formation of nanocolumns. This suggests a kinetic mechanism where the migration of Ga atoms toward the nanocolumn tip is crucial in nucleation, as shown in Figure . The lattice mismatch of the substrate stimulates nucleation, while an excess of nitrogen slows down the lateral growth rate, preventing island coalescence. The nanocolumns exhibit continuous growth as they assimilate Ga directly onto their upper regions and undergo Ga diffusion along their sidewalls, extending up to the apex. Remarkably, throughout this growth journey, the nanocolumns maintain their initial diameter, owing to the exceptionally high diffusion length of metal ad-atoms along the sidewalls of the columns [Citation125]. However, an increase in the Ga flux can lead to Ga accumulation at the topmost region of the nanocolumns, resulting in an enhanced lateral growth rate and an increase in the diameter of the nanocolumn top. This effect can eventually cause the nanocolumns to merge in their upper regions. Another approach is diffusion-induced growth, which exhibits dense diffusion on the sidewalls promoting the growth of thin rods with diameters ranging from 10 to 80 nm, but adsorption near the rod tip is only relevant in larger rods, resulting in short thick nanorods and thin elongated nanorods with high aspect ratio GaN nanorod aggregates [Citation125]. Bertness et al. [Citation126] investigated the nucleation of GaN growth on Si(111) using MBE. Their observations revealed that the nucleation density reaches saturation levels within the initial 30 min of growth, forming a high density of nanorods. However, as the deposition time is extended, the nanorod density decreases due to the coalescence of closely spaced nanorods resulting from lateral growth. To analyze the growth dynamics, statistical measurements, including the diameter, length, and volume of the GaN nanorods, were calculated over time theoretically based on a model that represents Si NW MBE growth. According to Foxon et al. [Citation42], the migration-enhanced epitaxy (MEE) mechanism enhances Ga diffusivity, even in robust N-atmospheric conditions, which restricts lateral growth and promotes growth direction vertically. Moreover, the diverse morphology of the nanorods causes notable disparities in the arrival rates of molecules on their top and sidewalls. As a consequence, there are discernible variations in the growth rate, even when diffusion is not considered a contributing factor [Citation127]. In Figure , very lengthy oblique features may be seen, likely reflecting the direction of Ga or N intake. Without rotation, growth is not fully reduced, but it appears to be a significant component [Citation42]. Koester et al. described an MOVPE approach for generating GaN nano or microwires that depended on the in-situ growth of a thin SiNx layer before NW epitaxy. Si doping has a considerable impact on GaN nanorod formation during MOVPE at high SiH4 levels. They determined that a high silane flow promotes growth in vertical directionally.
Figure 7. The typical nucleation approach. Smaller islands could face extinction as Ga diffuses toward other stable nuclei. (b) Illustration of the production of nanocolumns from stable nuclei revealing the direct integration of impinging Ga flux (j(L)) and substrate Ga diffusion (j(D)) into the nucleation base of the building block, extending to its apex. By multiplying the average diffusion length of Ga adatoms by two, the average distance between nanocolumns is calculated, where dCR denoted that the average critical distance signifies the typical proximity from which Ga adatoms can access the base of the nanocolumn. This distance is notably influenced by the growth temperature. Figures reproduced with permission from Ref. [Citation118] Copyright © 2008, Elsevier.
![Figure 7. The typical nucleation approach. Smaller islands could face extinction as Ga diffuses toward other stable nuclei. (b) Illustration of the production of nanocolumns from stable nuclei revealing the direct integration of impinging Ga flux (j(L)) and substrate Ga diffusion (j(D)) into the nucleation base of the building block, extending to its apex. By multiplying the average diffusion length of Ga adatoms by two, the average distance between nanocolumns is calculated, where dCR denoted that the average critical distance signifies the typical proximity from which Ga adatoms can access the base of the nanocolumn. This distance is notably influenced by the growth temperature. Figures reproduced with permission from Ref. [Citation118] Copyright © 2008, Elsevier.](/cms/asset/abc0e575-aafe-44d9-a807-029728ed29de/tjid_a_2282937_f0007_oc.jpg)
Figure 8. SEM comparison of GaN NWs, (a) with rotation (20 rpm) and (b) without rotation, using MBE. Figures reproduced with permission from Ref. [Citation42], Copyright © 2009, Elsevier.
![Figure 8. SEM comparison of GaN NWs, (a) with rotation (20 rpm) and (b) without rotation, using MBE. Figures reproduced with permission from Ref. [Citation42], Copyright © 2009, Elsevier.](/cms/asset/ef37eb0b-aa40-4e87-9ac5-f3c502177d6f/tjid_a_2282937_f0008_ob.jpg)
2.1.2. Selective area growth (SAG)
Owing to the applicant’s suitability, manufacturing of intricate building blocks with appropriate architectural designs based on the homogenous size and position of the template-assisted plane, as well as customized nanopillars, nanosheets, and nanofins, is made possible by SAG, as illustrated in Figure . This approach involves patterning that permits NW creation only where it is required and preventing it elsewhere. By carefully designing the mask pattern, may generate NWs in various forms as positioned precisely, enabling their incorporation into particular device topologies with improved functionality. This degree of control is crucial for applications like light-emitting diodes (LEDs), lasers, and photodetectors where NW positioning and alignment directly influence device performance. This tunability is highly desired for developing full-color displays, white-light generation, and other cutting-edge lighting technologies. For instance, in metal–organic vapor phase epitaxy (MOVPE) and high vacuum phase epitaxy (HVPE), SiO2 [Citation129] and metal-based films have been utilized as SAG masks, respectively. A homogeneous array of GaN nanorods was successfully fashioned by employing SAG and MOVPE on substrates with SiNx mask patterns. The use of triethyl gallium in MO-MBE and gas-source MBE has been extensively investigated as SAG demonstrations. However, achieving SAG through conventional rf-MBE has proven challenging. Although methods for SAG of nanocolumns using nitride Al nanodot patterns have been developed in rf-MBE, the control over nanocolumn shape was inadequate due to the distortion of the Al patterns before nitridation. Another approach, the Ti-mask SAG GaN method, utilizes a stable Ti nano-mask pattern to achieve precise control over the nanocolumns’s size and shape. For example, Ra et al. [Citation130] demonstrated the utilization of InGaN/AlGaN quantum dots (QDs) as the active medium in the selective-area epitaxy of GaN photonic crystals using plasma-assisted MBE. The process involved employing a thin Ti layer as a growth mask on a GaN/sapphire substrate and fabricating various nanoscale designs on the Ti mask using e-beam lithography, as depicted in Figure a. Subsequently, the growth of NWs occurred on the patterned substrate, consisting of n-GaN (400 nm), InGaN/AlGaN multiple QDs (10 pairs), and p-GaN (80 nm) on the top, as shown in Figure b. NW growth was confined to the mask-free hole pattern region, while the Ti mask region remained unaffected by epitaxial growth. Consequently, the apertures in the Ti mask precisely controlled the size and morphology of the GaN photonic crystals. By incorporating AlGaN barriers into the active zone instead of GaN barriers, the Al adatom’s shorter migration length compared to Ga and In adatoms enabled the creation of core–shell-like nanostructures with an AlGaN shell enclosing the InGaN QD active region.
Figure 9. Schematic illustration of SAG growth mechanism. Figures reproduced with permission from Ref. [Citation128], Copyright © 2009, Elsevier.
![Figure 9. Schematic illustration of SAG growth mechanism. Figures reproduced with permission from Ref. [Citation128], Copyright © 2009, Elsevier.](/cms/asset/8f1eeaae-3a75-4e85-a1d6-a171627cc2c0/tjid_a_2282937_f0009_oc.jpg)
Figure 10. (a) schematic representation of the hole pattern on the Ti mask layer for SAE, followed by various structural patterns and corresponding SEM images of nanoscale patterned crystals; (b) schematic representation of InGaN/AlGaN core-shell NW array on patterned substrate and corresponding PL characteristics profile (with comparison without the shell); (c) top-angle SEM images of nanoscale patterned crystals; (d) SEM image at 45° tilt-view of the triangular lattice photonic crystals (250 nm). Figures reproduced with permission from Ref. [Citation130], Copyright © 2017, John Wiley and Sons.
![Figure 10. (a) schematic representation of the hole pattern on the Ti mask layer for SAE, followed by various structural patterns and corresponding SEM images of nanoscale patterned crystals; (b) schematic representation of InGaN/AlGaN core-shell NW array on patterned substrate and corresponding PL characteristics profile (with comparison without the shell); (c) top-angle SEM images of nanoscale patterned crystals; (d) SEM image at 45° tilt-view of the triangular lattice photonic crystals (250 nm). Figures reproduced with permission from Ref. [Citation130], Copyright © 2017, John Wiley and Sons.](/cms/asset/535d6d59-db1e-4527-a21f-aab7a51b059a/tjid_a_2282937_f0010_oc.jpg)
Extensive research on the spontaneous production of III-nitride NWs on silicon (Si) into N-rich environments by MBE suggests the presence of a thin amorphous layer amongst the substrate and the origin of the NW [Citation38,Citation131]. A thorough study explaining the self-induced creation mechanism of GaN NWs grown on SixNx amorphous interlayers was reported by Consonni et al. [Citation132]. They accomplished this using an MBE technique that combines ex situ high-resolution transmission electron microscopy imaging with in situ high-energy electron diffraction measurements. According to their findings, two-dimensional nuclei act as initial structures for NW formation, and this transition is typically driven by surface energy anisotropy. In summary, these studies indicate that the formation of III-nitride NWs is not epitaxially connected to the underlying substrate, which suggests that they can be grown on various substrates, including amorphous glass, metals, plastic, and so on. The flexibility to grow on different substrates enables flexible, integrated photonic devices [Citation39,Citation133]. For instance, the MBE technique has been employed as energy efficient, self-assembled, InGaN/GaN QDs in NW LEDs for red emission wavelength, was directly grown on a metal substrate such as Ti-coated bulk polycrystalline-Mo, was reported by Zhao [Citation134].
More recently, the PA-MBE technique has been employed to achieve well-aligned and large-scale GaN NWs on denser SiOx templates, as reported by Zhao et al. [Citation135]. The growth parameters, such as substrate temperature, gallium (Ga) flux, and nitrogen flow rate, were optimized to achieve these results. Furthermore, examination using high-resolution TEM confirmed that these NWs exhibited non-stacking characteristics and were free from dislocations. In addition to GaN NWs, InGaN/GaN dot-in-a-wire LED nanostructures were developed and inspected. These structures displayed sturdier emissions compared to those unswervingly developed on Si substrates, possibly due to the lower light absorption of the Si substrate. The development of III-nitride NWs on unconventional substrates could open up new applications in Si photonics, flexible optoelectronics, and electronics. Another report on the attainment of high-efficiency green, yellow, and red InGaN/AlGaN dot-in-a-wire NW heterostructure LEDs grown on Si(111) by RF-MBE was reported by Philip et al. [Citation136].
Recently, the development of single NW LED arrays involves synthesizing NWs of various diameters using SA-MBE [Citation78]. This process allows for the creation of on-chip full-color (red, green, and blue – RGB) tunable single NW LED pixels. By growing individual sub-pixels with diameters of 220, 320, 420, and 630 nm, the resulting peak emission wavelengths were measured at 659, 625, 526, and 461 nm (see in Figure a–c), respectively [Citation34]. This promising on-chip fabrication technique offers several advantages, including enhanced functionalities compared to ensemble green and red GaN-based planar devices [Citation137]. It also exhibits high current density (7 kA/cm2 measured at ∼3 V). It enables the production of nano-LEDs with the smallest diameters, improved carrier density, and better heat dissipation compared with AlInGaN-based core-cell NWs on Si [Citation138]. Moreover, the favorable nanostructure enables effective strain relaxation, causing the emission peak to remain nearly constant with increasing current, indicating a modest QCSE [Citation139].
Figure 11. (a) Schematic representation of monolithically integrated multicolor single NW LED pixels in a single chip; (b) I-V characteristic profile of individual (Red, Orange, Green, and Blue (ROGB)) subpixel NW LEDs; (c) EL studies of the individual (ROGB) subpixel NW LEDs; and (d) Light intensity as the function of current density characteristic profile. Figures reproduced with permission from Ref. [Citation78], Copyright © 2016, American Chemical Society.
![Figure 11. (a) Schematic representation of monolithically integrated multicolor single NW LED pixels in a single chip; (b) I-V characteristic profile of individual (Red, Orange, Green, and Blue (ROGB)) subpixel NW LEDs; (c) EL studies of the individual (ROGB) subpixel NW LEDs; and (d) Light intensity as the function of current density characteristic profile. Figures reproduced with permission from Ref. [Citation78], Copyright © 2016, American Chemical Society.](/cms/asset/6a4e450f-9482-4c11-a088-cd1e70495257/tjid_a_2282937_f0011_oc.jpg)
Substantial efforts have been devoted to the growth of III-nitride NWs on patterned surfaces to achieve precise control over their position, height, and size uniformity [Citation140,Citation141]. In this growth process, a mask layer is initially applied to the substrate surface. Subsequently, the growth and formation of the NWs are guided by the nanoholes generated on the mask layer. The process of III-nitride NW growth on patterned substrates may differ from the accelerated growth or spontaneous creation of III-nitride NWs. Gacevic et al. conducted in-depth experimental and theoretical research on the MBE production of GaN NWs on a GaN substrate employing a TiNx mask [Citation142]. They proposed a two-stage growth process. During the initial growth stage, a single nanocrystal forms in each nanohole, driven by SAG kinetics. Starting nucleation occurs at the inner periphery of the nanohole. In the second growth stage, the generated nanocrystal undergoes morphological development, driven by the minimization of free-surface energy. It shows an initial cylindrical-like shape, transitions to an intermediate dodecagonal shape, and eventually adopts a final, thermodynamically stable hexagonal shape. From this point onward, the NW continues to grow vertically while maintaining its reliable hexagonal shape.
3. III-nitride nanowires for µ-LEDs
Compared to traditional QW devices, wire-in heterostructures can exhibit much lower dislocation densities and strain distribution due to effective lateral stress relaxation. There are two primary types of heterostructures: uniaxial and coaxial. Uniaxial heterostructures are created in the direction of the growth of the NW, while coaxial heterostructures involve a core–shell structure grown in seeded (catalyst-assisted) and self-seeded (catalyst-free) NWs. Historically, coaxial heterostructures are more frequently used for selected area NWs, while uniaxial heterostructures have recently shown some progress. The growth of uniaxial heterostructures is primarily limited by the challenges of avoiding concurrent coaxial growth while creating the uniaxial segment and the requirement for sufficient composition tunability. Furthermore, downsized NW devices can be seamlessly incorporated into silicon (Si) or other economically viable, expansive foreign substrates. This facilitates the on-chip amalgamation of LED arrays spanning from microscale to nanoscale, facilitating a myriad of applications, including but not limited to lighting, displays, lashing, imaging, sensing, hydrogen generation, and communications.
This section focuses on a review of recent advancements made on III-Nitride NW LEDs within the visible spectral regions. Furthermore, the conversation delves into the dynamics of carriers and various loss mechanisms observed in NW architectures, which include Auger recombination, randomized carrier dispersal due to subpar hole transit, electron leakage, and nonradiative surface recombination. Moreover, this study delves into in-depth analyses of full-color NW LED arrays, including the investigation of color-tunable NWs. Additionally, it emphasizes the remarkable progress made with uniaxial core–shell NW LEDs, demonstrating a remarkable enhancement in carrier injection efficiency. Particularly noteworthy is the successful construction of energy-efficient phosphor-free NW white LEDs, representing a highly promising advancement in the field.
3.1. Co-axial nanowires-LEDs
Co-axial NWs -LEDs are often composed of materials like silicon or sapphire and comprise vertically aligned III-nitride NWs with nonpolar (m-plane) and/or semipolar MQWs [Citation144]. The active zone, made up of coaxial shell layers around the NW core, is where the light-emitting process happens. The polarization field and the accompanying QCSE can be significantly reduced or entirely removed compared to conventional c-plane QW devices, which improves radiative recombination in the QW active region. The large active surface area of the active zone on the side wall also significantly improves the light extraction efficiency. For example, Qian et al. [Citation143] successfully presented the first triangular smooth-facet single GaN-based coaxial NW LEDs using GaN/InGaN/GaN core-multi-shell NW heterostructures. In this structure, an inner n-type GaN core and progressively deposited i-InGaN, i-GaN, p-AlGaN, and p-type GaN shells were utilized, as seen in Figure a (insect). The n-type GaN core functions as an electron injection layer, while the p-type GaN outer shell serves as a hole injection layer. Moreover, the InxGa1-xN layer with a tunable band gap, dependent on the value of x, enables efficient radiative recombination of injected carriers. Additionally, the AlGaN cladding layer has a wider band gap and lower index of refraction, which enhances the confinement of carriers and photons in the InGaN active layer. This approach results in the observation of smooth facet formation, as detailed in Figure a, demonstrating the establishment of a core–shell structure without any dislocations or boundaries. Electroluminescence studies demonstrate that core/multishell NWs when subjected to forward biases, operate as LEDs with a tunable emission range spanning from 365 to 600 nm and exhibit excellent QE, see Figure b. By adjusting the alloy composition in the active region during growth, the material bandgap can be precisely controlled, allowing for the emission of different colors of light. This flexibility facilitates the development of full-color displays and advanced lighting systems. The Co-axial heterostructure architecture of the LED promotes efficient carrier transport and photon extraction. Furthermore, the Co-axial design allows for effective light extraction, as photons generated within the NWs can be emitted laterally, reducing internal optical losses. InGaN Co-axial NW LED heterostructures have demonstrated significant advancements in terms of efficiency and color purity. Many promising developments have been reported regarding numerous attempts to realize large-surface emissive coaxial NWs using this directional growth approach. For instance, in 2013, Ra et al. [Citation80] successfully demonstrated the cohesive integration of InxGa1–xN/GaN multiple QW layers with hexagonal c-axis n-GaN NWs on Si(111) substrates, which was accomplished by employing a pulsed flow MOCVD method, where the MQW layers were grown coaxially on the sidewalls of the NWs. As of that controlled synthesis of uniaxial (c-plane) and coaxial (m-plane) heterostructures of InGaN/GaN MQW through metalorganic chemical vapor deposition (MOCVD), shown in Figure . An MQW single NW with c-plane and m-plane orientation was utilized to simultaneously produce the LEDs using a focused ion beam. To precisely assess the impact of polarization, electroluminescence with power dependency was conducted on both c-plane and m-plane oriented MQW NWs [Citation145]. The electrical properties of m-plane NWs surpassed those of c-plane NWs, mainly because they lack piezoelectric polarization fields. This study suggests that utilizing high-quality m-plane coaxial NWs holds the potential to produce exceptionally bright LEDs. Ongoing research aims to enhance the performance of these technologies by further optimizing material growth, device design, and fabrication procedures. Researchers strive to increase efficiency and extend device lifespan by improving crystal quality, reducing surface flaws, and optimizing contact surfaces. These advancements demonstrate significant promise for next-generation LED technology, with applications in optoelectronic components, displays, and solid-state lighting. Subsequently, a non-polar InGaN/GaN core–shell single NW was developed and reported by Changyi Li et al. [Citation87]. This NW was used for laser applications. In this instance, the manufacture of the NW was accomplished using a hybrid technique that includes a top-down, two-step etch process followed by a bottom-up, re-growth process. The hybrid technique applied enables precise geometrical control, substantial material enhancement, and effective optical confinement. Micro-photoluminescence was employed to evaluate the modal gain spectra and gain curves of the core–shell NW lasers, with subsequent analysis of the data conducted through the Hakki-Paoli method. The measured semipolar InGaN/GaN core–shell NWs had much lower lasing thresholds due to high optical gain, along with shorter cavity lengths and a smaller active area volume.
Figure 12. (a) Dark-field scanning TEM image of a cross-sectional GaN/InxGa1-xN/GaN/AlGaN CMS NW (insect: scheme of CMS cross-sectional view); (b) Normalized EL spectra recorded from five representative forward-biased multicolor CMS NW LEDs. Figures reproduced with permission from Ref. [Citation143], Copyright © 2005, American Chemical Society.
![Figure 12. (a) Dark-field scanning TEM image of a cross-sectional GaN/InxGa1-xN/GaN/AlGaN CMS NW (insect: scheme of CMS cross-sectional view); (b) Normalized EL spectra recorded from five representative forward-biased multicolor CMS NW LEDs. Figures reproduced with permission from Ref. [Citation143], Copyright © 2005, American Chemical Society.](/cms/asset/2d47d1d1-27a6-4590-b52c-e01b6e34389d/tjid_a_2282937_f0012_oc.jpg)
Figure 13. Schematic illustration of (a) c-plane (uniaxial) and (b) m-plane (coaxial) oriented MQW NWs, with respective FE-SEM images tilt-view FE-SEM image and HR-TEM images (bottom), of c-plane oriented (top from top left to right), and I–V characteristics of c- and m-plane-oriented NW LEDs and (b) the EL emission intensity of c- and m-plane-oriented NW LEDs at an injection current of 25 μA (bottom of right side). Figures reproduced with permission from Ref. [Citation80], Copyright © 2014, American Chemical Society.
