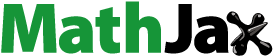
ABSTRACT
High-concentration protein formulation is of paramount importance in patient-centric drug product development, but it also presents challenges due to the potential for enhanced aggregation and increased viscosity. The analysis of critical quality attributes often necessitates the transfer of samples from their primary containers together with sample dilution. Therefore, there is a demand for noninvasive, in situ biophysical methods to assess protein drug products directly in primary sterile containers, such as prefilled syringes, without dilution. In this study, we introduce a novel application of water proton nuclear magnetic resonance (wNMR) to evaluate the aggregation propensity of a high-concentration drug product, Dupixent® (dupilumab), under stress conditions. wNMR results demonstrate a concentration-dependent, reversible association of dupilumab in the commercial formulation, as well as irreversible aggregation when exposed to accelerated thermal stress, but gradually reversible aggregation when exposed to freeze and thaw cycles. Importantly, these results show a strong correlation with data obtained from established biophysical analytical tools widely used in the pharmaceutical industry. The application of wNMR represents a promising approach for in situ noninvasive analysis of high-concentration protein formulations directly in their primary containers, providing valuable insights for drug development and quality assessment.
Introduction
Therapeutic proteins, especially monoclonal antibodies (mAbs), are increasingly being formulated at concentrations exceeding 100 mg/mL to enhance convenience for patients.Citation1 However, such high concentrations pose substantial challenges to product performance and stability, including high viscosity, protein self-association, and aggregation.Citation2,Citation3 One primary concern is the increased viscosity, often attributed to concentration-dependent protein–protein interactions, leading to protein self-association.Citation2,Citation4–9 This heightened viscosity can introduce complications in formulation, manufacturing, device development, and drug administration. Protein self-association, often triggered by unfavorable molecular attributes or solution properties, can result in gelation or phase separation.Citation4,Citation6,Citation10–12 Furthermore, protein aggregation, which can be influenced by factors like temperature, agitation, light exposure, and high concentration, is typically viewed as a critical quality attribute (CQA) when evaluating protein product quality. Such aggregation could pose potential risks to patient safety, decrease purification yields, reduce product shelf-life, and present manufacturing challenges.Citation5,Citation13–16 Therefore, detecting protein aggregation in high-concentration formulations is essential for the successful development and manufacturing of stable therapeutic proteins and mAbs.
High-concentration protein formulation, however, introduces a myriad of analytical challenges. For example, samples are removed from their primary containers or devices for many laboratory analyses. In addition, due to the inherent limitations and specific requirements of certain analytical techniques, proteins are frequently diluted to lower concentrations. For instance, routine laboratory applications such as dynamic light scattering (DLS),Citation17,Citation18 infrared (IR) spectroscopy (utilizing microfluidic modulation spectroscopy (MMS),Citation19,Citation20 circular dichroism (CD),Citation21,Citation22 and differential scanning calorimetry (DSC)Citation23,Citation24 typically handle protein concentrations ranging between 1 and 10 mg/mL. Principally, and in specific setups, techniques like MMS and DLS are capable of accommodating protein samples up to 200 mg/mL for analysis. In many cases, dilutions risk obfuscating concentration-dependent attributes like reversible protein aggregation. Moreover, chromatographic and electrophoretic assays might not faithfully represent non-covalent higher molecular weight forms. This discrepancy arises from factors intrinsic to these methods, such as column filters, requisite sample dilutions, and the use of mobile phases.Citation16 DLS, on the other hand, has inherent aggregate size limitations due to its reliance on Brownian motion where the intensity of the scattered light is proportional to the sixth power of the particle diameter as well as the ratio between the particle size and the wavelength of the incident light, making this technique very sensitive to the presence of large particles.Citation17,Citation18 Similarly, small-angle X-ray scattering measurements, dictated by instrument and detector design, are generally confined to sizes less than 1–2 µm.Citation25 Light-based particle characterization methodologies, including light obscuration methods (e.g., high accuracy (HIAC) liquid particle counter), microflow imaging (MFI), and various optical spectroscopic techniques, are not exempt from these challenges. They often necessitate sample perturbation and dilution to circumvent issues like a decrease in detector sensitivity from high refractive indices and potential detector overloading.Citation26–28