![Figure 13. Schematic illustration of (a) c-plane (uniaxial) and (b) m-plane (coaxial) oriented MQW NWs, with respective FE-SEM images tilt-view FE-SEM image and HR-TEM images (bottom), of c-plane oriented (top from top left to right), and I–V characteristics of c- and m-plane-oriented NW LEDs and (b) the EL emission intensity of c- and m-plane-oriented NW LEDs at an injection current of 25 μA (bottom of right side). Figures reproduced with permission from Ref. [Citation80], Copyright © 2014, American Chemical Society.](/cms/asset/f8ab1198-3284-4b80-ba49-13144a0b23da/tjid_a_2282937_f0013_oc.jpg)
Meantime, MOVPE-grown single NW InGaN/GaN core–shell LEDs with transparent graphene-based p-contact for hole injection were achieved, as reported by Tchernycheva et al. [Citation146]. The ability to adjust the color range from blue to green was achieved by increasing the rate at which the injection current flows because when the injection is low, the majority of carriers gather at the tip of the NWs. Moreover, the fabrication of core–shell NWs also involved the implementation of the pulsed laser deposition (PLD) technique [Citation147]. Figure displays the fabrication of a GaN/InGaN core–shell device with an ITO p-contact, reported by [Citation20]. This device achieved a significantly lower turn-on voltage of approximately 2.65 V compared to the planar counterpart device. Additionally, the core–shell device exhibited a consistent peak emission wavelength, which can be attributed to the absence of the QCSE in the nonpolar m-plane InGaN QW active region. Moreover, the device confirmed a transition from blue to red emission as the injection rate increased from 3 to 10 V (see Figure e and f).
Figure 14. Characteristic InGaN/GaN MQW nanorod LED and field distribution model scheme for color-tunable LED profile. (a) TEM image (low magnification) of InGaN/GaN MQW nanorod of the topmost plane at 45° angle; (b, c) higher magnification images, and (d) EDX spectrum profile; (e, f) Color tunability spectrum and corresponding photocopy as a function of current injection rate. Figures reproduced with permission from Ref. [Citation20], Copyright © 2011, John Wiley and Sons.
![Figure 14. Characteristic InGaN/GaN MQW nanorod LED and field distribution model scheme for color-tunable LED profile. (a) TEM image (low magnification) of InGaN/GaN MQW nanorod of the topmost plane at 45° angle; (b, c) higher magnification images, and (d) EDX spectrum profile; (e, f) Color tunability spectrum and corresponding photocopy as a function of current injection rate. Figures reproduced with permission from Ref. [Citation20], Copyright © 2011, John Wiley and Sons.](/cms/asset/131a7fcd-e2ac-4a40-b5e9-b9a4f1504d3c/tjid_a_2282937_f0014_oc.jpg)
In general, the deposition of the p-contact in GaN-based devices is typically limited to the nonpolar QW active region. Achieving high-efficiency GaN devices relies heavily on precise control of p-type doping and GaN structure [Citation148]. This is crucial because the carriers (holes) injected into the device need to traverse the polar and semi-polar top regions of the NWs. So far, magnesium has proven to be a successful p-type dopant for GaN-based optoelectronic and electrical devices. However, achieving efficient p-type doping is challenging due to several factors, including the high Mg receptor ionization energy, limited Mg solubility, and sensitivity to compensatory defects [Citation149–153]. Consequently, the limited availability of free holes in these materials worsens the issue of electron overflow from the active region, which results in an undesirable emission peak and diminishing efficiency, particularly when operating at higher injection currents [Citation154–156]. Numerous studies have been conducted on Mg-doped GaN structures, exploring its effects on structural properties [Citation157], surface charge parameters with Mg inclusion [Citation158–160], and photoelectrochemical measurements on Mg-doped GaN NWs [Citation35]. Recently, Ra et al. [Citation158] found that Mg-doping at various growth stages gives rise to distinct structural morphologies in p-GaN nanostructures, which depends on the level of Mg incorporation and the growth conditions. Polished nanocrystals were observed at lower Mg cell temperatures, while higher temperatures led to virtuous surfaces with enlarged NW diameter (260 nm in diameter with 700 nm in height) achieved at a low Mg cell temperature of 310°C (see Figure a). Upon raising the Mg cell temperature to 340 °C, the diameters steadily increased (310 nm), while the heights decreased from 700 nm to 620 nm (see Figure b). Moreover, the NW surfaces transformed from smoother to rougher shapes with an expanded upper section, and the top surface displayed a slightly rounded structure rather than a crisp hexagonal pattern (refer to Figure c). As the Mg cell temperature was further increased to >370°C, a noticeable increase in NW shape inhomogeneity occurred, and there was a sudden jump in NW diameter from 255 nm (Mg cell temperature at 290°C) to 320 nm, as shown in Figure d. Figure e displays the cathodoluminescence (CL) spectra of Mg-doped GaN NWs, revealing a broad spectral band emission characteristic of conventional Mg-doped GaN structures, compared to the strong near-band-edge emission observed in undoped GaN. The increase in Mg doping causes a red-shift in wavelength, leading to more prominent ultraviolet spectral and defect-state emissions [Citation148,Citation161,Citation162]. This effect is most pronounced in the sample with the highest Mg doping concentration, indicating a significantly higher density of Mg receptors within the structure. At low levels of Mg doping, an emission peak emerges at 3.24 eV, attributed to a deep ground state of Mg and associated with the donor–acceptor pair (DAP) process, specifically linked to the deep acceptor Mg, resulting in an energy level change at 3.16 eV. As the Mg doping concentration increases further, the emission peak undergoes a shift to a lower energy level at 2.98 eV, also associated with the DAP process, indicating the presence of a higher concentration of Mg atom impurities exceeding 5 × 1018 cm−3. Additionally, the turn-on voltage improves with increasing Mg doping (see Figure [f]). Furthermore, Figure (g) demonstrates that well-controlled Mg doping enhances luminescence efficiency in green emission (532 nm), but high Mg doping also leads to leakage current due to Mg impurities.
Figure 15. The p-i-n heterostructure NWs from a 45° tilted view and a top-view perspective captured by FE-SEM. These NWs were grown at three different Mg cell temperatures: (a) 310°C, (b) 340°C, and (c) 370°C; (d) presents the changes in NW diameter and length corresponding to the Mg cell temperature variations; (e) normalized CL spectra of doped and undoped GaN NWs; (f) I-V studies of low, medium, and high Mg-doping levels of GaN NWs; (g) EL characteristic spectra of low, medium, and high Mg-doping levels of GaN NWs (measured at 4 V under continuous wave bias). Figures reproduced with permission from Ref. [Citation158], Copyright © 2019, American Chemical Society.
![Figure 15. The p-i-n heterostructure NWs from a 45° tilted view and a top-view perspective captured by FE-SEM. These NWs were grown at three different Mg cell temperatures: (a) 310°C, (b) 340°C, and (c) 370°C; (d) presents the changes in NW diameter and length corresponding to the Mg cell temperature variations; (e) normalized CL spectra of doped and undoped GaN NWs; (f) I-V studies of low, medium, and high Mg-doping levels of GaN NWs; (g) EL characteristic spectra of low, medium, and high Mg-doping levels of GaN NWs (measured at 4 V under continuous wave bias). Figures reproduced with permission from Ref. [Citation158], Copyright © 2019, American Chemical Society.](/cms/asset/b3650be2-2166-4808-bfcc-5a659d0ddf53/tjid_a_2282937_f0015_oc.jpg)
To enhance the performance of the device, Kolper et al. [Citation163] developed an alternative approach. They created axial NW LEDs with a larger coverage area, employing a passivation lid and a translucent ITO conducting layer, which was applied across the entire surface of each NW. This ITO shell served as a current spreading layer, ensuring uniform injection of carriers into the active region of the LED device. Furthermore, that placed a transparent dielectric material within the gap between the NWs to enhance the LED’s performance.
By utilizing an ITO current spreading layer, both the series resistance of the core–shell LED structure and current thronging could be reduced (see the schematic illustration in Figure ). However, the IQE achieved thus far is within 20% to 40%, which falls short of the highest performance of c-plane LEDs [Citation20,Citation164]. The primary disadvantage associated with this arrangement is that adjacent NWs can easily absorb the photon emission by a single core–shell NW LED, reducing QE. Recent research report reveals that, due to the QCSE, the absorption coefficient of m-plane QWs with an identical In structure may be more than ten times greater than that of c-plane QWs, which mitigates the polarization issue [Citation163]. Core–shell NW LED performance may potentially be hampered by nonradiative surface recombination. Furthermore, more efficient core–shell NWs in the yellow, orange, and red wavelength regions have proven challenging to produce, in part because the MOCVD technique’s comparatively high growth temperature restricts the Indium incorporation.
Figure 16. A standard core-shell structure based on NWs, produced through epitaxial growth of the n-doped cores. The schematic representation portrays various components: a dark grey segment depicting the dielectric mask, a dark blue region representing the active layer, and a light gray area symbolizing p-doped GaN. The NW array contains an outer shell made of ITO, indicated by the color yellow. Additionally, the space within the NW array is filled with a transparent dielectric, depicted as light grey. Figures reproduced with permission from Ref. [Citation163] Copyright © 2012, John Wiley and Sons.
![Figure 16. A standard core-shell structure based on NWs, produced through epitaxial growth of the n-doped cores. The schematic representation portrays various components: a dark grey segment depicting the dielectric mask, a dark blue region representing the active layer, and a light gray area symbolizing p-doped GaN. The NW array contains an outer shell made of ITO, indicated by the color yellow. Additionally, the space within the NW array is filled with a transparent dielectric, depicted as light grey. Figures reproduced with permission from Ref. [Citation163] Copyright © 2012, John Wiley and Sons.](/cms/asset/ac47afae-6649-4907-89d7-56194926717f/tjid_a_2282937_f0016_oc.jpg)
A recent study by Yong-ho Ra and Cheul-ro Lee [Citation144] demonstrated a class of phosphor-free white LEDs using nonpolar core–shell InGaN/GaN NW tunnel junction topologies on Si. The structure consisted of six equally sized layers of hexagonal shell QWs (5-6 nm) with Al tunnel junctions (3 nm) formed in the m-plane (1100) direction, allowing uniform growth along all six faces of the hexagon (see Figure (i[D-iii])234). The formation of the tunnel junction was further confirmed by composition variation in epitaxial n-GaN/Al/p-GaN probes (Figure (ii–d)). The significance of this structure lies in the absence of a p-contact layer. The absence of noticeable changes in the I-V curves with increasing temperatures validated the effective functioning of the Al tunnel junction interconnect, even at low temperatures, resulting in a lesser voltage drop (3.1 V) (see Figure [iv-a]). Moreover, the tunnel junction device exhibited a significantly lower turn-on voltage due to the efficient hole injection of the Al tunnel junction and reduced contact resistance of the n-GaN layer. While both NW LED devices exhibited similar luminescence characteristics under electrical pumping, the tunnel junction core-shell LED demonstrated considerably enhanced hole injection and transport into the InGaN QW active region ((see Figure (iv-b)). This improvement is believed to result from the elimination of a resistive and absorptive p-GaN contact layer through the tunnel junction. The efficiency of the tunnel junction core-shell LED only slightly declined or remained stable when the current density was increased up to 2700 A/cm2, compared to the core-shell LED, which measured at 650 A/cm2 (see in Figure [v-a]). The enhancements in EQE and efficiency droop were attributed to several factors, including superior electron confinement and hole transportation provided by the tunnel junction, the nonpolar QW active region, the nearly defect-free InGaN NW construction, and increased carrier injection efficiency facilitated by the expanded core-shell n-GaN contact area (see in Figure [v-b]). The emission wavelength exhibited no discernible shift with increasing injection current, indicating minimal QCSE and the use of multiple-stacked QWs (see Figure [v-c]). The white-light emission remained highly stable, as evidenced by the derived ranges of x- and y-values (0.35 to 0.36 and 0.38 to 0.39, respectively) for injection currents ranging from 500 A/cm² to 2500 A/cm² (see in Figure [v-d]).
Figure 17. demonstrates the multiple-stacked nonpolar InGaN/GaN core–shell with tunnel junction interconnection structure (i) SEM image associated schematic structure (right) and cross-sectional view (insect image); (ii) SEM image of cross-sectional view of as-grown sample and Energy band diagram of a multiple-stacked core–shell TJ construction with two Al TJs and InGaN QWs, and EDX profile at TJ and n-GaN layer positions; (iii) Energy band diagram of the TJ based on n++-GaN/Al metal/p++-GaN construction; (iv) (a) comparison I-V features of the multi-stacked core-shell LED with and without TJ (insect image: schematic representation TJ core-shell LED device), and (b) I-V studies as the function of temperature using TJ core-shell LED device; (v) (a) current density Vs. light intensity at room temperature, (b) comparative EQE as a function of current densities, (c) EL spectrum profile under different injection currents, (d) CIF chromaticity diagram of the TJ core–shell LED. Figures reproduced with permission from Ref. [Citation144], Copyright © 2020, American Chemical Society.
![Figure 17. demonstrates the multiple-stacked nonpolar InGaN/GaN core–shell with tunnel junction interconnection structure (i) SEM image associated schematic structure (right) and cross-sectional view (insect image); (ii) SEM image of cross-sectional view of as-grown sample and Energy band diagram of a multiple-stacked core–shell TJ construction with two Al TJs and InGaN QWs, and EDX profile at TJ and n-GaN layer positions; (iii) Energy band diagram of the TJ based on n++-GaN/Al metal/p++-GaN construction; (iv) (a) comparison I-V features of the multi-stacked core-shell LED with and without TJ (insect image: schematic representation TJ core-shell LED device), and (b) I-V studies as the function of temperature using TJ core-shell LED device; (v) (a) current density Vs. light intensity at room temperature, (b) comparative EQE as a function of current densities, (c) EL spectrum profile under different injection currents, (d) CIF chromaticity diagram of the TJ core–shell LED. Figures reproduced with permission from Ref. [Citation144], Copyright © 2020, American Chemical Society.](/cms/asset/21e2117e-8dba-40c5-8ddb-a612a3dff2f1/tjid_a_2282937_f0017_oc.jpg)
3.2. Uniaxial nanowires
NWs are grown vertically along the axial direction, providing a unique geometry for efficient light extraction and enhanced device performance. The heterostructure design and fabrication for these axial NW LEDs is essentially the same as that for traditional QW LED devices, with the exception that a dielectric layer, such as SiO2, spin-on glass (SOG), polyimide, or perylene, is frequently employed to planarize the NW surface. Due to their unique one-dimensional structure, InGaN axial NWs offer several advantages over conventional planar LEDs. The NW geometry enables a larger surface-to-volume ratio, enhancing light extraction efficiency and reducing the requirement for thick absorbing layers. This leads to improved light extraction and higher internal QE. InGaN uniaxial NW LEDs have shown promising results in terms of their electrical and optical properties. However, challenges such as uniformity in NW growth, precise control of alloy composition, and efficient current injection still need to be addressed to fully exploit their potential for practical applications. Both top-down and bottom-up methods have been used for the development of these axial III-nitride NWs.
3.2.1. Top-down approach to uniaxial nanowires
Wang’s team [Citation165] has reported the development of improved blue and green InGaN/GaN-based nanorod array light emitting diodes (LEDs) by utilizing a self-assembled Ni nano-mask (10 nm thick) and dry etching techniques. These NW-array LEDs demonstrated higher output power compared to their flat counterparts due to enhanced light extraction efficiency, abridged strain distribution, and polarization fields. Furthermore, the NW LEDs displayed a slender radiation profile, characterized by intensified light emission primarily in the vertical direction. This phenomenon arose from the combined effects of the vertical directing effect and Bragg scattering facilitated through adjacent NWs [Citation166–168]. Unfortunately, the performance of these top-down NW devices was adversely affected by etching-induced surface damage, nonradiative surface recombination, and carrier leakage. Consequently, these issues resulted in abridged QE and efficiency droop at high injection current levels [Citation169,Citation170]. In the same way, the growth of planar heterostructures hindered the development of long-wavelength LEDs. To overcome these limitations, extensive research has focused on the bottom-up fabrication of NW LEDs, which shows promising potential in addressing these performance constraints.
3.2.2. Bottom-up approach to uniaxial nanowires
In this approach, NWs are typically synthesized starting from the atomic or molecular level and gradually built up to form the structure. This method utilizes building blocks at the atomic or molecular level, enabling precise and controlled development in terms of structural formation and composition. Possible approaches include growth formation guided by catalyst-assisted and self-seeding (catalyst-free) processes. Typically, catalyst-assisted methods such as the VLS method are widely employed in the earlier stages for the growth of metal catalysts on Si or sapphire substrates [Citation171]. Low-pressure MOVPE [Citation172], Hot-wall CVD [Citation173], and low-temperature halide chemical vapor deposition [Citation174] have all been used to produce such NWs. However, the optical and electrical performance of the device can be considerably diminished by the accidental introduction of metal catalysts into NW materials [Citation175]. On the other hand, catalyst-free or self-seeded axial NW LEDs have been utilized for spontaneous generation or SAG, achieving significantly better performance [Citation176]. MBE is an excellent choice for the growth of III-nitride NWs, which enables spontaneous formation without using an external metal catalyst. For example, self-seeded, well-aligned uniaxial (Al)InGaN NW LED heterostructures can be fabricated by MBE on arbitrarily large-area, low-cost Si or SiO2 substrates [Citation135]. In 2004, Kim et al. [Citation177] investigated the successful fabrication of uniaxial InGaN/GaN MQWs on a glass substrate using Metal Organic Hydride Vapor Epitaxy. The fabrication involved isolating individual nanorods (NRs) and establishing contact between p-type NRs and p-type electrodes. The nanorod sidewalls, which offer a large surface area and minimal dislocations, significantly enhance internal and extraction efficiency. As a result, these nanorod-based LEDs produce approximately 4.3 times more light intensity at 20 mA DC compared to conventional LED counterparts. SAG is another process utilized in the production of III-N NW LEDs, where NWs are grown on patterned substrates [Citation128,Citation178]. One notable advantage of SAG is its ability to accommodate thermal stress during heteroepitaxial growth and reduce threading dislocations on the formed surface by trapping and bending through the lateral overgrowth. A study conducted by Tanaka et al. [Citation178] focused on investigating the geometrical effects of SAE growth of GaN on sapphire to improve light extraction from GaN LEDs. To minimize experimental sampling errors, the researchers conducted systematic tests on size and spacing effects using a single 2-inch sapphire substrate containing 144 pattern arrays. Circular mask-opening windows in SiO2 were used for these experiments. The study revealed that altering the mask opening diameter resulted in a four-fold increase in layer thickness at 20-micrometer spacings and a three-fold increase at dot diameters of 350 micrometers. By achieving precise control over facet formation, the researchers demonstrated that GaN/InGaN MQW LEDs with well-aligned facets exhibited 2.5 times higher electroluminescence properties compared to non-faceted devices. In a recent study, Qwah et al. [Citation179] delved into exploring the influence of alloy disorder on vertical transport within unintentionally doped (UID) c-plane Ga-polar InGaN heterostructures. The main focus of their research was to understand how the disorder in the alloy composition affects the movement of charge carriers in the vertical direction. Their research involved both theoretical and experimental analyses, with a focus on demonstrating unipolar hole transport. Increasing the thickness of the UID In0.1Ga0.9N heterostructure layer from 15 nm to 30 nm resulted in a significantly high voltage drop of 2 V at 500 A/cm2, as in forward bias, compared to the reverse bias voltage drop (0.2 V at 500 A/cm2). Additionally, the voltage penalty for the QW structures increased as the number of QWs grew from one to three, in both the forward and reverse biases, with a rate of 0.25 V per QW at 500 A/cm2, see Figure . These findings provide valuable insights into the transport characteristics and behavior of UID c-plane Ga-polar InGaN heterostructures, shedding light on the impact of alloy disorder on their performance in vertical transport applications. The performance of uniaxial NW-LEDs with dual heterostructures, QW/QD, and QDs as active regions is described in the following section.
Figure 18. (a) Room temperature I-V characteristics of InGaN double heterostructure NW LED, fabricated on Si (001) (insect scheme); (b) observed EL spectrum as a function of injection current, and corresponding spectrum (inserted image). Figures reproduced with permission from Ref. [Citation180], Copyright © 2010, American Chemical Society.