Water proton nuclear magnetic resonance (wNMR) has proven to be an in-situ and noninvasive analytical technique capable of reliably detecting protein aggregation in aqueous solutions.Citation29–31 It uses benchtop time-domain NMR instruments to monitor the dynamics of the water proton (Citation1H2O) NMR signal, which probe solutes in aqueous solutions through the water proton transverse relaxation rate R2(Citation1H2O) rather than measuring the solute (e.g., protein) NMR signal directly.Citation32 Data collection is performed on sealed and labeled vials without any requirement for optical transparency. There is no sample preparation of any kind during measurement, which makes it feasible to conduct a noninvasive quantitative inspection of complex drug products. Previous research showed that wNMR can detect protein aggregation over a wide concentration and particle size range, such as human insulin (0.4 mg/mL),Citation33 bovine serum albumin and human γ-globulin (15 mg/mL),Citation34 and therapeutic mAbs (55 mg/mL).Citation29,Citation32 Further, wNMR can detect protein aggregation under flow conditions.Citation35 More recently, it was shown that wNMR can detect mAb oxidative degradation and excellent agreement was shown between wNMR data and principal component analysis (PCA) of high-field NMR data with a linear correlation coefficient of 0.950.Citation36 Moreover, proton exchange between water molecules and the exchangeable protons of protein and protein aggregates or assemblies makes wNMR a sensitive tool for probing the formation of aggregates. Our previous studies have demonstrated the effects of protein concentration and protein aggregation on wNMR observations for insulin, model systems (such as bovine serum albumin and human IgGs) and therapeutic proteins (mAbs). In these reports, we thoroughly explored the causes of R2(Citation1H2O) changes influenced by the aforementioned factors and provided the fundamental basics of the water sensitivity to the solute dynamics.Citation29,Citation30,Citation32–34,Citation37 The lower detection limit of aggregates will likely depend on factors such as protein type, protein concentration, formulation matrix, stress types, and duration. In a previous study using a mAb at 55 mg/mL formulated using 20 mM histidine at pH 6, we observed that a change of the mAb aggregation level by ~0.5% could be detected by R2(Citation1H2O).Citation29 In addition, high-field NMR was used to monitor the higher-order-structure (HOS) of mAbs in solution and to map mAb structure by two-dimensional (2D) NMR at natural abundance.Citation38,Citation39
In this study, we evaluated wNMR as a biophysical tool to probe protein aggregation in a prefilled syringe (PFS) and compared wNMR with orthogonal biophysical analytical techniques commonly used by the pharmaceutical industry. As a test agent, we studied the commercially available high-concentration mAb product, Dupixent® (dupilumab). Intact Dupixent® PFSs were subjected to heating and freeze–thaw (F/T) stresses in situ. These stress conditions can often induce various levels and diverse types of aggregates of a mAb. The water proton transverse relaxation rate R2(Citation1H2O) in the stressed syringes was directly and noninvasively measured using wNMR without aliquoting from PFSs (in situ detection). We then analyzed the samples invasively using a set of orthogonal techniques to assess protein aggregation, and to obtain structural and subvisible particle information using high-performance size-exclusion chromatography (HPSEC), DLS, CD, IR, DSC, protein stability analysis (UNCLE), and Aura (for subvisible particle analysis). By comparing results from different analytical techniques, we evaluate the capabilities of wNMR to detect protein aggregation in high-concentration solution directly, and as a noninvasive in situ tool for protein product quality assessment.
Materials and methods
Materials
Milli-Q water was obtained from Millipore Milli-Q® Integral 5 Water Purification System (Darmstadt, Germany) and the dupilumab formulation buffer was made in-house based on the formulation components from the product description (25 mM arginine, 20 mM histidine, 12 mM acetate, 0.2% polysorbate 80, 5% sucrose, and pH 5.9). Six syringes of Dupixent® were purchased through Myonex, LLC (Norristown, PA), with each syringe containing 2 mL of dupilumab of 150 mg/mL. These PFSs were directly subjected to prolonged heating stresses at 50°C and multiple F/T cycles of −80°C/5°C, as described below. Moreover, one PFS from the product packages was emptied and filled with the formulation buffer as the control sample. Eppendorf tubes and containers, high-performance liquid chromatography (HPLC) vials, and other general lab supplies were purchased from Thermo Fisher Scientific Inc. (Waltham, MA).
Samples to evaluate protein stability upon F/T cycles and thermal stress
Samples for F/T stress were held at −80°C for over 6 h and placed at 5°C to thaw overnight. After four F/T cycles, all samples were tested immediately by high-performance size-exclusion chromatography (HPSEC) or stored at 5°C for extended periods of time (e.g., 1 d 5 d, 9 d, 19d) for sequential analyses. Samples for thermal stress were placed at 50°C and pulled at various time points (0 h, 3 h, 6 h, 9 h, 12 h, 18 h, 24 h, and 48 h) for HPSEC assay, except for the direct incubation of samples at 50°C for DLS and CD measurements. The 48-h 50°C samples were also stored at 5°C for an extended time (1d and 5d) and subjected to HPSEC measurement.
wNMR
For all wNMR measurements, a variable temperature benchtop NMR (VT-NMR) instrument custom-made by Resonance Systems GmbH (Kirchheim/Teck, Germany), with a 1H resonance frequency of 22.4 MHz (0.52 T) and a permanent magnet temperature of 22°C, was used. The bore size of the instrument is 32 mm, which can accommodate drug products in their original containers (e.g., sealed vials, PFS, injection pens). For the stability of the permanent magnet of VT-NMR, the room temperature was kept at ~19°C. Temperature control of a sample inside the VT-NMR probe cavity is achieved by means of cooled airflow supplied by an AirJet XR902 cooler made by SP Scientific (Stone Ridge, NY), and regulated by the automated temperature controller of the instrument. Detailed experimental procedures and parameters are included in the supporting information (SI).
Alt text. An image displaying the Dupixent® syringe placed within a 26 mm glass NMR tube, prior to its insertion into a wide-bore, temperature-controlled benchtop NMR instrument. The figure emphasizes the advantage of water proton NMR, illustrating its capacity to gather data from drug products in their primary containers without necessitating any sample modifications.
Since the purchased syringes of Dupixent® were provided with a safety device body spanning larger than the bore size of the VT-NMR probe (ca. 45 mm vs. 32 mm), for wNMR measurements, the syringe was taken out of the shield after careful cutting of the shield clips holding the syringe inside the needle shield (Figure S1); the needle cap and the staked-in needle remained intact. To keep the retrieved syringe without the needle guard in the vertical position and in the center of the VT-NMR probe, the syringe was placed inside a round bottom borosilicate glass tube (20 mm OD), which, in turn, was inserted in a standard 26-mm NMR glass tube. shows the Dupixent® syringe about to be lowered into the VT-NMR probe bore for measurements.
Figure 1. Illustration of a Dupixent® syringe before being inserted into the VT-NMR probe for analysis at 5°C. wNMR can collect data on sealed intact products (in situ) without any sample preparation procedures eliminating all errors associated with sample preparation.

The water proton transverse relaxation rate R2(Citation1H2O) was measured using the standard Carr-Purcell-Meiboom-Gill (CPMG) pulse sequence,Citation40 90°x–(τ–180°y–τ)n, where τ is an interpulse delay in ms, the 90°- and 180°-pulses, 18 μs and 40 μs, respectively, were calibrated using a mineral oil standard (cat. No. M8410-100 ML, Sigma Aldrich, St. Louis, MO), and n is the number of spin-echoes varied from 1 to t/2τ, where t is the total time of the collection of spin-echo intensity decay. Other CPMG parameters used for the analysis of unstressed and stressed Dupixent® syringes as well as the formulation buffer are provided in the SI.
The values of R2(Citation1H2O) were extracted from the single-exponential fit of the experimental spin-echo intensity decay over time I(t) using EquationEquation (1)(1)
(1)
where I(t) is the spin-echo signal intensity at time t, I(0) is the spin-echo signal intensity extrapolated to t = 0, and t varies from 2τ to 2τn, as follows from the above definition of the number of collected spin-echoes, n. The typical t range in this work is from 3 s at 5°C to 18 s at 50°C. More details of wNMR measurement procedures and parameters are provided in the SI.
wNMR measurements were performed on unstressed Syringes 1–3 at 5°C. Afterwards, the content of Syringe 1 was transferred to glass vials for dilution studies. Syringe 1 was then filled with the formulation buffer to serve as a control sample in accelerated stress studies. Syringe 2 was subjected to repeated F/T cycles of (−25°C, 6 h)/(5°C, 18 h), while Syringe 3 was subjected to prolonged heating stress at 50°C for 48 h. Syringe 1, the control, was subjected first to the same repeated F/T cycles as Syringe 2, and then to the same heating stress as Syringe 3. Each wNMR measurement takes about 2.5 min. wNMR measurements were performed on Syringes 1–3 during the stress studies. Details on dilution and stress studies are presented in the SI.