![Figure 18. (a) Room temperature I-V characteristics of InGaN double heterostructure NW LED, fabricated on Si (001) (insect scheme); (b) observed EL spectrum as a function of injection current, and corresponding spectrum (inserted image). Figures reproduced with permission from Ref. [Citation180], Copyright © 2010, American Chemical Society.](/cms/asset/9343d818-d965-4712-8a5b-85d8933eb4e2/tjid_a_2282937_f0018_oc.jpg)
3.2.2.1. Wire in heterostructures
The InGaN active region within the NW heterostructure allows for the emission of several; colors spanning the visible spectrum. The essential p-type and n-type parts of the NW heterostructure are produced using doping procedures. To maximize the electrical and optical performance of the devices, techniques for establishing Ohmic connections and passivation layers are also essential. By adjusting the indium composition, the emission wavelength of the LED can be precisely tuned, offering flexibility. For instance, Hahn et al. [Citation17] demonstrated the heteroepitaxial growth of InxGa1-xN NW arrays (0.06 ≤ x ≤ 0.43) on c-plane sapphire (Al2O3 (001)) via halide chemical vapor deposition (HCVD). Figure illustrates the electrically driven color-tunable emission from a p-n junction of InxGa1-xN NW-based devices grown epitaxially on p-GaN(001). These devices exhibit emission in three distinct colors: blue (x = 0.06), green (x = 0.28), and orange (x = 0.43), showcasing the remarkable capability of color control through variation in the indium composition (x) within the NWs. Notably, when incorporating InGaN NWs into functional LEDs, p-n junctions must be formed for efficient carrier injection and recombination.
Figure 19. Illustrates SEM images (tilted at 45°) and corresponding PL spectra emission images of InxGa1-xN NW arrays on Al2O3 with X = 0.06, 0.28, and 0.43. Figures reproduced with permission from Ref. [Citation17], Copyright @ 2011, American Chemical Society.
![Figure 19. Illustrates SEM images (tilted at 45°) and corresponding PL spectra emission images of InxGa1-xN NW arrays on Al2O3 with X = 0.06, 0.28, and 0.43. Figures reproduced with permission from Ref. [Citation17], Copyright @ 2011, American Chemical Society.](/cms/asset/77e6ae07-2ebf-4fa7-a34f-c43101f4773c/tjid_a_2282937_f0019_oc.jpg)
Another study by Guo et al. [Citation180] successfully demonstrated the ability to tailor the peak emission wavelength of InGaN NWs (InGaN/GaN double heterostructure), ranging from ultraviolet to red, by adjusting the In content in the composition using MBE. This was achieved by forming wire-in-heterostructure, resulting in a ‘white’ emission when the In concentration was continuously varied during growth. The internal QE of these NWs can reach 20% to 35%. Photoluminescence studies conducted on InGaN NWs emitting at a wavelength of 490 nm at ambient temperature showed radiant and nonradiative lifetimes of 5.4 and 1.4 ns, respectively.
3.2.2.2. Quantum well nanowires
Recent studies have demonstrated improved carrier confinement in the NW active region through fabricating QW-in-wire heterostructure LEDs. Notably, Kikuchi et al. [Citation181] reported a fascinating advancement in the production of GaN/InGaN NWs within MQD LEDs on n-type Si (111) substrates. Their innovative design involved remote columnar in the n-GaN and InGaN/GaN MQD active region, while the diameters gradually increased in the p-GaN region through precise growth condition control. The most remarkable outcome was the creation of a nanocolumn LED with a continuous surface, completely free of any chasms. This achievement was made possible by employing a transparent p-type contact on top, ensuring the excellent optical properties of the isolated nanocolumn active region were maintained. The LED emitted light ranging from green to red under direct current operation at room temperature, with rectifying behavior observed and a typical turn-on voltage of 2.5-3.0 V. Another noteworthy advancement in this field was achieved by Guo et al. [Citation182] in 2011, who successfully developed InGaN/GaN disk-in-NW white light emitting diodes on Si (001) using plasma-assisted MBE. In this design, the upper section of the NWs, known as p-GaN, was grown laterally to form a merged film, which greatly simplified constructing the devices. As a result, the produced LEDs emitted a pleasant white light with precise chromaticity coordinates measured at x = 0.29 and y = 0.37. Additionally, these LEDs exhibited a correlated color temperature ranging between 5500 K and 6500 K, showcasing their potential for various applications. One of the remarkable features of these LEDs was their ability to maintain droop-free EQE (External QE) values even at high injection current densities, specifically up to 400 A/cm2. According to Kim et al. [Citation177], MQW-NW arrays were grown on sapphire utilizing MO-HVPE. As a result, the electroluminescence properties showed improved light intensity and a very minor peak shift at 460 nm at a high injection current of 100 mA compared with a low injection current of 20 mA, see Figure .
Figure 20. (a) Schematic representation of fabricated InGaN/GaN MQW nanorod array and is associated using SEM image (right), (b) observed EL characteristic spectrum using fabricated InGaN/GaN MQW nanorod array LED. Figures reproduced with permission from Ref. [Citation177], Copyright © 2004, American Chemical Society.
![Figure 20. (a) Schematic representation of fabricated InGaN/GaN MQW nanorod array and is associated using SEM image (right), (b) observed EL characteristic spectrum using fabricated InGaN/GaN MQW nanorod array LED. Figures reproduced with permission from Ref. [Citation177], Copyright © 2004, American Chemical Society.](/cms/asset/c43d764f-9e8a-4fa2-ab17-31aff0723bd3/tjid_a_2282937_f0020_oc.jpg)
Another promising achievement, studied by Armitage et al. [Citation183], is the demonstration of multicolor emission from a single InGaN QW grown on a series of c-axis GaN NW arrays with varying geometrical parameters, using MBE. Remarkably, the density of GaN wires exhibited a substantial influence on the InGaN PL emission, even in arrays with similar average diameters. Surprisingly, manipulating the wire density resulted in the production of a visually perceived white multicolored emission. The study also emphasized that the strain in the InGaN QW, influenced by the wire diameter, showed no correlation with the dependence of the InGaN photoluminescence (PL) emission on the geometrical parameters of the underlying GaN NW array. In light of these findings, the researchers proposed that the incorporation of In during InGaN development is influenced by variations in the InGaN alloy composition. Guo et al.’s [Citation182] presentation of InGaN/GaN NW LEDs with several disks integrated into the active region is another example of this single QW NWs structure.
More recently, Gujrati et al. [Citation184] made a significant breakthrough by indicating the fabrication of defect-free sidewalls in Multiple Shapes µ-LEDs through a straightforward liftoff and transfer process, leveraging SAG on a hexagonal-BN pattern. This innovative approach ensures the production of µ-LEDs with precise shapes and addresses the common issue of sidewall defects encountered in traditional fabrication methods. The utilization of h-BN as a template material provides an ideal growth surface, facilitating the successful transfer of the fabricated µ-LEDs. This research advancement holds immense potential for enhancing the performance and reliability of µ-LED technology, thereby contributing to the development of high-quality display systems and optoelectronic devices. The procedure begins by applying van der Waals epitaxy to grow a 2D h-BN layer on sapphire substrates. These substrates are adorned with silica masks, featuring square, triangular, and hexagonal patterns. These distinct patterns on the masks define the regions where the µ-LEDs will be fabricated, as illustrated in Figure . Subsequently, SAG of MQW LED heterostructures happens, resulting in ultra-smooth crystalline sidewalls that can reach an impressively small size of 1.4 µm. Because the h-BN layer lacks vertical chemical bonds, it allows for a straightforward mechanical lift-off and transfer process for an array of LED heterostructures. This process effectively reduces their size to as small as 8 µm. Subsequently, transparent indium tin oxide (ITO) p-contacts are uniformly deposited on the LEDs using a lift-off method, resulting in remarkably bright LEDs with enhanced performance.
Figure 21. Optical microscopic images of SiO2 masks such as (a) Square, (b) hexagon, and (c) triangle shapes. (here, the green part is opening). Figures reproduced with permission from Ref. [Citation184], Copyright © 2023, John Wiley and Sons.
![Figure 21. Optical microscopic images of SiO2 masks such as (a) Square, (b) hexagon, and (c) triangle shapes. (here, the green part is opening). Figures reproduced with permission from Ref. [Citation184], Copyright © 2023, John Wiley and Sons.](/cms/asset/1ffac44a-809b-4673-9a70-9af180cc838f/tjid_a_2282937_f0021_oc.jpg)
3.2.2.3. Quantum dot nanowire
Wire-in-dot nanostructures that are quantum-confined in three dimensions are due to the nanoscale dimensions of the QDs within the NW structure InGaN QD-NWs. These QDs confine electrons and holes in three dimensions, leading to discrete energy levels and quantum size effects. Furthermore, by altering the compositions and/or sizes of the dots within a single epitaxial growth step, it is possible to effectively regulate the temperature and color chromaticity. As a result, InGaN QD-NWs demonstrate remarkable optical and electronic properties, such as high photoluminescence quantum yield, narrow emission line widths, and fast carrier dynamics. For instance, Nguyen et al. [Citation185] published a report on catalyst-free InGaN/GaN dot-in-wire nanoscale heterostructures on Si (111) substrates. By changing the dot composition, they achieved strong emission spanning almost the entire visible wavelength range. Moreover, by adjusting the indium composition of the dots during a single epitaxial growth step, they demonstrated the production of white LEDs without phosphors. These devices exhibited relatively high internal QEs (>20%) and no apparent efficiency droop, even at current densities of up to approximately 200 A cm−2.
More recently, Ra et al. [Citation78] made a significant breakthrough by displaying the fabrication of multicolor single InGaN/GaN dot-in-NW light-emitting diodes (LEDs) on the same substrate using SAG. The size of the NW substantially impacts the structural and optical characteristics of InGaN/GaN QDs. As the NW diameter increases, the photoluminescence emission of single InGaN/GaN dot-in-NW structures consistently shifts toward the blue. This phenomenon is attributed to the significantly increased incorporation of indium (In) in NWs with smaller diameters, resulting from the greater contribution to In incorporation from the lateral diffusion of In adatoms. By combining the development of individual single-color emitting InGaN/GaN NW LEDs through composition changes during the growth phase, it was possible to fabricate multi-color (RGB) emissions in a single chip. Additionally, these NW LEDs display excellent electrical performance, with a turn-on voltage of under 2 V and minimal current leakage when the bias is reversed.
The aforementioned NW LED displays present numerous promising benefits, such as dislocation-free color tunability and quantum-controlled emission capabilities. However, they often suffer from modest output power, which makes them challenging prospects for future display technology. Additionally, a significant number of carrier loss channels and nonradiative recombination are likely present, as evidenced by the slow increase in EQE with injection current. The section that follows investigates the underlying reason behind the weak output power and low QE typically observed in NW LEDs.
3.3. Efficiency drop management in InGaN/GaN LEDs
Despite significant advances, the InGaN NW has a considerable distance to fully unbridle its potential, caused by its low QE-experiencing shortened output power. Generally, impurities or crystal defects found in semiconductors significantly impact the longevity of charge carriers by creating defect levels or energy levels that do not coincide with the boundaries of the band gap. These defect levels, also known as trap levels, often capture charge carriers. During a two-step recombination process called Shockley–Reid–Hall recombination, conduction electrons move from the conduction band to the defect state and then to the valence band, thereby eliminating a hole. These trap levels, situated within the forbidden band, possess the capacity to introduce significant faults and impact the overall behavior of the semiconductor.
‘Current drop’ is the term used to describe performance degradation caused by rising operating temperature or rising current density. For instance, InGaN/GaN LEDs now on the market are produced on standard c-plane on sapphire; nevertheless, the efficiency drops as the current density rises, a phenomenon known as ‘efficiency drop’ or ‘current drop’ [Citation186]. The efficiency and efficiency drop of a typical c-plane InGaN/GaN LED are shown in Figure . Despite the significant progress in this area, several factors caused by the lattice and temperature mismatch between III-nitrides and sapphire substrate have a major impact on the performance and applicability of III-nitride planar devices [Citation187–189]. As a result, a high density of threading dislocations ranging from 106 to 108 cm2 serves as non-radiative recombination centers. A set of reported values of InGaN QW nanostructure LED nonradiative recombination values is listed in Table . Similarly, the low output power and low QE of NW LEDs can be attributed as possible factors, including poor hole transport [Citation190,Citation191], carrier leakage [Citation192], Auger recombination [Citation193–195], PSF effect [Citation196,Citation197], quantum confined Stark effect (QCSE) [Citation198], carrier dislocation [Citation199,Citation200], and nonradiative surface recombination [Citation201,Citation202].
Figure 22. The internal QE and efficiency droop as a function of current density for a typical c-plane InGaN/GaN LED. Figures reproduced with permission from Ref. [Citation186], Copyright © 2018 Elsevier, Ltd.
![Figure 22. The internal QE and efficiency droop as a function of current density for a typical c-plane InGaN/GaN LED. Figures reproduced with permission from Ref. [Citation186], Copyright © 2018 Elsevier, Ltd.](/cms/asset/2481dc73-2433-405d-bc18-c2b01d3b4dc2/tjid_a_2282937_f0022_oc.jpg)
Table 2. Magnitudes of observed recombination parameters for InGaN QWs obtained from previous reports (λ, QW emission wavelength; (A) SRH recombination; (B) radiative recombination; (C) Auger recombination).
According to the ABF model, the IQE and carrier recombination studies are as follows:
(1)
(1) where,
represents the internal QE, and N, stands for the carrier density; A, B, and C are denoted such that the recombination coefficients of nonradiative SRH, radiative, and Auger, respectively. f(N) describes some higher-order carrier loss processes, such as Auger recombination and electron overflow.
Generally, the vast surface areas of NW devices facilitate highly efficient lateral stress relaxation, significantly reducing polarization fields and dislocation density. However, the high surface-to-volume ratios of NWs can introduce surface states and defects, potentially increasing carrier loss in NW LEDs. The impact of Auger recombination on the performance of GaN-based devices is still not well understood [Citation193,Citation210–212]. Moreover, in c-plane nitrides, spontaneous and piezoelectric polarization fields arise, causing the QCSE and reducing efficiency due to Auger recombination and electron leakage during heteroepitaxial growth [Citation10,Citation21,Citation22,Citation187,Citation213,Citation214]. Various factors such as internal heating, carrier injection and transport, and recombination dynamics in the active region can decline the efficiency and thermal performance of InGaN/GaN LEDs.
3.3.1. Auger recombination process
At high carrier concentrations, Auger recombination frequently takes place, entailing the transfer of surplus energy from an electron-hole pair to a neighboring particle in the system by a non-radiative recombination process. The likelihood of this Auger process significantly diminishes as the energy band gap increases, and in materials with wide gaps, it is commonly considered insignificant [Citation215,Citation216]. Delaney et al. [Citation217] explored the Auger coefficient calculation in wurtzite InGaN using first principle density-functional and many-body-perturbation theory. Their findings infer that, within the blue to green spectral range, the coefficient can reach a remarkably high value of 2 × 10−30 cm6 s−1, at room temperature. Notably, its magnitude exhibits a significant increase in the infrared region. Additionally, at high current densities, Auger recombination becomes a substantial radiative loss process due to its cubic power dependence on the free-carrier concentration. Eliseev et al. [Citation218] extracted various recombination balance parameter measurements for GaN/InGaN/AlGaN single-QW LEDs for green emission from optical power and carrier lifetime measurements, unveiling a broad range of Auger coefficient range, spanning from 1 × 10−34 to 5.37 × 10−28 cm6s−1. Later, Shen et al. conducted measurements on the Auger recombination coefficient in quasi-bulk GaN/InGaN QW LEDs under optical pumping, which revealed that the coefficient ranged from 1.4 × 10−30 to 2 × 10−31 cm6 s−1, alongside defect densities ranging from 107 to 109 cm−2. According to Kioupakis, the substantial Auger coefficient is primarily caused by indirect Auger recombination by phonon and alloy scattering [Citation211]. Nguyen et al. [Citation81] found a modest Auger coefficient in the dot-in wire system based on thorough investigations of the temperature-dependent emission properties of WLEDs. The Auger coefficient values observed in InGaN/GaN heterostructures are enumerated in Table . Investigation into the precise role of Auger recombination in the performance decline of InGaN LEDs is still crucial, especially for novel NW devices.
Table 3. Auger coefficients in InGaN/GaN heterostructures values based on the previous report.
3.3.2. Hole transport and carrier distribution losses
In general, various hole transport in InGaN/GaN NW heterostructure LED active regions is very inefficient, compared to electron transport, resulting in uneven distribution of carriers throughout the active region of the LED owing to high effective mass and low mobility of holes. The concentration of holes is mostly at the p-doped GaN layer and decreases significantly as one moves toward the n-GaN region [Citation186]. Such as the large valence band offset between InGaN and GaN materials results in significant energy barriers for hole injection and transport across the heterointerface [Citation191,Citation220–222]. This leads to a reduced hole injection efficiency and restricts the effective utilization of holes for light emission or carrier recombination [Citation221]. Instead, the occurrence of polarization-induced electric fields in these heterostructures further exacerbates the poor hole transport. The spontaneous and piezoelectric polarizations arising from the lattice mismatch between InGaN and GaN layers induce a built-in electric field across the structure [Citation216]. This field spatially separates the electrons and holes, making it challenging for holes to reach the active regions and participate in the desired optical processes. Additionally, the formation of potential barriers at the heterointerface due to alloy disorder and strain effects hinders hole transport [Citation223]. Localized compositional fluctuations within the InGaN layer create potential fluctuations, leading to scattering and trapping of holes, thus reducing their mobility and effective transport through the structure [Citation224]. The poor hole transport process significantly affects the performance of devices based on InGaN/GaN nanoscale heterostructures. In LEDs, for instance, limited hole injection and transport result in an imbalanced carrier population, leading to efficiency droop – a decline in LED efficiency at high current densities. This droop phenomenon restricts the maximum achievable light output and poses a fundamental limitation in the growth of high-power and high-efficiency LEDs [Citation62]. Several strategies have been proposed to overcome the poor hole transport challenges in InGaN/GaN downscaled heterostructures [Citation223,Citation225]. These include band engineering techniques to optimize the valence band alignment and reduce the energy barriers for hole injection [Citation82]. Interface engineering methods, such as strain engineering and interfacial layer insertion, aim to alleviate the effects of polarization-induced electric fields and enhance hole transport across the heterointerface [Citation62,Citation77,Citation81,Citation137,Citation186,Citation223].
3.3.3. Electron overflow/leakage
Electron leakage/overflow refers to the undesirable escape of electrons from the active region of an LED, leading to reduced current spreading and inefficient carrier recombination. Several factors contribute to electron leakage in InGaN NW LEDs. Electrons can tunnel through the potential barriers, like thin barrier layers or defects, and escape from the QW region [Citation226]. In conditions of high carrier density states, Auger recombination, a nonradiative process, can cause electrons to be quickly transferred to higher energy states, leading to leakage [Citation154,Citation227]. Surface states or defects at the NW sidewalls can trap electrons, reducing their effective injection into the active region [Citation202,Citation228]. Nguyen et al. [Citation229] published a detailed report on electron overflow in InGaN/GaN dot-in-wire LEDs. To curtail electron overflow, a frequently employed approach involves integrating a p-doped AlGaN electron-blocking layer between the active region and the p-GaN layer. However, the existence of strong polarization forces has reduced the efficacy of this method in ordinary c-plane QW LEDs. The introduction of a p-doped AlGaN electron-blocking layer could significantly reduce electron overflow in NW architectures. For injection currents of 1200 A/cm2 and operating in a wide temperature range, NW LEDs showed no efficiency droop. To decrease electron overflow in NW LEDs, additional methods, such as tunnel injection, have also been investigated [Citation144,Citation230].
3.3.4. SHR nonradiative recombination
These factors include the negative impact of SRH nonradiative recombination, which mainly arises owing to their high surface-to-volume ratios, the occurrence of surface states, and surface defects [Citation231]. Compared to conventional InGaN/GaN QW blue LEDs, which have a QE of only 10 A/cm2, NW LEDs demonstrate a gradual improvement in their QE as the current increases. Their peak efficiency is achieved at relatively high injection levels (4100 A/cm2). However, under conditions of high excitation, the dominance of surface recombination, along with carrier spillover/leakage, can lead to a decrease in efficiency in NW LEDs. To tackle this issue, various passivation techniques can be employed to minimize nonradiative surface recombination and significantly enhance the performance of these devices.
3.3.5. Carrier injection efficiency
The issues related to material quality, band offsets, strain, surface states, and Auger recombination pose significant limitations to achieving high efficiency. NW devices exhibit significantly low carrier injection efficiencies (less than 10%) primarily attributed to the prevalence of nonradiative surface recombination, setting them apart from conventional QW LEDs, which achieve nearly perfect carrier injection efficiencies. Defects, such as threading dislocations and point defects, can act as nonradiative recombination centers, reducing the efficiency of carrier injection and resulting in lower device performance. The band offsets between the InGaN well and barrier layers significantly influence carrier injection efficiency. InGaN NW MQWs often suffer from a high electron barrier owing to the large conduction band discontinuity between the InGaN QW and barrier materials. This barrier creates a potential energy barrier for electrons, leading to poor carrier injection from the barrier to the well. Consequently, the injection of electrons into the active region is restricted, adversely affecting the overall device performance. InGaN NWs experience strain and piezoelectric fields due to lattice mismatch between the InGaN layers and that growing substrate. These fields can distress the carrier dynamics within the MQWs. The strain-induced piezoelectric fields can cause spatial separation of electrons and holes, reducing the overlap, hence the recombination probability. This spatial separation hampers the carrier injection efficiency and leads to a diminution in the overall QE of the device. Studies have verified a rapid decline in carrier injection efficiency as the diameter of NWs decreases. NWs with diameters around 100 nm exhibit considerably less than 10% carrier injection efficiency. The inherently low carrier injection efficiency of these devices accounts for the typically quoted extremely low output power of standard NW LEDs. Furthermore, the substantial loss of carriers due to nonradiative surface recombination leading to high junction temperatures can be added to undermine the efficiency of NW LEDs.
3.4. Progress on efficiency loss overcoming strategy
3.4.1. Uniaxial core-shell NW LEDs
The inadequate QE and output power of uniaxial NW LEDs can be primarily ascribed to the surface recombination efficiency, leading to non-radiative recombination. To tackle this challenge, various surface passivation procedures have been extensively studied [Citation85,Citation232–235]. Among these techniques, the combined in-situ grownup large bandgap shell has emerged as an exceptionally promising and efficient approach to augment the performance of these devices [Citation223,Citation236]. Because the effectiveness of light extraction can be greatly improved with an integrated monolithic light reflector and NW-based LEDs. A high-quality Al metal sheet immediately coated by the MBE method during the epitaxial growth serves as the optical reflection for the GaN-based NW LEDs. Light extraction is positioned quite vertically in GaN-based NW LEDs [Citation41]. Additionally, SAG presents a viable method for effortlessly producing well-organized arrays of GaN NWs with excellent crystal quality and the essential aspect ratio. In addition, a state-of-the-art etching method is being developed for making NW with the support of the Al layer acts as a mirror. The nearly flawless NW LED heterostructures exhibit significantly enhanced light emission efficiency, completely devoid of any dislocation-related emissions. For instance, Kazuma et al. [Citation237] discovered that when AlGaN spacers were inserted after the formation of each InGaN QWs, the NW-based LEDs displayed a distinct red shift from 430 nm to 520 nm. The AlGaN spacer’s ability to prevent the breakdown of InGaN QWs while raising the growing temperature for GaN barriers demonstrates the potential for extending the EL emission wavelength. GaN/InGaN MQWs in NWs critical non-polarization quality was verified by EL spectra, which disclosed a steady emission spectrum as a function of injection current. Additionally, changes in c-plane MQS and yellow-red emission associated with point defects were validated by comparing EL and photoluminescence spectra by altering the activation annealing time and lowering the growth temperature of InGaN QWs.
Nguyen et al. [Citation229] have showcased a novel InGaN/GaN/AlGaN uniaxial heterostructure core-shell NW LED on Si, which exhibits a merger recombination effect and remarkable enhancement in carrier injection efficiency, thus further enhancing its overall effectiveness. The core-shell formation consists of an InGaN/GaN dot-in-wire as the core and an epitaxial large-band AlGaN as reflected in the shell. Additionally, this core-shell heterostructure was grown spontaneously, aided by a diffusion-controlled process. The large bandgap shell significantly controls nonradiative surface recombination, resulting in an upsurge in carrier injection efficiency caused by strong carrier confinement. The fabricated devices using the InGaN/GaN/AlGaN uniaxial core-shell NW heterostructure LED achieved an output power of approximately 1.5 mW at room temperature, which is more than two orders of magnitude stronger than NW LEDs without AlGaN shell coverage. Eventually, the same group reported another unique approach, a self-organized InGaN/GaN/AlGaN axial core-shell NW heterostructure LED [Citation223]. In this case, the GaN barrier layer was substituted by AlGaN, resulting in InGaN/AlGaN dot-in-wire core-shell LEDs. As the QD active region expanded, it has led to the vertical alignment of InGaN QDs closer to the center of the NW. Consequently, this development naturally caused the development of an AlGaN shell with a substantial bandgap surrounding the QDs. Thorough elemental analysis has verified the existence of an outer shell composed of Al-rich AlGaN encapsulating the InGaN QDs. It was discovered that using a stable, homogeneous core-shell heterostructure significantly enhanced the performance of NW LEDs. The final carrier lifetime increased significantly, from 0.3 ns to 4.5 ns, as a result of decreased surface recombination. The utilization of core-shell NW LEDs featuring p-doped AlGaN barriers enables the dispersion of electron-blocking layers throughout the structure. This dispersal enhances their effectiveness in preventing electron overflow when contrasted with the conventional setup where AlGaN electron-blocking layers are sited between the active region and p-GaN. The outcome of this design modification is a notably elevated output power of 5.2 mW.
To prevent surface recombination, several approaches have been employed, such as epitaxial growth or wet sulfide; however, these pricey or unstable processes disregard the viability of commercial production. A unique method using ion implantation was recently developed by Liu et al. [Citation238] to change sidewall high-density surface defects by successfully preventing carrier surface non-radiative recombination. As demonstrated by the scheme in Figure a, the following ICP etching procedure may have produced Ga-based dangling bonds, resulting in non-radiative recombination centers. The sidewall damage is caused by these unstable Ga-O and Ga-OH bonds, which can form when the dangling bonds come into contact with -O in the atmosphere or -OH in a potassium-based solution. This damage occurs due to the non-radiative recombination of electrons and holes at these connections. To prevent that, Ga-N and -N may substitute for -O and -OH after ion implantation, and this passivation also fills the hole defects. It significantly reduces non-radiative recombination on the sidewall surface. Figure b proves a substantial improvement in turn-on and bias voltages after ion implantation, with values decreasing from 3.4 V and 4.44 V to 2.08 V and 4.04 V, respectively. Additionally, the reverse leakage current is significantly reduced, and the LOP (light output power) increases by approximately 17.6%, as shown in Figure (c and d). Furthermore, N ion implantation leads to a 33% increase in the EQE of the packaged µLEDs chip, as depicted in Figure e. Subsequently, the implementation strategy of He+ was employed to reduce point defects [Citation239].
Figure 23. (a) Schematic representation on surface chemical structural modification when ion implementation process; comparative performance analysis before and after ion implementation µ-LEDs (b) I-V studies, (c) reverse leakage current, (d) LOP, and (e) EQE, respectively. Figures reproduced with permission from Ref. [Citation238], Copyright © 2021 Elsevier Ltd.
![Figure 23. (a) Schematic representation on surface chemical structural modification when ion implementation process; comparative performance analysis before and after ion implementation µ-LEDs (b) I-V studies, (c) reverse leakage current, (d) LOP, and (e) EQE, respectively. Figures reproduced with permission from Ref. [Citation238], Copyright © 2021 Elsevier Ltd.](/cms/asset/1a523c1c-6cd4-42e5-82d4-dc9cf302ac68/tjid_a_2282937_f0023_oc.jpg)
Wang et al. [Citation138] reported the direct growth of a core–shell heterostructure NW-based on AlInGaN on Si. In this study, an In-rich core and an Al-rich shell were spontaneously fashioned through the MBE techniques. From this approach, with a well-defined AlGaN shell successfully developed, the device realized a long carrier lifetime time of about up to 2 ns. With the dimension of 1 × 1 mm2 nonpackaged devices show a very high output power of over 30 mW, without aiding light extraction mode. Moreover, it has been shown the tunable emission spectrum ranges from 430 to 630 nm.
Recently, Ra et al. [Citation240] demonstrated the fabrication of an all-epitaxial, electrically injected, surface-emitting green laser using dislocation-free gallium nitride nanocrystal arrays, which utilize photonic band edge modes. The nanocrystal surface-emitting laser (NCSEL) diode was designed without the need for aid-based conventional distributed Bragg reflectors (DBRs). Figure illustrates the NCSEL as arrays of InGaN/AlGaN nanocrystals with precise control over size, spacing, and surface shape. The absence of dislocations in these nanostructures is attributed to effective strain relaxation. To mitigate the QCSE, numerous InGaN QDs are merged into the semipolar planes of the active region. Moreover, an AlGaN shell structure (Figure (ii)) is implemented around the active region of the NCSEL to prevent surface recombination. The novel aspect of this study lies in utilizing the photonic band edge resonant effect of the nanocrystal array to create an electrically injected NCSEL, eliminating the need for thick and resistive conventional DBRs. Operating at a wavelength of 523.1 nm, the device displays a remarkably low threshold current density of approximately 400 A/cm2 and maintains consistent performance at room temperature, as shown in Figure (iii). Notably, this threshold’s current density is significantly smaller compared to previously described blue LDs. This breakthrough opens up new possibilities for the design of low-threshold NCSEL spanning the ultraviolet to deep visible range (200 nm–600 nm). It overcomes limitations imposed by substrate accessibility, the scarcity of superior DBRs, and excessive lattice mismatch, thereby establishing a new paradigm in device performance.
Figure 24. Schematic representation of the InGaN nanocrystal arrays for the surface-emitting laser diode (NCSEL); (i) nanocrystal arrays and consistent SEM images; (ii) NW heterostructure and consistent SEM images as well as EDX spectrum; (iii) fabrication of NCSEL device; (a) I-V studies with surface contact area of about 25 µm2 (insect: current-leakage profile under reverse bias), (b) EL spectra recorded as a function of injection currents (200–400 A cm−2). Figures reproduced with permission from Ref. [Citation240], Copyright © 2020 Science Ltd.
![Figure 24. Schematic representation of the InGaN nanocrystal arrays for the surface-emitting laser diode (NCSEL); (i) nanocrystal arrays and consistent SEM images; (ii) NW heterostructure and consistent SEM images as well as EDX spectrum; (iii) fabrication of NCSEL device; (a) I-V studies with surface contact area of about 25 µm2 (insect: current-leakage profile under reverse bias), (b) EL spectra recorded as a function of injection currents (200–400 A cm−2). Figures reproduced with permission from Ref. [Citation240], Copyright © 2020 Science Ltd.](/cms/asset/659b1bd9-f363-47e2-81dc-3994a70a5863/tjid_a_2282937_f0024_oc.jpg)
3.4.2. Excitonic µ-LEDs
The fundamental limitations causing a reduction in LED efficiency [Citation241], as discussed in the section on efficiency drop management, stem from various factors such as Shockley-Read-Hall recombination, Auger recombination, and electron leakage. At high current densities, Auger recombination and electron overflow contribute to efficiency droop, while at low injection current, Shockley-Read-Hall recombination significantly limits QE [Citation34]. Consequently, the enhanced surface recombination in micro and nanoscale LEDs imposes a fundamental limitation on the external QE of traditional QW structures. Notably, the use of excitons, which are bound states formed by the strong Coulomb interaction between electrons and holes [Citation242,Citation243], offers a promising solution to significantly enhance the radiative recombination efficiency. This improvement creates an opportunity to make micro and nanoscale LEDs relatively resistant to defects and traps.
In conventional c-plane InGaN QW structures, excitonic effects encounter obstacles caused by piezoelectric polarization fields and the QCSE [Citation244,Citation245]. These factors diminish the exciton binding energy, limiting its potential to enhance µ-LED efficiency. Nonetheless, recent research indicates that GaN-based heterostructures can surmount this limitation through quantum and nanoscale engineering [Citation246,Citation247]. For instance, InGaN NWs with reduced dimensions show approximately two orders of magnitude increase in exciton oscillator strength compared to traditional QWs, owing to efficient strain relaxation [Citation248]. Moreover, in extreme monolayer III-nitrides, exciton binding energies as large as 200 meV to 1 eV have been achieved, and structures grown along semi-polar and non-polar planes exhibit even greater exciton binding energy due to reduced polarization fields and enhanced electron-hole wavefunction overlap [Citation243,Citation249]. These advancements suggest that NW excitonic LEDs, empowered by strain and quantum engineering, hold great promise as a solution to overcome the efficiency bottleneck of micro and nanoscale LEDs.
In an excitonic LED, electroluminescence arises from the fusion of an electron and a hole, as opposed to the conventional recombination of freely moving charge carriers. This unique characteristic reduces the negative effects of Shockley-Read-Hall recombination, thanks to the robust interaction between electrons and holes. As a result, the efficiency of excitonic LEDs is notably enhanced. These LEDs exhibit a remarkable and rapid rise in both EQE and device output power with increasing injection current, surpassing the capabilities of conventional InGaN QW µ-LEDs.
Pandey et al. [Citation250] investigated the impact of excitons on the performance of µ-LEDs. The study focused on two distinct NW arrays, referred to as array A and array B, which had different dimensions. Array A consisted of NWs with a diameter of around 125 nm and a lattice constant of 245 nm, while array B comprised NWs with a diameter of 165 nm but the same lattice constant. Following the fabrication of µ-LED devices, the devices in array A were designated as Device A, and those in array B were named Device B. Both devices featured injection windows covering an area of approximately 750 nm × 750 nm, as depicted in the inset of Figure a. The J-V characteristics of these devices were plotted in Figure a, demonstrating rectifying behavior with minimal reverse bias leakage, indicating well-defined p−n junction formation. Remarkably, device A, with smaller NW diameters, exhibited a unique excitonic effect, leading to an exceptionally high peak EQE of 25.2% (Figure a). This phenomenon was distinct and had not been observed in c-plane QW LEDs. In c-plane QW LEDs, the excitonic effect is weakened due to strong QCSE and high plasma damage caused by mesa etching, which makes it difficult to observe the effect at low injection currents. At high injection currents, the presence of indium-rich nanoclusters becomes saturated, and most of the emission arises from the diffuse c-plane InGaN through free electron-hole recombination rather than excitonic emission. Consequently, the transition from excitonic emission to band-to-band recombination yields a similar performance for NWs with different dimensions, as depicted in Figure b and c at relatively high current densities.
Figure 25. Comparative photonic performance studies for Devices A and B, which (a) illustrate the current-voltage (J-V) characteristics of Devices (insect: SEM image providing a detailed view of a representative injection opening within the NW array). (b) displays the light output power (Lop) as a function of injection current (I) (insect: green emission originating from a submicron device). (c) EQE as the function of current densities. (d) EL spectra as the function of current densities, respectively. (e) and (f) represents the relative EQE (dashed green curve) as well as individual contributions of the lower energy emission (black dashed curve) and higher energy emission (red dashed curve) for (e) Device A and (f) Device B, respectively. The solid blue curves in both figures are the measured EQE for each device, overlaid on top. Figures reproduced with permission from Ref. [Citation250], Copyright © 2023, American Chemical Society.
![Figure 25. Comparative photonic performance studies for Devices A and B, which (a) illustrate the current-voltage (J-V) characteristics of Devices (insect: SEM image providing a detailed view of a representative injection opening within the NW array). (b) displays the light output power (Lop) as a function of injection current (I) (insect: green emission originating from a submicron device). (c) EQE as the function of current densities. (d) EL spectra as the function of current densities, respectively. (e) and (f) represents the relative EQE (dashed green curve) as well as individual contributions of the lower energy emission (black dashed curve) and higher energy emission (red dashed curve) for (e) Device A and (f) Device B, respectively. The solid blue curves in both figures are the measured EQE for each device, overlaid on top. Figures reproduced with permission from Ref. [Citation250], Copyright © 2023, American Chemical Society.](/cms/asset/b094637a-9d18-4a5c-9f7e-d0f16f159f0e/tjid_a_2282937_f0025_oc.jpg)
Examining Figure d of the EL spectra, it becomes clear that the emission characteristics are influenced by the injection currents. At low injection currents, corresponding to the peak EQE, the emission is primarily governed by the lower energy peak centered around 515 nm. This emission is linked to excitonic emission originating from the indium-rich semi-polar quantum disks. As the injection currents increase, the emission from these quantum disks becomes saturated, and a shift occurs in the dominant emission mechanism. Band-to-band recombination from the central region of the NW (c-plane quantum disks) takes over, resulting in a progressively more prominent higher energy emission at approximately 475 nm–490 nm. Eventually, this higher energy emission becomes the dominant peak. In contrast, for Device B, no transition in the EL emission peak energy is observed beyond the maximum EQE of 4.1%. This observation suggests a prevalence of free electron-hole recombination in Device B. Upon close comparison of the EQE curves for Device A and Device B, they become nearly identical for injection currents above a few A/cm2, as depicted in Figure c. In this operational regime, both devices exhibit band-to-band recombination behavior, resembling conventional QW LED devices.
A comprehensive investigation into the influence of excitonic emission and band-to-band recombination on the overall EQE was conducted. Figure e illustrates the relative EQE for the lower-energy emission peak, approximately at 515 nm, in Device A (attributed to excitonic emission), and the higher-energy emission peak around 490 nm. Similarly, Figure f depicts the same plots for Device B. Remarkably, the relative EQE of the combined emissions from these peaks, represented by the dashed green curves for each device, closely mirrors the actual measured EQE shown by the solid blue curves. Both Devices A and B exhibit comparable trends, reaching peaks at a few A/cm2. This EQE behavior aligns with that of conventional QW LEDs and can be attributed to the prevalence of band-to-band recombination, in addition to Shockley-Read-Hall recombination and electron overflow mechanisms. However, the contribution of lower-energy emissions (dashed black curves in Figures (e and f)) differs between Devices A and B.
Device A exhibits several distinct characteristics, including a smaller diameter, efficient strain relaxation, enhanced faceting, and reduced polarization fields. Hence, in the low current regime, the low-energy (excitonic) emission is considerably more dominant than the higher-energy (band-to-band) emission. This significant difference in emission types leads to an unparalleled efficiency level, previously unattainable for µ-LEDs. On the contrary, Device B does not display a substantial excitonic effect, resulting in the low-energy emission remaining negligible across the entire range of measured currents. These investigations and analyses provide indisputable evidence that the remarkable efficiency enhancement observed in Device A cannot be attributed to variations in light extraction efficiency. Instead, it can be traced back to the excitonic emission originating from the strain-relaxed semi-polar quantum disks formed in dislocation-free NW arrays.
3.5 Nanowire red micro LEDs
The low efficiency of p-type doping has been attributed as a significant factor due to various limitations, including the high ionization energy of Mg acceptors, low solubility of Mg, and the tendency to form compensating defects [Citation251,Citation252]. Additionally, the low density of free holes in these materials can exacerbate electron overflow from the active region, leading to undesired emission peaks and reduced efficiency, particularly at higher injection currents. This issue becomes more pronounced in conventional top-down µLEDs, where plasma etching is commonly employed to define the device injection mesa. In this study, we focused on bottom-up red-emitting NW LEDs with different levels of Mg doping in the p-GaN layer. These sub-micron LED devices exhibited a clear enhancement in efficiency with increasing Mg dopant incorporation.
Referring to them as samples A and B, we utilized Mg BEP of approximately 5 × 10−8 and 5 × 10−9 Torr, respectively, for the p-doped layer [Citation160]. The J-V curves of the fabricated devices, shown in Figure a, demonstrate that device B exhibits higher forward and reverse currents compared to device A, indicating increased current leakage due to non-optimized Mg doping. Figure b presents the L-I characteristics of devices A and B, where a significant improvement in output power is observed at similar current densities for the device with higher Mg doping (sample A). The findings demonstrate that increased Mg doping results in a decrease in forward leakage current and leads to a boost in output power at comparable voltages. The marginally earlier turn-on voltage observed in device B can be attributed to the surplus current flowing through the leakage paths. Therefore, while the bottom-up approach effectively minimizes surface recombination, the degradation of the p-type layer remains a concern in achieving efficient micro LEDs. Although the disparity in Mg doping can contribute to the differences in the electrical properties of the devices, it is challenging to isolate the impact of doping incorporation and etching damage since plasma etching is essential for microLED fabrication.
Figure 26. (a) J–V characteristics of devices A and B. (b) L–I characteristics of devices A and B. (c) Variation of EQE with current density for devices A and B. The solid curves are a guide to the eye. (d) Electroluminescence spectra measured at different injection currents for device A. Figures reproduced with permission from Ref. [Citation160], Copyright © 2023, AIP Publishing.
![Figure 26. (a) J–V characteristics of devices A and B. (b) L–I characteristics of devices A and B. (c) Variation of EQE with current density for devices A and B. The solid curves are a guide to the eye. (d) Electroluminescence spectra measured at different injection currents for device A. Figures reproduced with permission from Ref. [Citation160], Copyright © 2023, AIP Publishing.](/cms/asset/eca499e8-dd16-4fff-9e2d-41b5daada9e4/tjid_a_2282937_f0026_oc.jpg)
The EQE measured for the devices at various injection currents are plotted in Figure c. The EQE peak increases and shifts to lower currents as the Mg flux increases. Device A, with high Mg incorporation, achieved a maximum EQE of approximately 8.3% at a current density of around 1 A/cm2. Figure d illustrates the normalized electroluminescence (EL) spectra for device A, ranging from approximately 0.5 to 14 A/cm2 in injection currents. At a current of approximately 0.5 A/cm2, the spectrum exhibits an emission peak at approximately 650 nm, resulting in a deep red color when the device operates near its efficiency peak. An optical microscope image of device A during operation is provided in the inset of Figure d, displaying vivid red emission even in a sub-micrometer scale device.