Differential scanning calorimetry
The thermal unfolding profile of protein samples was determined in duplicated measurement by VP-DSC (Valerian Plotnikov differential scanning calorimetry, Malvern, United Kingdom). A temperature ramp was applied from 5°C to 120°C with a scan rate of 90°C/h. Samples were diluted to ~1 mg/mL with the formulation buffer (25 mM arginine, 20 mM histidine, 12 mM acetate, 0.2% polysorbate 80, 5% sucrose, and pH 5.9) prior to measurement, and the same formulation buffer was used as the reference solution, with 1 mL loading volume for both. The sample thermograms are corrected using those obtained from background buffer runs. Both cells of the DSC instrument were filled with the formulation buffer. Protein unfolding temperatures (e.g., Tonset, Tm1, Tm2) were determined using Origin (version 7, Origin Lab, Massachusetts, USA). In addition, the heat capacity values were normalized by sample exact concentration (1.062 mg/mL), molecular weight (147 kDa), and cell volume (0.142 mL) to report as CP (isobaric heat capacity in kcal/mol⋅°C) for generating thermal unfolding profiles.
Dynamic light scattering
Size distribution and melting (thermal profiles) of protein samples were measured using a DynaPro II plate reader (Wyatt Technology, Santa Barbara, USA). The measurements were performed directly in 96-well and 384-well glass-bottom microplates (Corning, Corning, NY) using either 80 uL or 40 uL sample volume, respectively, topped with 10–20 uL silicone oil (Alfa Aesar, Ward Hill, MA) to prevent evaporation. The plate was centrifuged for 1 min at 4000 rpm prior to measurement. Data were collected and processed with the DYNAMICS software version 7.8 (Wyatt Technology, Santa Barbara, USA). For 50°C time course measurement, a measurement interval of 120 min was applied for up to 48 h (about 2 d) in total. For the melting experiment, a temperature ramp from 20°C to 90°C was applied at an interval of 5°C. Each measurement was performed with 10 acquisitions of 5 s per acquisition as DLS settings. All protein samples were analyzed neat at a concentration of ~150 mg/mL in the formulation buffer (no dilution applied). Peak hydrodynamic diameters of the monomer were obtained directly from the reports in instrument software DYNAMICS (version 7.8) for reporting and then corrected with sample viscosity and plotted over time course or temperature ramp separately.
High-performance size-exclusion chromatography
HPSEC separation of protein samples was performed using a YMC (Devens, MA) Pack Diol-200 column (300 × 8.0 mm, 5 um) on Waters Alliance HPLC system (Waters Corp., Milford, MA). Samples were injected neat at ~150 mg/mL with 2 uL injection volume at various time and temperature points on the column for HPSEC analyses with a mobile phase of 50 mM phosphate, 450 mM arginine monohydrochloride pH 7.0 with an isocratic setting at a flow rate of 0.5 mL/min and with UV (Ultraviolet) detection at 280 nm for protein detection. Column and sample temperatures were set at 25°C and 5°C, respectively. For each test sample, the area percent for the monomer, total high molecular weight (HMW) species, and total low molecular weight (LMW) species were calculated.
Tagg and Tm measurement using UNCLE
Native static light scattering (SLS) and fluorescence to monitor protein aggregation temperature (Tagg, the temperature at which proteins show a tendency to oligomerize and aggregate) and determination of the unfolding temperatures (Tm) were performed on protein samples using an UNCLE instrument (Unchained Labs, Boston, MA). Filters UV (266 nm) (for small aggregates) and Blue (473 nm) (for large protein aggregates) were used with 10 s exposure time per measurement. Each measurement was performed with four acquisitions of 5 s per acquisition as SLS settings. All samples were analyzed at their original concentration (~150 mg/mL) with a 9 uL injection volume. A temperature ramp from 20°C to 90°C was applied with a 1.0°C/min increment. All data were analyzed directly with instrument software Uncle Analysis (version 5.03) and Tm and Tagg were determined using internal software algorithm on BCM (Barycentric Mean peak intensity wavelength, nm) or SLS473 (counts.nm) vs. Temperature (ºC) plots, respectively.
Near-UV circular dichroism
CD analysis was performed using a Jasco J-1500 spectropolarimeter (Jasco, Easton, MD). The instrument detector chamber was purged with N2 for at least 5 min before sample measurement. Near-UV CD spectra were acquired at 50°C with a time course of up to 48 h with 120 min increments. Near-UV CD measurement was performed using a 0.01 mm pathlength cuvette (Hellma Analytics, Plainview, NY) with 1 nm bandwidth and scanning range at 240–350 nm. The protein samples were analyzed with neat concentration at ~150 mg/mL and 40 uL loading volume. Spectra of each sample were acquired, and the baseline was corrected by subtracting the spectral attributes of corresponding diluents, and formulation buffer for Near-UV CD.
Fourier-transform infrared spectroscopy
Fourier-transform IR spectroscopy analysis was performed using a RedShiftBio AQS3 Pro IR instrument (RedShift BioAnalytics, Inc., Burlington, MA). IR spectra of stressed and control samples (diluted with a formulation buffer to 2 mg/mL as working concentration, with 1.5 mL loading volume per well) were acquired at room temperature in the wavenumber range of 1600–1700 cm−1 with 4 cm−1 resolution and five acquisitions per measurement. Spectra from replicas of the same sample are averaged out with a Savitzky-Golay smoothing value of 19 and plotted. The content of total α-helix % and total β-sheet % from each sample is calculated using AQS3 delta software via the built-in deconvolution function and reported to one decimal place.
Subvisible particle counts using Aura
Subvisible particulate characterization on protein samples (neat concentration at ~150 mg/mL) was performed using the subvisible particle instrument Aura (Halo Labs, Burlingame, CA). Each sample was mixed well with gentle pipetting and loaded onto the Aura black membrane 96-well plate (Halo Labs, Burlingame, CA) with a 30 uL loading volume for measurement. Sub-visible particulates in the range of ≥2 μm, ≥5 μm, ≥10 μm, and ≥25 μm were analyzed by applying a standard filter in the instrument software Particle Vue (version 3.1.0.29) and reporting the corrected data as particles/mL to zero decimal point.
Results
We conducted heating and F/T stress studies and used wNMR to assess the biophysical stability of dupilumab, the active agent in Dupixent®, against stresses. As SEC and DLS can qualitatively evaluate protein aggregates, and CD and IR can evaluate the potential structural changes in these stressed samples,Citation41–43 we used these techniques as orthogonal analytical methods to compare with wNMR findings.
Assessing product content uniformity using in situ wNMR
Content uniformity is a CQA of drug products.Citation44 The NMR transverse relaxation rate of water, R2(Citation1H2O), is sensitive to protein concentrationCitation45 and, therefore, can be used for content uniformity assessment. We previously demonstrated that in the absence of protein aggregation, R2(Citation1H2O) increases linearly with protein concentration while in the presence of protein aggregation, R2(Citation1H2O) increases nonlinearly with protein concentration.Citation37 Using a marketed insulin product, FlexPen®, we demonstrated that R2(Citation1H2O) decreases linearly with the insulin concentration when the original insulin content in the product was diluted by the formulation buffer.Citation46 These studies form the basis of using R2(Citation1H2O) to assess content uniformity. The average coefficient of variance (CV) value in our experiments was 0.29%. In this study, we measured three PFSs of Dupixent® using wNMR. Each PFS of Dupixent® was measured in triplicates at 5°C to obtain R2(Citation1H2O) signal. shows the R2(Citation1H2O) values detected in Syringes 1–3. The average R2(Citation1H2O) of the three syringes is 6.820 ± 0.046 s−1 with a CV of 0.007. The small variation of the R2(Citation1H2O) value of the three PFSs indicates that they have good content uniformity. When the drug solution in Syringe 1 is replaced by the formulation buffer, its R2(Citation1H2O) is 0.694 ± 0.001 s−1. The much higher R2(Citation1H2O) value of the drug solution than the formulation buffer is consistent with the high mAb concentration in the drug solution.