3.5. Phosphor-free nanowire white LEDs
Today’s LED technology utilizes rare earth-doped phosphors to produce long-wavelength emissions such as green, yellow, orange, and red, as well as white light emission. However, the frequency range extends beyond just a few MHz [Citation253]. Unfortunately, the conversion of blue light emitted by InGaN QW LEDs into green, red, and white light presents significant challenges. These challenges include reduced device efficiency, compromised device stability, and diminished light quality [Citation53,Citation254–257]. These problems arise due to factors such as the delayed Stokes transfer process and extended lifetime of YAG:Ce phosphor [Citation258], the resistive-capacitance (RC) time delay of wide-area WLEDs, the QCSE, and the intrinsic piezoelectric polarization from InGaN/GaN QWs [Citation259]. Moreover, the YAG:Ce phosphor has notable drawbacks, including a broad emission spectrum, a lack of a red spectrum component, large particle size (∼10 µm), color conversion limitations, and phosphor efficiency droop [Citation260]. Another consequence is the gradual degradation of these phosphor materials due to the heat generated during the operation of phosphor-converted-white LEDs. This unintentional change in the phosphors properties results in a decrease in their light conversion efficiency, ultimately leading to blue-shifted white emissions [Citation261]. On the contrary, researchers have developed alternative color conversion materials such as conjure-polymer [Citation259] QDs [Citation262] and carbon dots [Citation263] to overcome the constraints of phosphor-converted white LEDs (PC-WLEDs). However, WLEDs utilize these new color conversion materials, despite having a typical short fluorescence lifetime of several or tens of nanoseconds [Citation264]. Consequently, these solutions fail to address the reduction in the RC time delay and output polarization field in extremely small chip lighting applications. More importantly, WLEDs operate at extremely high current densities, often exceeding kA/cm2 [Citation265], which is impractical for real-world applications due to µ-LED efficiency droop and heat dissipation issues [Citation264].
Recent studies have ushered in a new era in InGaN NW LED lighting, paving the way for phosphor-free white LEDs, which offer a viable alternative for producing effective light across the entire visible spectrum [Citation17,Citation20,Citation81,Citation180,Citation266,Citation267]. Achieving white light emission can involve a combination of multiple colors emitted within a single NW, which can be controlled by adjusting the composition in the active region [Citation180,Citation223,Citation236]. For instance, a class of phosphor-free white LEDs using nonpolar core–shell InGaN/GaN NW tunnel junction topologies on Si has been reported as highly stable white-light emission with a CCT of 4700 K (see in Figure (d)) [Citation144]. Additionally, WLEDs with core–shell tunnel junction NWs have dramatically boosted output power by decreasing nonradiative surface recombination and enhancing carrier injection efficiency [Citation144]. Another approach involves precise control of white light emission by varying the size and composition of the InGaN QD active region, along with incorporating nearly defect-free GaN NW phases [Citation81,Citation185]. In the realm of phosphor-free white LEDs, various contemporary techniques for the fabrication of phosphor-free white LEDs exist, including layer-by-layer indium gallium nitride (InGaN) growth, employing different indium compositions in each active layer [Citation268–270], or integrating InGaN QDs into InGaN layer structure [Citation271,Citation272]. These NWs possess several advantageous characteristics that can greatly improve the efficiency, lifespan, and overall quality of solid-state lighting. By directly generating and controlling light across extensive visible spectral regions, these NWs offer a multitude of benefits. Furthermore, phosphor-free NW white LED devices have shown remarkable internal QE values [Citation81,Citation180,Citation267]. Compared to conventional InGaN/GaN QW structures, the injected carriers collected by the unique localized states of InGaN QDs, with alleviative QCSE, exhibit a broad spectrum and high modulation bandwidth. Notably, Wan et al. unveiled a single-chip white light-emitting diode (WLED) incorporating self-assembled InGaN QDs, showcasing an extensive range of correlated color temperatures (CCT) spanning from 1600 K to 6000 K. This device showed an impressive color rendering index (CRI) of 75 (in Figure a and d). Furthermore, the WLED demonstrated a significant modulation bandwidth of 150 MHz at a low current density of 72 A cm−2 (in Figure b), with a −3 dB modulation depth (in Figure c) [Citation264].
Figure 27. The characteristic performance of single chip WLEDs, namely (a) CCD and CRI as a function of injection current, (b) frequency response, (c) bandwidth as a function of injection current density, and (d) EL spectra at 72 A cm-2. The bottom images show that EL spectrum images correspond to increasing injection current density response. Figures reproduced with permission from Ref. [Citation264], Copyright: © 2020 Chinese Laser Press.
![Figure 27. The characteristic performance of single chip WLEDs, namely (a) CCD and CRI as a function of injection current, (b) frequency response, (c) bandwidth as a function of injection current density, and (d) EL spectra at 72 A cm-2. The bottom images show that EL spectrum images correspond to increasing injection current density response. Figures reproduced with permission from Ref. [Citation264], Copyright: © 2020 Chinese Laser Press.](/cms/asset/ff913bca-3ffd-4fd2-a22a-0d2c33d94584/tjid_a_2282937_f0027_oc.jpg)
3.6. Full-color nanowire LEDs
A full-color emitter simultaneously emits the primary colors red, green, and blue (RGB), and when their wavelengths coincide, they combine to produce secondary colors. When the RGB emissions are perfectly synchronized, they generate white light at the center. In principle, the correlative color temperature (CCT) of LEDs should have the ability to be tuned over a wide range, which is vital for countless claims, including displays [Citation22,Citation273], backlighting [Citation273,Citation274], and real-time identification of biological cells [Citation275]. In terms of imaging optics, maintaining brightness simply requires minimizing optical energy transmission in the air. However, non-imaging techniques aiming to overcome this limitation must address optical loss mechanisms occurring on the LED chip, such as light deflection through texturing, resorption in the active layers, and impurity or free-carrier absorption. The radiation pattern of light from the active layer, often overlooked in discussions about LED light extraction, is another important factor [Citation22].
Currently, on-chip fabrication of full-color LEDs is achieved by employing phosphor-assisted tunable emission from blue GaN-based LEDs [Citation276,Citation277]. However, the spectrum for acceptable color emission significantly limits power generation flexibility, resulting in limited Color Rendering Index (CRI) values and Stokes fluorescence loss [Citation278]. An alternative approach involves on-chip integration of submicron-sized, high-efficiency multicolor light sources, such as red, green, and blue (RGB), which emit broad-spectrum light in the visible region with high CRI values, bypassing phosphor [Citation22,Citation76]. Thus, nano-LEDs integrated on a single chip could become the ultimate emissive LEDs with low power consumption, incredibly small size, and excellent CRI [Citation34]. However, the efficiency of GaN-based LEDs in the wavelength range of >560 nm is currently very low due to the lack of suitable lattice-matched substrates for high-In content QWs formation. In this regard, high-efficiency NW LEDs with emission wavelengths spanning the entire visible spectrum range show great promise for the development of high-performance full-color LEDs. The one-dimensional columnar architecture of each nanostructure, necessary for producing ultra-high-definition displays, allows customization of the emission cone and direction [Citation279].
µ-LEDs face a fundamental limitation in terms of efficiency cliff caused by surface recombination, which becomes dominant in small-area devices. This limitation arises from the decrease in the contribution of In adatoms due to lateral displacement as the NW diameter increases. By designing NWs with varying diameters, it is possible to achieve different InGaN compositions, leading to different emission wavelengths. Single nano-LED pixel arrays can also operate at very high current densities and improve heat dissipation [Citation137]. In the context of these new technological domains, the development of full-color, programmable emitters, including LEDs and lasers, on a single chip is crucial. To achieve this, precise compositional alterations of the alloy must be made at several nanostructured regions, completed in a single process step. In this regard, Sekiguchi et al. [Citation280] reported the monolithic integration of three-primary-color nano-light-emitting diodes by regulating the composition of InGaN QWs on the same wafer, enabling on-chip full-color emission.
The experiment utilized Ti-mask SAG by rf-plasma-assisted MBE to create InGaN/GaN MQW nanocolumn arrays on the same substrate, with nanocolumn widths ranging from 137 nm to 270 nm. As the nanocolumn diameter increased, the emission color transitioned from blue to red (479 to 632 nm in wavelength), as shown in Figure . The beam shadow effect of the nearby NWs clearly explains the mechanism behind the emission color change. Albert et al. [Citation281] demonstrated the manufacturing control of blue, green, and red light emissions with wavelength control by altering the growth temperature, In/Ga ratio, and III/V ratio. They showed that low growth temperatures and high In/Ga ratios encourage the incorporation of In and that changing these parameters directly affects the structural properties of the materials. Furthermore, they demonstrated that a single nanopillar heterostructure composed of three red, green, and blue-emitting InGaN segments can generate white light.
Figure 28. SEM characteristic images and their associated optical emissive images of InGaN/GaN NW columns. Typical diameters are (a) 143 nm, (b) 159 nm, (c) 175 nm, (d) 196 nm, (e) 237 nm, and (f) 270 nm, respectively. Figures reproduced with permission from Ref. [Citation280], Copyright © 2010, AIP Publishing.
![Figure 28. SEM characteristic images and their associated optical emissive images of InGaN/GaN NW columns. Typical diameters are (a) 143 nm, (b) 159 nm, (c) 175 nm, (d) 196 nm, (e) 237 nm, and (f) 270 nm, respectively. Figures reproduced with permission from Ref. [Citation280], Copyright © 2010, AIP Publishing.](/cms/asset/23c9b1c4-99d9-4ef8-980b-420abf80dbd0/tjid_a_2282937_f0028_oc.jpg)
In their study, Wang et al. [Citation76] highlighted the ability to achieve controllable and tunable full-color light generation. They accomplished this by integrating blue, green/yellow, and orange/red InGaN NW light-emitting diodes (LEDs) on a Si substrate using a three-step SA-MBE growing technique, as depicted in Figure a. Figure b illustrates the PL characteristic spectrum profile of the as-grown LED subpixel measured at 300 K, revealing that the emission wavelength changes as a function of the diameter of the NWs. The arrangement of multicolor subpixels in a latitudinal orientation facilitated controlled light mixing at the chip level, and enabled the emission of color-tunable light with correlated color temperature (CCT) values ranging from 1900K to 6800 K, all while maintaining exceptional color rendering capacity (see Figure [c and d]).
Figure 29. (a) schematic representation of the integration of a multicolor LED on a chip. (b) illustrates the normalized PL characteristic spectrum profile of the as-grown LED subpixel measured at 300 K. (c) showcases the normalized EL characteristic spectrum profile of the as-grown LED subpixels. (d) displays the output spectra of a sample triple-color LED pixel, consisting of three 300 × 300 µm2 devices individually biased as the function CW injection currents. Figures reproduced with permission from Ref. [Citation76], Copyright © 2014, Optical Society of America.
![Figure 29. (a) schematic representation of the integration of a multicolor LED on a chip. (b) illustrates the normalized PL characteristic spectrum profile of the as-grown LED subpixel measured at 300 K. (c) showcases the normalized EL characteristic spectrum profile of the as-grown LED subpixels. (d) displays the output spectra of a sample triple-color LED pixel, consisting of three 300 × 300 µm2 devices individually biased as the function CW injection currents. Figures reproduced with permission from Ref. [Citation76], Copyright © 2014, Optical Society of America.](/cms/asset/9fe7a65d-1b20-433b-965c-704395bcd0df/tjid_a_2282937_f0029_oc.jpg)
In a recent study, Ra et al. [Citation78] showcased the successful assembly of multicolor, single InGaN/GaN NW LED arrays on a single chip through the implementation of epitaxial SAG. To regulate the size of the NWs, a hole-patterned titanium mask was implemented during the process. Additionally, electron-beam lithography (EBL) was employed on a GaN/Sapphire substrate to define nanoscale growth patterns of arrays with varying diameters and spacings, as demonstrated in Figure . According to Figure c, the peak emission wavelength varies as a function of NW diameter, which ranges from 150 nm to 2 µm, under the same epitaxy conditions, these fluctuations demonstrate a consistent blue shift as the NW diameter increases.
Figure 30. The schematic illustration of on-chip full-color LED fabrication process (a) hole patterned Ti mask with various diameters (b) the formation of multicolor single InGaN/GaN dot-in-NW LED pixel arrays and Single InGaN/GaN dot-in-NW LED architectures, (c) Peak emission wavelength of a single InGaN/GaN NW as a function of NW diameter, grown at 795 (sample I), 810 (sample II), and 825°C (sample III), (d) Normalized PL spectra of InGaN/GaN dot-in-NW structures in Sample II, recorded at ambient temperature, as a function of NW diameter. Figures reproduced with permission from Ref. [Citation78], Copyright © 2016, American Chemical Society.
![Figure 30. The schematic illustration of on-chip full-color LED fabrication process (a) hole patterned Ti mask with various diameters (b) the formation of multicolor single InGaN/GaN dot-in-NW LED pixel arrays and Single InGaN/GaN dot-in-NW LED architectures, (c) Peak emission wavelength of a single InGaN/GaN NW as a function of NW diameter, grown at 795 (sample I), 810 (sample II), and 825°C (sample III), (d) Normalized PL spectra of InGaN/GaN dot-in-NW structures in Sample II, recorded at ambient temperature, as a function of NW diameter. Figures reproduced with permission from Ref. [Citation78], Copyright © 2016, American Chemical Society.](/cms/asset/3ca94269-99a5-42a2-9f35-d6da3558ec48/tjid_a_2282937_f0030_oc.jpg)
Specifically, the emission wavelengths of sample II NWs shift from 640 to 465 nm as the diameter increases. Similar trends are observed for NWs in samples I and III, albeit with a smaller tuning range due to variations in the growth environment. Figure d illustrates the photoluminescence (PL) emission spectra for NWs as a function of the InGaN/GaN NW diameter. Moreover, two sets of ensemble NWs were generated with average spacings of approximately 56 and 72 nm, respectively. Both the diameter and spacing of the NWs are crucial in governing the emission characteristics. As shown in Figure , it was observed that increasing the NW diameter while maintaining a constant spacing caused the peak emission wavelength to shift toward the red region. Specifically, with NW spacings of 56 and 72 nm, the blue and red curves in Figure c display a slight redshift (5 nm–13 nm) in the PL emission wavelength for identical NW diameters. This redshift can be attributed to the beam shadowing effect, resulting in reduced Ga incorporation at the growth front of the NWs. Furthermore, it was noted that the redshift becomes more pronounced as the NW spacing decreases.
Figure 31. Normalized photoluminescence (PL) spectra of the group of InGaN/GaN dot-in-NWs (a) the spectra for NWs with diameters of 133, 141, 166, 177, and 252 nm are in with an average spacing of 56 nm, (b) the spectra for NWs with diameters of 90, 130, 159, 175, 196, 260, and 500 nm are in with an average spacing of 72 nm, and, (c) a correlation between the peak emission wavelength and NW diameter with the blue curve denoting an average spacing of 56 nm and the red curve denoting an average spacing of 76 nm. Figures reproduced with permission from Ref. [Citation78], Copyright © 2016, American Chemical Society.
![Figure 31. Normalized photoluminescence (PL) spectra of the group of InGaN/GaN dot-in-NWs (a) the spectra for NWs with diameters of 133, 141, 166, 177, and 252 nm are in with an average spacing of 56 nm, (b) the spectra for NWs with diameters of 90, 130, 159, 175, 196, 260, and 500 nm are in with an average spacing of 72 nm, and, (c) a correlation between the peak emission wavelength and NW diameter with the blue curve denoting an average spacing of 56 nm and the red curve denoting an average spacing of 76 nm. Figures reproduced with permission from Ref. [Citation78], Copyright © 2016, American Chemical Society.](/cms/asset/4e46312d-e062-4bfb-9cc3-f1e52df53462/tjid_a_2282937_f0031_oc.jpg)
Similarly, Liu et al. showcased a significant advancement by integrating full-color (RGB – red, green, and blue) tunable single NW LED pixels, with lateral dimensions as small as 150 nm, onto a single device, as shown in Figure a. This remarkable feat was made possible by utilizing a single manufacturing method, which capitalized on the findings from a previous study [Citation78]. These have a typical height of 650 nm, a hexagonal shape, smooth side faces, and typical diameters of blue (D ∼630 nm), green (D ∼420 nm), orange (D ∼320 nm), and red (D ∼220 nm). A typical color emission concerning the dimensions of the I-V curves is shown in Figure b. In Figure c, individual EL spectra are presented for sub-pixels with widths of 220, 320, 420, and 630 nm, along with their corresponding peak emission wavelengths of 659, 625, 526, and 461 nm, respectively. Additionally, Figure d demonstrates that the EL intensity increases nearly linearly with the injection current. Notably, devices with larger diameters emit more light at the same current density due to their larger effective area. We attribute the ability of nano-LEDs with smaller surfaces to operate at higher current densities to their improved thermal and conduction efficiency. As the current increases, the emission peak remains relatively constant, indicating a small QCSE.
3.7. Stretchable-bendable transparent InGaN NW µ-LEDs
High-performance stretchable and bendable InGaN NW µ-LEDs, offering enhanced dynamic range, superior readability in diverse lighting conditions, and unprecedented form factors enable their adaptation in various devices such as wearable electronics [Citation282–284], foldable screens [Citation285,Citation286] and bio-integrated devices [Citation284,Citation287–290]. Recent research has shown that amorphous Si-based substrates can be utilized to create high-quality III-nitride NW LED heterostructures, presenting an opportunity for the development of flexible, multicolored, and highly efficient NW LEDs [Citation81,Citation180,Citation182,Citation229,Citation244,Citation291–296]. In addition, an InGaN/GaN NW LED for metal substrate with improved emission properties was demonstrated by Nguyen et al. [Citation297]. Here, as grown InGaN/GaN NW LEDs on SiOx/Si were directly transferred to a copper substrate. These devices exhibited superior performance compared to NW LEDs on Si, as evidenced by their improved current-voltage characteristics and emission intensity. This improvement can be attributed to the reduced photon absorption and enhanced heat dissipation capabilities of the supporting substrate. In a similar vein, Park et al. recently demonstrated the production of NW LEDs on polymer substrates using a comparable method [Citation298]. Besides, applying an accurate amount of compressive strain has been found to boost EQE and simultaneously alleviate efficiency droop. This phenomenon was presented in a notable study conducted by Luo et al. [Citation299] which observed that by subjecting a flexible LED to just 1% compressive strain, a remarkable 14.2% increase in peak EQE and a significant 17.9% reduction in efficiency droop was achieved. In this context, Kochetkov et al. successfully demonstrated a transparent and strain-insensitive InGaN/GaN core-shell NW µ-LED through a two-step process involving the growth of InGaN/GaN core-shell NWs using MOCVD on a PDMS substrate, followed by a picking and placing procedure, as shown in Figure a. This innovative LED design incorporates SWCNT electrodes and vertical nitride microwire arrays stretched over a PDMS membrane, enabling stretchability. The electroluminescence (EL) signal shows only a slight reduction of less than 15% after 20 stretching cycles, highlighting its capability for repeated stretching, as shown in Figure b. Furthermore, by utilizing SWCNT pads on a stretched PDMS/MW membrane, stable stretch electrical contact is achieved, ensuring consistent performance [Citation287].
Figure 32. (a) The schematic fabrication process workflow of a PDMS/MW membrane, the process involves: (i) fixing the SWCNT contact pads onto the PDMS/MW membrane, (ii) connecting the SWCNT contact pads with copper wires and silver lacquer, (iii) establishing electrical contact to the bottom side of the PDMS/MW membrane, and (iv) burying the relaxed LED device into the PDMS. (B) The figure also presents the working voltage during the stretching test of the LED at a constant injection current. Figures reproduced from Ref. [Citation287], under the terms of an open access Creative Commons CC BY 4.0 license.
![Figure 32. (a) The schematic fabrication process workflow of a PDMS/MW membrane, the process involves: (i) fixing the SWCNT contact pads onto the PDMS/MW membrane, (ii) connecting the SWCNT contact pads with copper wires and silver lacquer, (iii) establishing electrical contact to the bottom side of the PDMS/MW membrane, and (iv) burying the relaxed LED device into the PDMS. (B) The figure also presents the working voltage during the stretching test of the LED at a constant injection current. Figures reproduced from Ref. [Citation287], under the terms of an open access Creative Commons CC BY 4.0 license.](/cms/asset/4930a527-9970-4741-a4a2-a3d7e23eb37c/tjid_a_2282937_f0032_oc.jpg)
Jeong et al. [Citation284] achieved a successful fabrication of a flexible NW LED through the application of a remote heteroepitaxy. They employed GaN NW p-n junction arrays on a c-Al2O3 wafer, which was connected to graphene. Figure illustrates the fabrication, where the NW LED arrays were transferred from the c-Al2O3 wafer to a graphene substrate. Using graphene facilitated the transfer of the NW LED arrays onto a copper plate. The spatially separated NW arrays allowed for versatile deformable NW LEDs in different shapes while maintaining device performance without significant degradation. Furthermore, employing this methodology empowers us to attain reusability, thereby ensuring reproducibility through the utilization of a single substrate without any noticeable degradation in performance. The fabricated NW LED device exhibited I-V characteristics with an electrical threshold of ∼ 4 V and a leakage current of ∼ 5 × 10−3 A at −5 V. Additionally, the full width at half maximum (FWHM) of the electroluminescence (EL) spectra was slightly greater for the NW LED in comparison to the thin film LED, suggesting the necessity for additional enhancements in this particular area.