Figure 2. R2(Citation1H2O) of three PFSs of Dupixent® (data taken at 5°C, 0.52 T, 22.4 MHz for1H) from wNMR. The R2(Citation1H2O) value and error bar (standard deviation, STD) for each syringe was the arithmetic average from triplicate measurements.

Alt text. This figure demonstrates the consistency of the NMR transverse relaxation rate of water, measured using a benchtop NMR instrument. Displayed as a bar graph, the average value of water relaxation for each of the three tested syringes, each measured in triplicate, reveals a significant reproducibility in the readings.
Detecting reversible mAb aggregation using ex situ wNMR
Many factors can affect protein stability in formulation, manufacturing, shipping, and storage. In this section, we used wNMR to evaluate the impact of dilution on Dupixent®. Dilution of the drug solution with the formulation buffer was performed ex situ in Nalgene® cryo-vials, and wNMR measurements were also done on these vials (ex situ of the original syringe), each filled with 1 mL drug solution of differing mAb concentrations, which were achieved through a serial 1:1 v:v dilution of the original drug solution of 150 mg/mL of Dupixent®. shows R2(Citation1H2O) of the concentration series at 150, 75, 37.5, 18.8, 9.4, and 0 mg/mL. R2(Citation1H2O) displays a clear nonlinear dependence on the mAb concentration, C(mAb), with an upward swing at high concentrations. This type of nonlinear dependence of R2(Citation1H2O) is indicative of mAb aggregation at higher concentrations, and such an aggregation is reversed by dilution.Citation37 However, as the mAb concentration increases, the viscosity of the solution is also likely to increase, which may affect R2(Citation1H2O).
Figure 3. (a) R2(Citation1H2O) vs. dupilumab concentration at 25°C. The highest dupilumab concentration is 150 mg/mL, which is the undiluted drug solution in Dupixent®, which has a viscosity of 5.82 cP at 25°C. (b) R2(Citation1H2O) vs. viscosity for glycerol-water mixtures at 25°C. The highest glycerol concentration is 80% w/w, with a viscosity of 47.0 cP at 25°C.

Alt text. This figure presents a relationship between the NMR transverse relaxation rate of water and the Dupixent® concentration and viscosity at 25°C, this graph signifies that an increase in mAb viscosity is not the sole contributor to elevated water relaxation time as mAb concentration rises.
To assess the impact of viscosity on R2(Citation1H2O), we prepared a series of glycerol-water mixtures, with the glycerol concentration ranging from 0% to 80% w/w (0 to 963 mg/mL) and measured their R2(Citation1H2O) values. Glycerol is often used as a surrogate in viscosity studies.Citation47,Citation48 The R2(Citation1H2O) vs. glycerol concentration plot is shown in Figure S2. The viscosity of the glycerol-water mixtures is reported in the literatureCitation49 and is listed in Table S2 along with R2(Citation1H2O) values. plots R2(Citation1H2O) vs. viscosity for the glycerol-water mixtures. R2(Citation1H2O) grows nonlinearly with viscosity. However, while the growth of R2(Citation1H2O) accelerates at high mAb concentration, the growth of R2(Citation1H2O) decelerates at high viscosity. This contrast suggests that, although viscosity elevates R2(Citation1H2O) as the mAb concentration increases, it is not the sole factor. Indeed, at 25°C, as the glycerol concentration increases from 0% to 50% w/w (560 mg/mL), which has a viscosity of 5.10 centipoise (cP), the magnitude of R2(Citation1H2O) increase is 0.965 s−1 (from 0.407 to 1.372 s−1). In comparison, as the dupilumab concentration increases from 0 to 150 mg/mL, which has a viscosity of 5.89 cP (comparable to 50% w/w glycerol-water), the magnitude of R2(Citation1H2O) increase is 6.87 s−1 (from 0.808 to 7.68 s−1). Dupilumab induces a much larger increase in R2(Citation1H2O) than would be expected from viscosity alone. Hence, the accelerated rise of R2(Citation1H2O) at high dupilumab concentration likely reflects reversible mAb association, as reported previously.Citation37
Profile of thermal stability
To further evaluate the effects thermal stress and dilution have on protein stability, we obtained the thermal profile of Dupixent® by using DSC. As shown in , DSC data identify three unfolding transition temperatures (Tm1, Tm2, Tm3) during the thermal denaturation process at ~66°C, ~72°C, and ~81°C, respectively, and the protein unfolding onset temperature, Tonset, at ~59°C. As such, the thermal stress at ~50°C in a short time scale (less than 50 h) will likely not result in a significant unfolding of dupilumab because the Tonset is close to 60°C. Based on these data, we planned to have the sample in the syringe or vial stressed at 50°C as an accelerated thermal stress condition in this study to determine its impact on R2(Citation1H2O), protein aggregation, and other attributes of Dupixent®.