Figure 33. (a) Schematic demonstration of the fabrication process of a flexible and deformable LED using heteroepitaxial NW arrays on a metal plate; (b) Performance analysis of the fabricated LED device (λ = 500 nm) in various configurations such as twisted, 90°-folded crushed, and 180°-folded shapes, operated at 100 mA. (c) The collection consists of images showcasing blue LED (λ = 450 nm) placed on different surfaces, such as a pen and the slim edge of a plastic box, at a current of 100 mA; (d) The images displayed depict miniature LED lights measuring 10 mm by 10 mm, at a wavelength of 450 nm. These LED lights are specifically designed to be attached to the back legs of a LEGO minifigure, as shown in the left two panels. The right three panels showcase the same LEGO minifigure with various leg postures, each with LED lights affixed to them. During the experiments, the LED lights were operated at a current of 100 mA. Figures reproduced with permission from Ref. [Citation284], Copyright © 2020, Science.
![Figure 33. (a) Schematic demonstration of the fabrication process of a flexible and deformable LED using heteroepitaxial NW arrays on a metal plate; (b) Performance analysis of the fabricated LED device (λ = 500 nm) in various configurations such as twisted, 90°-folded crushed, and 180°-folded shapes, operated at 100 mA. (c) The collection consists of images showcasing blue LED (λ = 450 nm) placed on different surfaces, such as a pen and the slim edge of a plastic box, at a current of 100 mA; (d) The images displayed depict miniature LED lights measuring 10 mm by 10 mm, at a wavelength of 450 nm. These LED lights are specifically designed to be attached to the back legs of a LEGO minifigure, as shown in the left two panels. The right three panels showcase the same LEGO minifigure with various leg postures, each with LED lights affixed to them. During the experiments, the LED lights were operated at a current of 100 mA. Figures reproduced with permission from Ref. [Citation284], Copyright © 2020, Science.](/cms/asset/ac7b4c98-490d-4fbc-a517-5a36ac0a95ef/tjid_a_2282937_f0033_oc.jpg)
3.8. Summary and feature outlook of this discussion
In summary, this paper highlights the remarkable advancements in full-color InGaN-based micro LEDs, including improved emission efficiency, miniaturization, color uniformity, loss management, and accuracy. This collective information can help us open up exciting possibilities for high-resolution displays and various applications. The continuous advancement of this technology will pave the way for innovative and immersive visual experiences. Further research and development efforts are needed to address the remaining challenges and drive the adoption of full-color InGaN-based micro LEDs in the consumer electronics market.
In reality, a potential future aims to push the boundaries by enhancing these device capabilities through chip-scale integration to further expand the performance GaN-based device gamut. Advancements are ceaseless. To enhance performance or provide new functionality, numerous strategies – typically categorized as monolithic or multifaceted – have been devised [Citation100,Citation300]. Continued research and advancements in materials, device fabrication, and manufacturing techniques are expected to lead to further improvements in performance, scalability, and cost-effectiveness. These displays have the potential to revolutionize the consumer electronics industry by offering displays with exceptional image quality, low power consumption, and flexible form factors.
The prime ongoing research is the development of large-scale, high-resolution displays using III-nitride NW technology. Efforts are focused on achieving seamless integration of NWs on large-area substrates while maintaining uniformity and high yield. Additionally, the optimization of NW materials and device structures is being explored to enhance efficiency, color gamut, and reliability. The next level of exploration is the integration of NW displays with emerging technologies such as augmented reality (AR) and virtual reality (VR). The small pixel sizes and high pixel density of NW displays make them ideal candidates for creating immersive visual experiences in these applications. Efforts are underway to develop efficient fabrication processes and address the challenges of scalability to enable the widespread adoption of NW-based displays in AR/VR systems.
Consequently, to achieve an extensive production of flexible III-nitride nanocolumn photonic devices, it is crucial to uncover an affordable technique for generating high-quality materials on a large scale. These materials must be capable of being seamlessly integrated into devices, exhibiting performance comparable to that of conventional epilayers. The swift and successful resolution of these challenges, along with the advancement of complementary photonic components such as lasers and photodetectors, could potentially lead to the replacement of flexible integrated photonics by III-nitride NWs.
Conflict of interest
Some IP related to the NW work at McGill University and the University of Michigan has been licensed to NS Nanotech, Inc. and NX Fuels, Inc., which were co-founded by Z. Mi. The University of Michigan and Mi have a financial interest in the company.
Disclosure statement
No potential conflict of interest was reported by the author(s).
Additional information
Funding
Notes on contributors

Veeramuthu Vignesh
Veeramuthu Vignesh received his PhD degree from vellore institute of technology (VIT), Chennai, Tamilnadu, India in 2020. He currently holds a position as a post-doctoral researcher in Research Center for Advanced Materials Development (RCAMD) at Jeonbuk National University (JBNU), South Korea. His research revolves around the intriguing realm of nanostructure materials, with a primary focus on energy storage and conversion technologies such as supercapacitors, batteries, water-splitting, as well as applications in optoelectronic devices like LEDs and LDs.

Yuanpeng Wu
Yuanpeng Wu is currently a Research Investigator at the Department of Electrical and Computer Engineering at the University of Michigan, Ann Arbor. He received his BS degree from Shanxi University in 2012 and M.Eng. degree from Zhejiang University in 2015 in China. His current research interests include synthesis and characterizations of III-V wide bandgap semiconductors, simulation and fabrication of electronic and optoelectronic devices such as LEDs, lasers, quantum light sources, and memory devices.

Sung-Un Kim
Sung-Un Kim is currently working on his Ph.D. under the supervision of Prof. Yong-Ho Ra in the Division of Advanced Materials Engineering of Jeonbuk National University (JBNU), Republic of Korea. His primary research endeavors revolve around exploring the fascinating realm of low-dimensional semiconductors, photonic and electronic devices, encompassing a range of technologies, such as LED, LD, and display optics.

Jeong-Kyun Oh
Jeong-Kyun Oh is currently working towards his Ph.D. at Jeonbuk National University (JBNU), Republic of Korea, under the guidance of Prof. Yong-Ho Ra in the Division of Advanced Materials Engineering. His research focuses on the captivating field of low-dimensional semiconductors and delves into various technologies like LED, LD, display optics and water splitting (Hydrogen generation).

Chandran Bagavath
Chandran Bagavath received his PhD degree from Crystal Growth Centre, Anna University, Chennai, Tamilnadu, India in 2019. He currently holds a position as a post-docctoral researcher at Jeonbuk National University (JBNU), South Korea, under the guidance of Prof. Yong-Ho Ra in the Division of Advanced Materials Engineering. His research focuses on the captivating field of semiconductor materials for microelectronic, optoelectronic and Energy conversion applications.

Dae-Young Um
Dae-Young Um currently holds a position as a Research Professor in the Division of Advanced Materials Engineering at Jeonbuk National University (JBNU), Republic of Korea. He received his PhD degree under the supervision of Prof. Cheul-Ro Lee from JBNU in 2021. His primary research interests are in the fields of low-dimensional nanostructures-based semiconductors and their applications in optoelectronic, electronic, clean energy, and nano devices like LED, LD, Photodetector, Photoelectrochemical Water Splitting, Solar Cell, and Transistor.

Zetian Mi
Zetian Mi is a Professor at the University of Michigan. His teaching and research interests are in the areas of low dimensional semiconductors and their applications in photonic, electronic, clean energy, and quantum devices. He has received the Science and Engineering Award from W. M. Keck Foundation, the David E. Liddle Research Excellence Award, and the IEEE Photonics Society Distinguished Lecturer Award. He currently serves as the Editor-in-Chief of Progress in Quantum Electronics, Serial Editor of Semiconductors and Semimetals, and Vice President for Conferences of IEEE Photonics Society. He is a fellow of IEEE, OSA, and SPIE, and co-founder of NS Nanotech, Inc. and NX Fuels, Inc.

Yong-Ho Ra
Yong-Ho Ra is an assistant professor in the Division of Advanced Materials Engineering of Jeonbuk National University (JBNU), Republic of Korea. His teaching and research interests revolve around epitaxial growth/synthesis and characterizations of III-nitride-based nanowire/-rod, quantum dot, and thin film heterostructures. His work encompasses both fundamental material studies and their applications in photonic, electronic, and energy devices.
References
- T. Wu, C.W. Sher, Y. Lin, C.F. Lee, S. Liang, Y. Lu, S.W.H. Chen, W. Guo, H.C. Kuo, and Z. Chen, Appl. Sci. 8, 1557 (2018).
- J. Day, J. Li, D.Y.C. Lie, C. Bradford, J.Y. Lin, and H.X. Jiang, in Quantum Sens. Nanophotonic Devices IX, edited by M. Razeghi, E. Tournie, and G.J. Brown (2012), p. 82681X.
- J. El Ryu, S. Park, Y. Park, S.W. Ryu, K. Hwang, and H.W. Jang, Adv. Mater. 2204947 (2023).
- J.J. Wierer, and N. Tansu, Laser Photonics Rev. 13, 1900141 (2019).
- S.S. Konoplev, K.A. Bulashevich, and S.Y. Karpov, Phys. Status Solidi. 215, 1700508 (2018).
- H.W. Choi, C.W. Jeon, M.D. Dawson, P.R. Edwards, R.W. Martin, and S. Tripathy, J. Appl. Phys. 93, 5978 (2003).
- H. Jiang, and J. Lin, Nat. Electron. 6, 257 (2023).
- C.W. Tang, and S.A. VanSlyke, Appl. Phys. Lett. 51, 913 (1987).
- C. Adachi, Jpn. J. Appl. Phys. 53, 060101 (2014).
- Y. Matsumoto, and Y. Takaki, J. Soc. Inf. Disp. 25, 515 (2017).
- D. Nakamura, H. Ikeda, N. Sugisawa, Y. Yanagisawa, S. Eguchi, S. Kawashima, M. Shiokawa, H. Miyake, S. Idojiri, A. Ishii, M. Yokoyama, Y. Hirakata, and S. Yamazaki, J. Soc. Inf. Disp. 23, 464 (2015).
- T. Tsujimura, OLED Display Fundamentals and Applications (John Wiley & Sons, Inc, Hoboken, New Jersey, 2017).
- S.-H. Kim, M.-Y. Lee, K. Woo, H. Youn, T.-M. Lee, E.K. Lee, and S. Kwon, Int. J. Precis. Eng. Manuf. 18, 1111 (2017).
- T.Y. Lee, L.Y. Chen, Y.Y. Lo, S.S. Swayamprabha, A. Kumar, Y.M. Huang, S.C. Chen, H.W. Zan, F.C. Chen, R.H. Horng, and H.C. Kuo, ACS Photonics. 9, 2905 (2022).
- O. Ambacher, J. Phys. D. Appl. Phys. 31, 2653 (1998).
- N. Alfaraj, M.M. Muhammed, K.H. Li, B. Janjua, R.A. Aljefri, H. Sun, T.K. Ng, B.S. Ooi, I.S. Roqan, and X. Li, AIP Adv. 7, 125113 (2017).
- C. Hahn, Z. Zhang, A. Fu, C.H. Wu, Y.J. Hwang, D.J. Gargas, and P. Yang, ACS Nano. 5, 3970 (2011).
- T. Kuykendall, P. Ulrich, S. Aloni, and P. Yang, Nat. Mater. 6, 951 (2007).
- S.-U. Kim, J.-K. Oh, D.-Y. Um, B. Chandran, C.-R. Lee, and Y.-H. Ra, IEEE Photonics J. 15, 1 (2023).
- Y.J. Hong, C.-H. Lee, A. Yoon, M. Kim, H.-K. Seong, H.J. Chung, C. Sone, Y.J. Park, and G.-C. Yi, Adv. Mater. 23, 3284 (2011).
- S. Nakamura, Science (80-.). 281, 956 (1998).
- M.R. Krames, O.B. Shchekin, R. Mueller-Mach, G.O. Mueller, L. Zhou, G. Harbers, and M.G. Craford, IEEE/OSA J. Disp. Technol. 3, 160 (2007).
- Z. Zhuang, D. Iida, and K. Ohkawa, Jpn. J. Appl. Phys. 61, SA0809 (2022).
- J. Cui, J. Zhou, and H. Xiao, J. Alloys Compd. 924, 166567 (2022).
- M.F. Huang, Y.L. Huang, J.Y. Chang, Y.H. Shih, and Y.K. Kuo, Micro Nanostructures. 167, 207202 (2022).
- S. Cheng, Z. Wu, B. Langelier, X. Kong, T. Coenen, S. Hari, Y. Ra, R.T. Rashid, A. Pofelski, H. Yuan, X. Li, Z. Mi, H. Guo, and G.A. Botton, Adv. Opt. Mater. 8, 2000481 (2020).
- S. Li, and A. Waag, J. Appl. Phys. 111, 071101 (2012).
- K.C. Kim, M.C. Schmidt, H. Sato, F. Wu, N. Fellows, M. Saito, K. Fujito, J.S. Speck, S. Nakamura, and S.P. DenBaars, Phys. Status Solidi - Rapid Res. Lett. 1, 125 (2007).
- H.-J. Shih, I. Lo, Y.-C. Wang, C.-D. Tsai, Y.-C. Lin, Y.-Y. Lu, and H.-C. Huang, Crystals. 12, 417 (2022).
- H.P. Maruska, and J.J. Tietjen, Appl. Phys. Lett. 15, 327 (1969).
- A.D. Bolshakov, V.V. Fedorov, K.Y. Shugurov, A.M. Mozharov, G.A. Sapunov, I.V. Shtrom, M.S. Mukhin, A.V. Uvarov, G.E. Cirlin, and I.S. Mukhin, Nanotechnology 30, 395602 (2019).
- O. Landré, V. Fellmann, P. Jaffrennou, C. Bougerol, H. Renevier, and B. Daudin, Phys. Status Solidi C. 7, 2246 (2010).
- K.A. Bertness, A. Roshko, L.M. Mansfield, T.E. Harvey, and N.A. Sanford, J. Cryst. Growth. 300, 94 (2007).
- X. Liu, Y. Wu, Y. Malhotra, Y. Sun, Y.H. Ra, R. Wang, M. Stevenson, S. Coe-Sullivan, and Z. Mi, J. Soc. Inf. Disp. 28, 410 (2020).
- J. Kamimura, P. Bogdanoff, M. Ramsteiner, P. Corfdir, F. Feix, L. Geelhaar, and H. Riechert, Nano Lett. 17, 1529 (2017).
- J. Chai, Q. Liu, L. Chen, B. Cao, D. Kong, T. Lin, W. Wang, and G. Li, Adv. Electron. Mater. 9, 2201193 (2023).
- Y. Wu, B. Liu, Z. Li, T. Tao, Z. Xie, K. Wang, X. Xiu, D. Chen, H. Lu, R. Zhang, and Y. Zheng, Phys. Status Solidi Appl. Mater. Sci. 217, 1900729 (2020).
- T. Tabata, J. Paek, Y. Honda, M. Yamaguchi, and H. Amano, Phys. Status Solidi Curr. Top. Solid State Phys. 9, 646 (2012).
- S. Zhao, R. Wang, S. Chu, and Z. Mi, IEEE Nanotechnol. Mag. 13 (6, (2019).
- S.-U. Kim, and Y.-H. Ra, Nanomaterials 11, 9 (2020).
- Y.H. Ra, and C.R. Lee, Adv. Mater. Technol. 6, 2000885 (2021).
- C.T. Foxon, S.V. Novikov, J.L. Hall, R.P. Campion, D. Cherns, I. Griffiths, and S. Khongphetsak, J. Cryst. Growth. 311, 3423 (2009).
- B.G. Hagar, M. Abdelhamid, E.L. Routh, P.C. Colter, and S.M. Bedair, Appl. Phys. Lett. 121, 052104 (2022).
- A.K. Tan, N.A. Hamzah, M.A. Ahmad, S.S. Ng, and Z. Hassan, Mater. Sci. Semicond. Process. 143, 106545 (2022).
- M.I. Md Taib, M.A. Ahmad, E.A. Alias, A.I. Alhassan, I.A. Ajia, M.M. Muhammed, I.S. Roqan, S.P. DenBaars, J.S. Speck, S. Nakamura, and N. Zainal, Semicond. Sci. Technol. 38, 035025 (2023).
- G. Li, P. Wang, X. He, Y. Meng, F. Liang, M. Zhou, and D. Zhao, Mater. Res. Express. 9, 066404 (2022).
- D. Kim, K.M. Song, U.J. Jung, S. Kim, D.S. Shin, and J. Park, Appl. Sci. 10 (2020).
- M.A. Johar, H.G. Song, A. Waseem, J.H. Kang, J.S. Ha, Y.H. Cho, and S.W. Ryu, Nanoscale 11, 10932 (2019).
- Y.H. Ra, R. Navamathavan, J.H. Park, and C.R. Lee, ACS Appl. Mater. Interfaces 5, 2111 (2013).
- Y.-H. Ra, R. Navamathavan, and C.-R. Lee, CrystEngComm. 14, 8208 (2012).
- Y. Ra, R. Navamathavan, J. Park, and C. Lee (2013).
- Z. Wang, S. Zhu, X. Shan, Z. Yuan, X. Cui, and P. Tian, Opt. Lett. 46, 4358 (2021).
- S. Zhao, H.P.T. Nguyen, M.G. Kibria, and Z. Mi, Prog. Quantum Electron. 44, 14 (2015).
- R. Raj, V. Vignesh, Y.-H. Ra, R. Nirmala, C.-R. Lee, and R. Navamathavan, J. Photonics Energy 7, 016001 (2017).
- S. Nakamura, T. Mukai, and M. Senoh, Appl. Phys. Lett. 64, 1687 (1994).
- T. Onuma, S. Keller, S.P. DenBaars, J.S. Speck, S. Nakamura, U.K. Mishra, T. Sota, and S.F. Chichibu, Appl. Phys. Lett. 88, 111912 (2006).
- Y. Xi, J.Q. Xi, T. Gessmann, J.M. Shah, J.K. Kim, E.F. Schubert, A.J. Fischer, M.H. Crawford, K.H.A. Bogart, and A.A. Allerman, Appl. Phys. Lett. 86, 1 (2005).
- Y. Wu, X. Liu, A. Pandey, P. Zhou, W.J. Dong, P. Wang, J. Min, P. Deotare, M. Kira, E. Kioupakis, and Z. Mi, Prog. Quantum Electron. 85, 100401 (2022).
- P. Feng, C. Xu, J. Bai, C. Zhu, I. Farrer, G.M. De Arriba, and T. Wang, ACS Appl. Electron. Mater. 4, 2787 (2022).
- B. Park, J.K. Lee, C.T. Koch, M. Wölz, L. Geelhaar, and S.H. Oh, Adv. Sci. 9, 2200323 (2022).
- F. Glas, Phys. Rev. B - Condens. Matter Mater. Phys. 74, 121302 (2006).
- C. Zhao, N. Alfaraj, R. Chandra Subedi, J.W. Liang, A.A. Alatawi, A.A. Alhamoud, M. Ebaid, M.S. Alias, T.K. Ng, and B.S. Ooi, Prog. Quantum Electron. 61, 1 (2018).
- R.M. Farrell, E.C. Young, F. Wu, S.P. Denbaars, and J.S. Speck, Semicond. Sci. Technol. 27, 024001 (2012).
- J. Moneta, G. Staszczak, E. Grzanka, P. Tauzowski, P. Dłużewski, and J. Smalc-Koziorowska, J. Appl. Phys. 133, 045304 (2023).
- C. Li, Y. Piao, B. Meng, Y. Hu, L. Li, and F. Zhang, Int. J. Mach. Tools Manuf. 172, 103827 (2022).
- C. Haller, J.F. Carlin, G. Jacopin, W. Liu, D. Martin, R. Butté, and N. Grandjean, Appl. Phys. Lett. 113 (2018).
- F.C.P. Massabuau, S.L. Sahonta, L. Trinh-Xuan, S. Rhode, T.J. Puchtler, M.J. Kappers, C.J. Humphreys, and R.A. Oliver, Appl. Phys. Lett. 101, 212107 (2012).
- A.E. Romanov, E.C. Young, F. Wu, A. Tyagi, C.S. Gallinat, S. Nakamura, S.P. Denbaars, and J.S. Speck, J. Appl. Phys. 109, 103522 (2011).
- Y. Jiang, Y. Li, Y. Li, Z. Deng, T. Lu, Z. Ma, P. Zuo, L. Dai, L. Wang, H. Jia, W. Wang, J. Zhou, W. Liu, and H. Chen, Sci. Rep. 5, 10883 (2015).
- S. Hammersley, M.J. Kappers, F.C.P. Massabuau, S.L. Sahonta, P. Dawson, R.A. Oliver, and C.J. Humphreys, Appl. Phys. Lett. 107 (2015).
- Z. Lv, H. Wang, and H. Jiang, J. Phys. Chem. C. 125, 16643 (2021).
- X. Zhao, K. Sun, S. Cui, B. Tang, H. Hu, and S. Zhou, Adv. Photonics Res. (2023).
- H.S. Wasisto, J.D. Prades, J. Gülink, and A. Waag, Appl. Phys. Rev. 6, 041315 (2019).
- J.H. Moon, B. Kim, M. Choi, K.Y. Woo, B.S. Kim, S. Ahn, S. Jun, Y.H. Song, and Y.H. Cho, Adv. Mater. 35 (2023).
- J. Zhang, L.D. Zhang, X.F. Wang, C.H. Liang, X.S. Peng, and Y.W. Wang, J. Chem. Phys. 115, 5714 (2001).
- R. Wang, H.P.T. Nguyen, A.T. Connie, J. Lee, I. Shih, and Z. Mi, Opt. Express. 22, A1768 (2014).
- A. Pandey, Y. Malhotra, P. Wang, K. Sun, X. Liu, and Z. Mi, Photonics Res. 10, 1107 (2022).
- Y.H. Ra, R. Wang, S.Y. Woo, M. Djavid, S.M. Sadaf, J. Lee, G.A. Botton, and Z. Mi, Nano Lett. 16, 4608 (2016).
- C. Mazuir, J. Nanophotonics 1, 013503 (2007).
- Y.H. Ra, R. Navamathavan, H. Il Yoo, and C.R. Lee, Nano Lett. 14, 1537 (2014).
- H.P.T. Nguyen, S. Zhang, K. Cui, X. Han, S. Fathololoumi, M. Couillard, G.A. Botton, and Z. Mi, Nano Lett. 11, 1919 (2011).
- H. Sun, and X. Li, Phys. Status Solidi Appl. Mater. Sci. 216, 1800420 (2019).
- A. Waag, X. Wang, S. Fündling, J. Ledig, M. Erenburg, R. Neumann, M. Al Suleiman, S. Merzsch, J. Wei, S. Li, H.H. Wehmann, W. Bergbauer, M. Straßburg, A. Trampert, U. Jahn, and H. Riechert, Phys. Status Solidi Curr. Top. Solid State Phys. 8, 2296 (2011).
- M. Sheen, Y. Ko, D. Kim, J. Kim, J. Byun, Y. Choi, J. Ha, K.Y. Yeon, D. Kim, J. Jung, J. Choi, R. Kim, J. Yoo, I. Kim, C. Joo, N. Hong, J. Lee, S.H. Jeon, S.H. Oh, J. Lee, N. Ahn, and C. Lee, Nature. 608, 56 (2022).
- X. Liu, Y. Sun, Y. Malhotra, A. Pandey, P. Wang, Y. Wu, K. Sun, and Z. Mi, Photonics Res. 10, 587 (2022).
- M.M. Taher, S. Al-yousif, and N.M. Ahmed, Results Phys. 20, 103732 (2021).
- C. Li, J.B. Wright, S. Liu, P. Lu, J.J. Figiel, B. Leung, W.W. Chow, I. Brener, D.D. Koleske, T.S. Luk, D.F. Feezell, S.R.J. Brueck, and G.T. Wang, Nano Lett. 17, 1049 (2017).
- D. Kong, Y. Zhou, J. Chai, S. Chen, L. Chen, L. Li, T. Lin, W. Wang, and G. Li, J. Mater. Chem. C. 10, 14080 (2022).
- J.H. Park, R. Nandi, J.K. Sim, D.Y. Um, S. Kang, J.S. Kim, and C.R. Lee, RSC Adv. 8, 20585 (2018).
- H.P.T. Nguyen, Y. Li, and Z. Mi, in CLEO Sci. Innov. CLEO_SI 2012 (OSA, Washington, D.C., 2012), p. CF2J.6.
- R. Cheriton, S.M. Sadaf, L. Robichaud, J.J. Krich, Z. Mi, and K. Hinzer, Commun. Mater. 1, 63 (2020).
- S. Noh, J. Shin, Y.T. Yu, M.Y. Ryu, and J.S. Kim, Nanomaterials 13, 358 (2023).
- M.G. Kibria, F.A. Chowdhury, S. Zhao, B. AlOtaibi, M.L. Trudeau, H. Guo, and Z. Mi, Nat. Commun. 6, 6797 (2015).
- H.J. Joyce, Q. Gao, H. Hoe Tan, C. Jagadish, Y. Kim, J. Zou, L.M. Smith, H.E. Jackson, J.M. Yarrison-Rice, P. Parkinson, and M.B. Johnston, Prog. Quantum Electron. 35, 23 (2011).
- A. Zhang, G. Zheng, and C.M. Lieber, Nanosci. Technol. 15–37 (2016).
- J. Xiong, E.L. Hsiang, Z. He, T. Zhan, and S.T. Wu, Light Sci. Appl. 10, 216 (2021).
- Z. Liu, C.H. Lin, B.R. Hyun, C.W. Sher, Z. Lv, B. Luo, F. Jiang, T. Wu, C.H. Ho, H.C. Kuo, and J.H. He, Light Sci. Appl. 9, 83 (2020).
- D. Hwang, A. Mughal, C.D. Pynn, S. Nakamura, and S.P. DenBaars, Appl. Phys. Express 10, 032101 (2017).
- J.M. Smith, R. Ley, M.S. Wong, Y.H. Baek, J.H. Kang, C.H. Kim, M.J. Gordon, S. Nakamura, J.S. Speck, and S.P. Denbaars, Appl. Phys. Lett. 116 (2020).
- W.Y. Fu, and H.W. Choi, Phys. Status Solidi - Rapid Res. Lett. 16, 2100628 (2022).
- W.Y. Fu, K.K.Y. Wong, and H.W. Choi, Appl. Phys. Lett. 95 (2009).
- C. Böcklin, R.G. Veprek, S. Steiger, and B. Witzigmann, Phys. Rev. B - Condens. Matter Mater. Phys. 81, 155306 (2010).
- Y.D. Zhuang, J. Bruckbauer, P.A. Shields, P.R. Edwards, R.W. Martin, and D.W.E. Allsopp, J. Appl. Phys. 116 (2014).
- C. Feng, J.A. Huang, and H.W. Choi, ACS Photonics. 3, 1294 (2016).
- W.Y. Fu, and H.W. Choi, Optica. 5, 765 (2018).
- W.Y. Fu, and H.W. Choi, Appl. Phys. Lett. 118 (2021).
- V. Ramesh, A. Kikuchi, K. Kishino, M. Funato, and Y. Kawakami, J. Appl. Phys. 107 (2010).
- R.S. Wagner, and W.C. Ellis, Appl. Phys. Lett. 4, 89 (1964).
- Y.B. Tang, X.H. Bo, C.S. Lee, H.T. Cong, H.M. Cheng, Z.H. Chen, W.J. Zhang, I. Bello, and S.T. Lee, Adv. Funct. Mater. 18, 3515 (2008).
- X. Weng, R.A. Burke, and J.M. Redwing, Nanotechnology 20, 085610 (2009).
- G. Seryogin, I. Shalish, W. Moberlychan, and V. Narayanamurti, Nanotechnology 16, 2342 (2005).
- A. Bao, Mater. Sci. Technol. 33, 765 (2017).
- H.J. Fan, P. Werner, and M. Zacharias, Small 2, 700 (2006).
- J. Li, C. Lu, B. Maynor, S. Huang, and J. Liu, Chem. Mater. 16, 1633 (2004).
- F. Qian, Y. Li, S. Gradečak, D. Wang, C.J. Barrelet, and C.M. Lieber, Nano Lett. 4, 1975 (2004).
- M.A. Sanchez-Garcia, E. Calleja, E. Monroy, F.J. Sanchez, F. Calle, E. Muñoz, and R. Beresford, J. Cryst. Growth 183, 23 (1998).
- E.S. Jang, Y.H. Ra, Y.M. Lee, S.H. Yun, D.W. Kim, R. Navamathavan, J.S. Kim, I.H. Lee, and C.R. Lee, Jpn. J. Appl. Phys. 48, 0910011 (2009).
- J. Ristić, E. Calleja, S. Fernández-Garrido, L. Cerutti, A. Trampert, U. Jahn, and K.H. Ploog, J. Cryst. Growth. 310, 4035 (2008).
- M. Yoshizawa, A. Kikuchi, N. Fujita, K. Kushi, H. Sasamoto, and K. Kishino, J. Cryst. Growth. 189–190, 138 (1998).
- K.A. Bertness, A. Roshko, N.A. Sanford, J.M. Barker, and A.V. Davydov, J. Cryst. Growth. 287, 522 (2006).
- O. Landré, C. Bougerol, H. Renevier, and B. Daudin, Nanotechnology 20, 415602 (2009).
- R. Songmuang, O. Landré, and B. Daudin, Appl. Phys. Lett. 91 (2007).
- H. Sekiguchi, T. Nakazato, A. Kikuchi, and K. Kishino, J. Cryst. Growth. 300, 259 (2007).
- V. Consonni, M. Knelangen, L. Geelhaar, A. Trampert, and H. Riechert, Phys. Rev. B - Condens. Matter Mater. Phys. 81, 085310 (2010).
- R.K. Debnath, R. Meijers, T. Richter, T. Stoica, R. Calarco, and H. Lüth, Appl. Phys. Lett. 90 (2007).
- K.A. Bertness, A. Roshko, L.M. Mansfield, T.E. Harvey, and N.A. Sanford, J. Cryst. Growth 310, 3154 (2008).
- E. Galopin, L. Largeau, G. Patriarche, L. Travers, F. Glas, and J.C. Harmand, Nanotechnology 22, 245606 (2011).
- K. Kishino, H. Sekiguchi, and A. Kikuchi, J. Cryst. Growth 311, 2063 (2009).
- A. Usui, H. Sunakawa, A. Sakai, and A.A. Yamaguchi, Japanese J. Appl. Physics, Part 2 Lett. 36, L899 (1997).
- Y.H. Ra, R.T. Rashid, X. Liu, J. Lee, and Z. Mi, Adv. Funct. Mater. 27 (2017).
- H.Q.T. Bui, R.T. Velpula, B. Jain, O.H. Aref, H.D. Nguyen, T.R. Lenka, and H.P.T. Nguyen, Micromachines 10, 492 (2019).
- V. Consonni, M. Hanke, M. Knelangen, L. Geelhaar, A. Trampert, and H. Riechert, Phys. Rev. B - Condens. Matter Mater. Phys. 83, 035310 (2011).
- B.J. May, A.T.M.G. Sarwar, and R.C. Myers, Appl. Phys. Lett. 108 (2016).
- C. Zhao, T.K. Ng, N. Wei, A. Prabaswara, M.S. Alias, B. Janjua, C. Shen, and B.S. Ooi, Nano Lett. 16, 1056 (2016).
- S. Zhao, M.G. Kibria, Q. Wang, H.P.T. Nguyen, and Z. Mi, Nanoscale 5, 5283 (2013).
- M.R. Philip, D.D. Choudhary, M. Djavid, K.Q. Le, J. Piao, and H.P.T. Nguyen, J. Sci. Adv. Mater. Devices 2, 150 (2017).
- Z. Gong, S. Jin, Y. Chen, J. McKendry, D. Massoubre, I.M. Watson, E. Gu, and M.D. Dawson, J. Appl. Phys. 107, 013103 (2010).
- R. Wang, X. Liu, I. Shih, and Z. Mi, Appl. Phys. Lett. 106, 261104 (2015).
- M.G. Kibria, S. Zhao, F.A. Chowdhury, Q. Wang, H.P.T. Nguyen, M.L. Trudeau, H. Guo, and Z. Mi, Nat. Commun. 5, 3825 (2014).
- G. Li, Y. Yao, and M. Dagenais, J. Cryst. Growth 524, 125181 (2019).
- X. Wang, S. Li, M.S. Mohajerani, J. Ledig, H.-H. Wehmann, M. Mandl, M. Strassburg, U. Steegmüller, U. Jahn, J. Lähnemann, H. Riechert, I. Griffiths, D. Cherns, and A. Waag, Cryst. Growth Des. 13, 3475 (2013).
- Ž Gačević, D. Gómez Sánchez, and E. Calleja, Nano Lett. 15, 1117 (2015).
- F. Qian, S. Gradečak, Y. Li, C.Y. Wen, and C.M. Lieber, Nano Lett. 5, 2287 (2005).
- Y.H. Ra, and C.R. Lee, Nano Lett. 20, 4162 (2020).
- R. Navamathavan, V. Vignesh, R. Nirmala, and R. Raj, Adv. Sci. Eng. Med. 8, 925 (2016).
- M. Tchernycheva, P. Lavenus, H. Zhang, A.V. Babichev, G. Jacopin, M. Shahmohammadi, F.H. Julien, R. Ciechonski, G. Vescovi, and O. Kryliouk, Nano Lett. 14, 2456 (2014).
- O. Hayden, A.B. Greytak, and D.C. Bell, Adv. Mater. 17, 701 (2005).
- S. Choi, H.G. Song, Y.S. Yoo, C. Lee, K.Y. Woo, E. Lee, S.D. Roh, and Y.H. Cho, ACS Photonics 5, 2825 (2018).
- S. Brochen, J. Brault, S. Chenot, A. Dussaigne, M. Leroux, and B. Damilano, Appl. Phys. Lett. 103 (2013).
- U. Kaufmann, P. Schlotter, H. Obloh, K. Köhler, and M. Maier, Phys. Rev. B - Condens. Matter Mater. Phys. 62, 10867 (2000).
- G. Miceli, and A. Pasquarello, Phys. Rev. B. 93, 165207 (2016).
- G. Namkoong, W.A. Doolittle, and A.S. Brown, Appl. Phys. Lett. 77, 4386 (2000).
- S. Pezzagna, P. Vennéguès, N. Grandjean, and J. Massies, J. Cryst. Growth 269, 249 (2004).
- J. Piprek, and S. Li, Opt. Quantum Electron. 42, 89 (2010).
- H.Y. Ryu, J.I. Shim, C.H. Kim, J.H. Choi, H.M. Jung, M.S. Noh, J.M. Lee, and E.S. Nam, IEEE Photonics Technol. Lett. 23, 1866 (2011).
- J. Cho, E.F. Schubert, and J.K. Kim, Laser Photonics Rev. 7, 408 (2013).
- F. Furtmayr, M. Vielemeyer, M. Stutzmann, J. Arbiol, S. Estradé, F. Peir, J.R. Morante, and M. Eickhoff, J. Appl. Phys. 104, 034309 (2008).
- Y.H. Ra, and C.R. Lee, ACS Photonics 6, 2397 (2019).
- Q. Wang, X. Liu, M.G. Kibria, S. Zhao, H.P.T. Nguyen, K.H. Li, Z. Mi, T. Gonzalez, and M.P. Andrews, Nanoscale 6, 9970 (2014).
- A. Pandey, Y. Xiao, M. Reddeppa, Y. Malhotra, J. Liu, J. Min, Y. Wu, and Z. Mi, Appl. Phys. Lett. 122, 151103 (2023).
- B. Liu, T. Hu, Z. Wang, L. Liu, F. Qin, N. Huang, and X. Jiang, Cryst. Res. Technol. 47, 207 (2012).
- M.A. Reshchikov, and H. Morkoç, J. Appl. Phys. 97, 061301 (2005).
- C. Kölper, M. Sabathil, F. Römer, M. Mandl, M. Strassburg, and B. Witzigmann, Phys. Status Solidi. 209, 2304 (2012).
- S. Li, X. Wang, S. Fündling, M. Erenburg, J. Ledig, J. Wei, H.H. Wehmann, A. Waag, W. Bergbauer, M. Mandl, M. Strassburg, A. Trampert, U. Jahn, H. Riechert, H. Jönen, and A. Hangleiter, Appl. Phys. Lett. 101 (2012).
- J. Bai, Q. Wang, and T. Wang, J. Appl. Phys. 111, 113103 (2012).
- H. Zhao, G. Liu, X.-H. Li, G.S. Huang, J.D. Poplawsky, S.T. Penn, V. Dierolf, and N. Tansu, Appl. Phys. Lett. 95, 061104 (2009).
- M.-Y. Ke, C.-Y. Wang, L.-Y. Chen, H.-H. Chen, H.-L. Chiang, Y.-W. Cheng, M.-Y. Hsieh, C.-P. Chen, and J. Huang, IEEE J. Sel. Top. Quantum Electron. 15, 1242 (2009).
- Y.D. Zhuang, C.J. Lewins, S. Lis, P.A. Shields, and D.W.E. Allsopp, IEEE Photonics Technol. Lett. 25, 1047 (2013).
- C.-H. Chang, L.-Y. Chen, L.-C. Huang, Y.-T. Wang, T.-C. Lu, and J.J. Huang, IEEE J. Quantum Electron. 48, 551 (2012).
- L.-Y. Chen, C.-K. Li, J.-Y. Tan, L.-C. Huang, Y.-R. Wu, and J.J. Huang, IEEE J. Quantum Electron. 49, 224 (2013).
- V. Gottschalch, G. Wagner, J. Bauer, H. Paetzelt, and M. Shirnow, J. Cryst. Growth 310, 5123 (2008).
- F. Scholz, V. Härle, F. Steuber, H. Bolay, A. Dörnen, B. Kaufmann, V. Syganow, and A. Hangleiter, J. Cryst. Growth 170, 321 (1997).
- E. Stern, G. Cheng, E. Cimpoiasu, R. Klie, S. Guthrie, J. Klemic, I. Kretzschmar, E. Steinlauf, D. Turner-Evans, E. Broomfield, J. Hyland, R. Koudelka, T. Boone, M. Young, A. Sanders, R. Munden, T. Lee, D. Routenberg, and M.A. Reed, Nanotechnology 16, 2941 (2005).
- G. Pozina, C.W. Hsu, N. Abrikossova, and C. Hemmingsson, Crystals 13, 373 (2023).
- L.J. Lauhon, M.S. Gudiksen, and C.M. Lieber, Philos. Trans. R. Soc. London. Ser. A Math. Phys. Eng. Sci. 362, 1247 (2004).
- A.P. Vajpeyi, A.O. Ajagunna, G. Tsiakatouras, A. Adikimenakis, E. Iliopoulos, K. Tsagaraki, M. Androulidaki, and A. Georgakilas, Microelectron. Eng. 86, 812 (2009).
- H.-M. Kim, Y.-H. Cho, H. Lee, S. Il Kim, S.R. Ryu, D.Y. Kim, T.W. Kang, and K.S. Chung, Nano Lett. 4, 1059 (2004).
- A. Tanaka, R. Chen, K.L. Jungjohann, and S.A. Dayeh, Sci. Rep. 5, 17314 (2015).
- K.S. Qwah, M. Monavarian, W.Y. Ho, Y.-R. Wu, and J.S. Speck, Phys. Rev. Mater. 6, 044602 (2022).
- W. Guo, M. Zhang, A. Banerjee, and P. Bhattacharya, Nano Lett. 10, 3356 (2010).
- A. Kikuchi, M. Kawai, M. Tada, and K. Kishino, Japanese J. Appl. Physics, Part 2 Lett. 43, L1524 (2004).
- W. Guo, A. Banerjee, P. Bhattacharya, and B.S. Ooi, Appl. Phys. Lett. 98, 193102 (2011).
- R. Armitage, and K. Tsubaki, Nanotechnology 21, 195202 (2010).
- R. Gujrati, A. Srivastava, P. Vuong, V. Ottapilakkal, Y.N. Sama, T.H. Ngo, T. Moudakir, G. Patriarche, S. Gautier, P.L. Voss, S. Sundaram, J.P. Salvestrini, and A. Ougazzaden, Adv. Mater. Technol. (2023).
- H.P.T. Nguyen, K. Cui, S. Zhang, S. Fathololoumi, and Z. Mi, Nanotechnology 22, 445202 (2011).
- H. Fu and Y. Zhao, Nitride Semicond. Light. Diodes Mater. Technol. Appl. Second Ed. (Elsevier, 2018), pp. 299–325.
- J.S. Speck, and S.J. Rosner, Phys. B Condens. Matter. 273–274, 24 (1999).
- Y. Zhang, M.J. Kappers, D. Zhu, F. Oehler, F. Gao, and C.J. Humphreys, Sol. Energy Mater. Sol. Cells 117, 279 (2013).
- D. Shi, S. Feng, Y. Qiao, and P. Wen, Solid. State. Electron. 109, 25 (2015).
- J. Xie, X. Ni, Q. Fan, R. Shimada, Ü Özgür, and H. Morkoç, Appl. Phys. Lett. 93 (2008).
- X. Ni, Q. Fan, R. Shimada, Ü Özgür, and H. Morkoç, Appl. Phys. Lett. 93, 171113 (2008).
- M.-H. Kim, M.F. Schubert, Q. Dai, J.K. Kim, E.F. Schubert, J. Piprek, and Y. Park, Appl. Phys. Lett. 91, 183507 (2007).
- Y.C. Shen, G.O. Mueller, S. Watanabe, N.F. Gardner, A. Munkholm, and M.R. Krames, Appl. Phys. Lett. 91, 141101 (2007).
- J. Iveland, L. Martinelli, J. Peretti, J.S. Speck, and C. Weisbuch, Phys. Rev. Lett. 110, 177406 (2013).
- C.M. Jones, C.H. Teng, Q. Yan, P.C. Ku, and E. Kioupakis, Appl. Phys. Lett. 111 (2017).
- A. David, and M.J. Grundmann, Appl. Phys. Lett. 96, 103504 (2010).
- J. Hader, J. V. Moloney, and S.W. Koch, in Phys. Simul. Optoelectron. Devices XIV, edited by M. Osinski, F. Henneberger, and Y. Arakawa (2006), p. 61151T.
- V. Fiorentini, F. Bernardini, F. Della Sala, A. Di Carlo, and P. Lugli, Phys. Rev. B. 60, 8849 (1999).
- S.F. Chichibu, T. Azuhata, M. Sugiyama, T. Kitamura, Y. Ishida, H. Okumura, H. Nakanishi, T. Sota, and T. Mukai, J. Vac. Sci. Technol. B Microelectron. Nanom. Struct. 19, 2177 (2001).
- X. Meng, L. Wang, Z. Hao, Y. Luo, C. Sun, Y. Han, B. Xiong, J. Wang, and H. Li, Appl. Phys. Lett. 108 (2016).
- H.P.T. Nguyen, M. Djavid, K. Cui, and Z. Mi, Nanotechnology 23, 194012 (2012).
- G. Kim, J.H. Kim, E.H. Park, D. Kang, and B.-G. Park, Opt. Express 22, 1235 (2014).
- M. Zhang, P. Bhattacharya, J. Singh, and J. Hinckley, Appl. Phys. Lett. 95, 201108 (2009).
- M. Meneghini, N. Trivellin, G. Meneghesso, E. Zanoni, U. Zehnder, and B. Hahn, J. Appl. Phys. 106, 114508 (2009).
- A. Laubsch, M. Sabathil, J. Baur, M. Peter, and B. Hahn, IEEE Trans. Electron Devices 57, 79 (2010).
- K.W. Williams, N.R. Monahan, D.D. Koleske, M.H. Crawford, and X.Y. Zhu, Appl. Phys. Lett. 108 (2016).
- K.J. Vampola, N.N. Fellows, H. Masui, S.E. Brinkley, M. Furukawa, R.B. Chung, H. Sato, J. Sonoda, H. Hirasawa, M. Iza, S.P. Denbaars, and S. Nakamura, Phys. Status Solidi Appl. Mater. Sci. 206, 200 (2009).
- C.C. Pan, S. Tanaka, F. Wu, Y. Zhao, J.S. Speck, S. Nakamura, S.P. Den Baars, and D. Feezel, Appl. Phys. Express 5, 062103 (2012).
- Y. Narukawa, M. Ichikawa, D. Sanga, M. Sano, and T. Mukai, J. Phys. D. Appl. Phys. 43, 354002 (2010).
- W. Guo, M. Zhang, P. Bhattacharya, and J. Heo, Nano Lett. 11, 1434 (2011).
- E. Kioupakis, P. Rinke, K.T. Delaney, and C.G. Van de Walle, Appl. Phys. Lett. 98, 161107 (2011).
- F. Bertazzi, M. Goano, and E. Bellotti, Appl. Phys. Lett. 97, 231118 (2010).
- P.T. Barletta, E. Acar Berkman, B.F. Moody, N.A. El-Masry, A.M. Emara, M.J. Reed, and S.M. Bedair, Appl. Phys. Lett. 90, 151109 (2007).
- J.-I. Shim, H. Kim, D.-P. Han, D.-S. Shin, and K.S. Kim, in Gall. Nitride Mater. Devices IX, edited by J.-I. Chyi, Y. Nanishi, H. Morkoç, J. Piprek, E. Yoon, and H. Fujioka (2014), p. 89861S.
- Y.-W. Lin, C.-K. Wang, Y.-Z. Chiou, H.-M. Chang, and S.-J. Chang, J. Photonics Energy 5, 057612 (2015).
- J. Piprek, Phys. Status Solidi. 207, 2217 (2010).
- K.T. Delaney, P. Rinke, and C.G. Van de Walle, Appl. Phys. Lett. 94, 191109 (2009).
- P.G. Eliseev, M. Osin’ski, H. Li, and I.V. Akimova, Appl. Phys. Lett. 75, 3838 (1999).
- S.-C. Ling, T.-C. Lu, S.-P. Chang, J.-R. Chen, H.-C. Kuo, and S.-C. Wang, Appl. Phys. Lett. 96, 231101 (2010).
- E. Matioli, C. Neufeld, M. Iza, S.C. Cruz, A.A. Al-Heji, X. Chen, R.M. Farrell, S. Keller, S. DenBaars, U. Mishra, S. Nakamura, J. Speck, and C. Weisbuch, Appl. Phys. Lett. 98 (2011).
- J.H. Zhu, S.M. Zhang, H. Wang, D.G. Zhao, J.J. Zhu, Z.S. Liu, D.S. Jiang, Y.X. Qiu, and H. Yang, J. Appl. Phys. 109, 093117 (2011).
- S.-H. Han, C.-Y. Cho, S.-J. Lee, T.-Y. Park, T.-H. Kim, S.H. Park, S. Won Kang, J. Won Kim, Y.C. Kim, and S.-J. Park, Appl. Phys. Lett. 96, 051113 (2010).
- H.P.T. Nguyen, M. Djavid, S.Y. Woo, X. Liu, A.T. Connie, S. Sadaf, Q. Wang, G.A. Botton, I. Shih, and Z. Mi, Sci. Rep. 5, 7744 (2015).
- P. Chatzopoulou, I.G. Vasileiadis, P. Komninou, V. Pontikis, T. Karakostas, and G.P. Dimitrakopulos, Crystals 13, 700 (2023).
- M. Hajdel, M. Chlipała, M. Siekacz, H. Turski, P. Wolny, K. Nowakowski-Szkudlarek, A. Feduniewicz-żmuda, C. Skierbiszewski, and G. Muziol, Materials (Basel) 15, 237 (2022).
- K.J. Vampola, M. Iza, S. Keller, S.P. DenBaars, and S. Nakamura, Appl. Phys. Lett. 94, 061116 (2009).
- Ü Özgür, X. Ni, X. Li, J. Lee, S. Liu, S. Okur, V. Avrutin, A. Matulionis, and H. Morkoç, Semicond. Sci. Technol. 26, 014022 (2011).
- X. Ni, X. Li, J. Lee, S. Liu, V. Avrutin, Ü Özgür, H. Morkoç, and A. Matulionis, J. Appl. Phys. 108, 033112 (2010).
- H.P.T. Nguyen, K. Cui, S. Zhang, M. Djavid, A. Korinek, G.A. Botton, and Z. Mi, Nano Lett. 12, 1317 (2012).
- M. Djavid, H.P.T. Nguyen, S. Zhang, K. Cui, S. Fan, and Z. Mi, Semicond. Sci. Technol. 29, 085009 (2014).
- W. Shockley, and W.T. Read, Phys. Rev. 87, 835 (1952).
- P.T. Valentim, J.P. Vasco, I.J. Luxmoore, D. Szymanski, H. Vinck-Posada, A.M. Fox, D.M. Whittaker, M.S. Skolnick, and P.S.S. Guimarães, Appl. Phys. Lett. 102 (2013).
- S.A. Chevtchenko, M.A. Reshchikov, Q. Fan, X. Ni, Y.T. Moon, A.A. Baski, and H. Morkoç, J. Appl. Phys. 101, 113709 (2007).
- N. Tajik, C.M. Haapamaki, and R.R. Lapierre, Nanotechnology 23, 315703 (2012).
- M. Musolino, D. Van Treeck, A. Tahraoui, L. Scarparo, C. De Santi, M. Meneghini, E. Zanoni, L. Geelhaar, and H. Riechert, J. Appl. Phys. 119 (2016).
- H.P.T. Nguyen, S. Zhang, A.T. Connie, M.G. Kibria, Q. Wang, I. Shih, and Z. Mi, Nano Lett. 13, 5437 (2013).
- K. Ito, W. Lu, S. Katsuro, R. Okuda, N. Nakayama, N. Sone, K. Mizutani, M. Iwaya, T. Takeuchi, S. Kamiyama, and I. Akasaki, Nanoscale Adv. 4, 102 (2022).
- S. Liu, S. Han, C. Xu, H. Xu, X. Wang, D. Wang, and Y. Zhu, Opt. Mater. (Amst). 121, 111579 (2021).
- J. Slawinska, G. Muziol, M. Siekacz, H. Turski, M. Hajdel, M. Zak, A. Feduniewicz-Zmuda, G. Staszczak, and C. Skierbiszewski, Opt. Express 30, 27004 (2022).
- Y.H. Ra, R.T. Rashid, X. Liu, S.M. Sadaf, K. Mashooq, and Z. Mi, Sci. Adv. 6, 1 (2020).
- X. Liu, Y. Sun, Y. Malhotra, A. Pandey, Y. Wu, K. Sun, and Z. Mi, Appl. Phys. Lett. 119 (2021).
- Y. Wu, P. Wang, W. Lee, A. Aiello, P. Deotare, T. Norris, P. Bhattacharya, M. Kira, E. Kioupakis, and Z. Mi, Appl. Phys. Lett. 122, 160501 (2023).
- Y. Wu, X. Liu, P. Wang, D.A. Laleyan, K. Sun, Y. Sun, C. Ahn, M. Kira, E. Kioupakis, and Z. Mi, Appl. Phys. Lett. 116 (2020).
- Y. Wu, Y. Xiao, I. Navid, K. Sun, Y. Malhotra, P. Wang, D. Wang, Y. Xu, A. Pandey, M. Reddeppa, W. Shin, J. Liu, J. Min, and Z. Mi, Light Sci. Appl. 11 (2022).
- A. Aiello, Y. Wu, A. Pandey, P. Wang, W. Lee, D. Bayerl, N. Sanders, Z. Deng, J. Gim, K. Sun, R. Hovden, E. Kioupakis, Z. Mi, and P. Bhattacharya, Nano Lett. 19, 7852 (2019).
- Z. Wang, H. Sun, Q. Zhang, J. Feng, J. Zhang, Y. Li, and C.Z. Ning, Light Sci. Appl. 9, 39 (2020).
- S. Zhao, X. Liu, Y. Wu, and Z. Mi, Appl. Phys. Lett. 109 (2016).
- X. Liu, Y. Wu, Y. Malhotra, Y. Sun, and Z. Mi, Appl. Phys. Lett. 117 (2020).
- D. Bayerl, and E. Kioupakis, Appl. Phys. Lett. 115 (2019).
- A. Pandey, J. Min, M. Reddeppa, Y. Malhotra, Y. Xiao, Y. Wu, K. Sun, and Z. Mi, Nano Lett. 23, 1680 (2023).
- Y. Wu, D.A. Laleyan, Z. Deng, C. Ahn, A.F. Aiello, A. Pandey, X. Liu, P. Wang, K. Sun, E. Ahmadi, Y. Sun, M. Kira, P.K. Bhattacharya, E. Kioupakis, and Z. Mi, Adv. Electron. Mater. 6, 2000337 (2020).
- X. Liu, Y. Sun, Y. Malhotra, Y. Wu, and Z. Mi, Opt. Express 29, 32826 (2021).
- S. Rajbhandari, J.J.D. McKendry, J. Herrnsdorf, H. Chun, G. Faulkner, H. Haas, I.M. Watson, D. O’Brien, and M.D. Dawson, Semicond. Sci. Technol. 32, 023001 (2017).
- A.A. Setlur, V.R. Emil, S.H. Claire, J.-H. Her, M.S. Alok, N. Karkada, M. Satya Kishore, N. Prasanth Kumar, D. Aesram, A. Deshpande, B. Kolodin, S.G. Ljudmil, and U. Happek, Chem. Mater. 22, 4076 (2010).
- C. Sommer, P. Hartmann, P. Pachler, H. Hoschopf, and F.P. Wenzl, J. Alloys Compd. 520, 146 (2012).
- R. Haitz, and J.Y. Tsao, Phys. Status Solidi Appl. Mater. Sci. 208, 17 (2011).
- C. Kölper, M. Sabathil, M. Mandl, M. Strassburg, and B. Witzigmann, J. Light. Technol. 30, 2853 (2012).
- D. Xue, C. Ruan, Y. Zhang, H. Chen, X. Chen, C. Wu, C. Zheng, H. Chen, and W.W. Yu, Nanotechnology 29, 455708 (2018).
- H. Chun, P. Manousiadis, S. Rajbhandari, D.A. Vithanage, G. Faulkner, D. Tsonev, J.J.D. McKendry, S. Videv, E. Xie, E. Gu, M.D. Dawson, H. Haas, G.A. Turnbull, I.D.W. Samuel, and D.C. O’Brien, IEEE Photonics Technol. Lett. 26, 2035 (2014).
- N.C. George, K.A. Denault, and R. Seshadri, Annu. Rev. Mater. Res. 43, 481 (2013).
- G. Meneghesso, M. Meneghini, and E. Zanoni, J. Phys. D. Appl. Phys. 43, 354007 (2010).
- S. Mei, X. Liu, W. Zhang, R. Liu, L. Zheng, R. Guo, and P. Tian, ACS Appl. Mater. Interfaces 10, 5641 (2018).
- Z. Tian, P. Tian, X. Zhou, G. Zhou, S. Mei, W. Zhang, X. Zhang, D. Li, D. Zhou, R. Guo, S. Qu, and A.L. Rogach, Nanoscale 11, 3489 (2019).
- R. Wan, X. Gao, L. Wang, S. Zhang, X. Chen, Z. Liu, X. Yi, J. Wang, J. Li, W. Zhu, and J. Li, Photonics Res. 8, 1110 (2020).
- H. Cao, S. Lin, Z. Ma, X. Li, J. Li, and L. Zhao, IEEE Electron Device Lett. 40, 267 (2019).
- Y.J. Hong, C.-H. Lee, A. Yoon, M. Kim, H.-K. Seong, H.J. Chung, C. Sone, Y.J. Park, and G.-C. Yi, Adv. Mater. 23, 3224 (2011).
- H.W. Lin, Y.J. Lu, H.Y. Chen, H.M. Lee, and S. Gwo, Appl. Phys. Lett. 97, 073101 (2010).
- B. Damilano, N. Grandjean, C. Pernot, and J. Massies, Jpn. J. Appl. Phys. 40, L918 (2001).
- M. Yamada, Y. Narukawa, and T. Mukai, Jpn. J. Appl. Phys. 41, L246 (2002).
- S.-C. Shei, J.-K. Sheu, C.-M. Tsai, W.-C. Lai, M.-L. Lee, and C.-H. Kuo, Jpn. J. Appl. Phys. 45, 2463 (2006).
- X.H. Wang, H.Q. Jia, L.W. Guo, Z.G. Xing, Y. Wang, X.J. Pei, J.M. Zhou, and H. Chen, Appl. Phys. Lett. 91, 161912 (2007).
- H. Li, P. Li, J. Kang, Z. Li, Z. Li, J. Li, X. Yi, and G. Wang, Appl. Phys. Express 6, 102103 (2013).
- E. Jang, S. Jun, H. Jang, J. Lim, B. Kim, and Y. Kim, Adv. Mater. 22, 3076 (2010).
- J.H. Oh, S.J. Yang, and Y.R. Do, Light Sci. Appl. 3, e141 (2014).
- E.F. Schubert, and J.K. Kim, Science (80-.) 308, 1274 (2005).
- W. Bin Im, N. George, J. Kurzman, S. Brinkley, A. Mikhailovsky, J. Hu, B.F. Chmelka, S.P. Denbaars, and R. Seshadri, Adv. Mater. 23, 2300 (2011).
- T. Honda, T. Kobayashi, S. Egawa, M. Sawada, K. Sugimoto, and T. Baba, J. Cryst. Growth 298, 736 (2007).
- E.F. Schubert, Light-Emitting Diodes, Second Edition (Cambridge University Press, 2006).
- A. Yanagihara, S. Ishizawa, and K. Kishino, Appl. Phys. Express 7, 112102 (2014).
- H. Sekiguchi, K. Kishino, and A. Kikuchi, Appl. Phys. Lett. 96, 231104 (2010).
- S. Albert, A. Bengoechea-Encabo, X. Kong, M.A. Sanchez-Garcia, E. Calleja, and A. Trampert, Appl. Phys. Lett. 102, 181103 (2013).
- T. Yokota, P. Zalar, M. Kaltenbrunner, H. Jinno, N. Matsuhisa, H. Kitanosako, Y. Tachibana, W. Yukita, M. Koizumi, and T. Someya, Sci. Adv. 2 (2016).
- T. Someya, Z. Bao, and G.G. Malliaras, Nature 540, 379 (2016).
- J. Jeong, J. Jeong, Q. Wang, J. Cha, J. Cha, D.K. Jin, D.K. Jin, D.H. Shin, S. Kwon, B.K. Kang, J.H. Jang, W.S. Yang, Y.S. Choi, J. Yoo, J.K. Kim, C.H. Lee, S.W. Lee, A. Zakhidov, S. Hong, S. Hong, M.J. Kim, M.J. Kim, M.J. Kim, Y.J. Hong, and Y.J. Hong, Sci. Adv. 6 (2020).
- C.H. Yang, B.H. Chen, J.X. Zhou, Y.M. Chen, and Z.G. Suo, Adv. Mater. 28, 4480 (2016).
- J.X. Wang, C.Y. Yan, G.F. Cai, M.Q. Cui, A.L.S. Eh, and P.S. Lee, Adv. Mater. 28, 4490 (2016).
- F.M. Kochetkov, V. Neplokh, V.A. Mastalieva, S. Mukhangali, A.A. Vorob’ev, A.V. Uvarov, F.E. Komissarenko, D.M. Mitin, A. Kapoor, J. Eymery, N. Amador-Mendez, C. Durand, D. Krasnikov, A.G. Nasibulin, M. Tchernycheva, and I.S. Mukhin, Nanomaterials 11, 1503 (2021).
- A. Chortos, J. Liu, and Z. Bao, Nat. Mater. 15, 937 (2016).
- C.M. Zgierski-Johnston, S. Ayub, M.C. Fernández, E.A. Rog-Zielinska, F. Barz, O. Paul, P. Kohl, and P. Ruther, Prog. Biophys. Mol. Biol. 154, 51 (2020).
- Y. Huang, E.L. Hsiang, M.Y. Deng, and S.T. Wu, Light Sci. Appl. 9, 105 (2020).
- M. Alonso-Orts, R. Hötzel, T. Grieb, M. Auf der Maur, M. Ries, F. Nippert, B. März, K. Müller-Caspary, M.R. Wagner, A. Rosenauer, and M. Eickhoff, Discov. Nano. 18, 27 (2023).
- L. Dvoretckaia, V. Gridchin, A. Mozharov, A. Maksimova, A. Dragunova, I. Melnichenko, D. Mitin, A. Vinogradov, I. Mukhin, and G. Cirlin, Nanomaterials 12, 1993 (2022).
- C. Zhao, T.K. Ng, R.T. Elafandy, A. Prabaswara, G.B. Consiglio, I.A. Ajia, I.S. Roqan, B. Janjua, C. Shen, J. Eid, A.Y. Alyamani, M.M. El-Desouki, and B.S. Ooi, Nano Lett. 16, 4616 (2016).
- S. Zhang, A.T. Connie, D.A. Laleyan, H.P.T. Nguyen, Q. Wang, J. Song, I. Shih, and Z. Mi, IEEE J. Quantum Electron. 50, 483 (2014).
- S.M. Sadaf, Y.H. Ra, H.P.T. Nguyen, M. Djavid, and Z. Mi, Nano Lett. 15, 6696 (2015).
- M. Tchernycheva, A. Messanvi, A. De Luna Bugallo, G. Jacopin, P. Lavenus, L. Rigutti, H. Zhang, Y. Halioua, F.H. Julien, J. Eymery, and C. Durand, Nano Lett. 14, 3515 (2014).
- H.P.T. Nguyen, Q. Wang, and Z. Mi, J. Electron. Mater. 43, 868 (2014).
- Y. Park, S. Jahangir, Y. Park, P. Bhattacharya, and J. Heo, Opt. Express 23, A650 (2015).
- X. Luo, W. Song, F. Gao, J. Shi, C. Cheng, J. Guo, L. He, Q. Liu, Y. Yang, S. Li, and Q. Wu, Adv. Eng. Mater. 23, 2001430 (2021).
- K.H. Li, W.Y. Fu, and H.W. Choi, Prog. Quantum Electron. 70, 100247 (2020).