Figure 4. Thermal profile of Dupixent® from DSC measurements. The same sample (dupilumab) was measured in duplicates in DSC measurement, and the profiles were overlayed. Thermal transition temperatures were included in a table embedded in the figure. Two replicated profiles were presented using solid blue and red, respectively.

Alt text. A thermal profile of Dupixent® is depicted, showcasing data related to protein unfolding temperatures. This graph substantiates the rationale behind the chosen temperature for applying thermal stress to Dupixent®, ensuring no protein unfolding during the incubation phase.
The impact of prolonged heating stress on the drug product was assessed in real-time using wNMR. Syringe 3 was put in the VT-NMR magnet bore at 50°C for 48 h. R2(Citation1H2O) was measured once every 30 min. Syringe 1, filled with the formulation buffer, served as the control, and went through the same heating stress and data collection procedure (50°C for 48 h, R2(Citation1H2O) measured once every 30 min). The R2(Citation1H2O, t) dependence obtained for Syringe 3 (0 ≤ t ≤ 48 h) is used to evaluate the impact of heating stress on the drug product. To our knowledge, these are the first results reported for a drug product presented in a PFS that was monitored directly while under heating stress.
Alt text. This figure delineates the repercussions of thermal stress on Dupixent® by comparing the NMR transverse relaxation rate of water and the average protein hydrodynamic particle size in solution. The data, derived from wNMR and DLS, respectively, indicate consistent trends in responses upon 50°C thermal stress over a 48-h period.
A gradual increase (from 2.351 s−1 to 2.433 s−1) of R2(Citation1H2O) was observed during the 48-h heating period (), indicating that the protein (dupilumab) is slowly aggregating at 50°C, even though 50°C is below the onset of thermal unfolding. The magnitude of R2(Citation1H2O) increase at 50°C is ~0.08 s−1. After this heating period, R2(Citation1H2O, mAb) was measured again at 5°C and compared with R2(Citation1H2O, mAb) measured at 5°C before heating, R2(Citation1H2O, mAb) increased by ~0.07 s−1 (Figure S3). This suggests that heat-induced dupilumab aggregation is mostly irreversible, i.e., most mAb aggregates persist after the sample temperature reverts to 5°C.
Figure 5. Impact of heating stress (50°C) on Dupixent® monitored in situ by wNMR (a) and ex situ by DLS (b). The slope and intercept of the linear fitting of the raw data are shown in the plots.

A similar upward trend was detected in the average hydrodynamic size (in diameter, nm) that was determined via DLS of the molecule (dupilumab) in its formulation buffer. Heating stress at 50°C caused the sample to gradually increase the average hydrodynamic size in solution over the 48-h incubation period (), demonstrating that dupilumab underwent aggregation during heating stress.
Alt text. This figure shows the impact of aggregation rate change of protein molecule upon thermal stress (50°C) and the trending of changes for both % HMW and %Monomer is with multiple slopes during the 48-hr period.
In addition, protein aggregation was quantitatively evaluated using HPSEC. Accelerated thermal stress (50°C) caused the sample to gradually form HMW aggregates in solution, with periodical increasing from ~1% at the initial time point to ~7% at the 48-h time point. The increase rate also slows down over time (slope in various regions on the plot) during this period (). HMW percentage is composed of the total percentage of HMW1 and HMW2, which are the two distinct HMW species eluted earlier than the monomer peak and are distinguished by their molecular weight (Figure S4). Accordingly, the monomer peak percentage gradually decreased over the 48-h time course (). Compared to the control sample, there is an increase in the HMW percentage once the samples are stressed at 50°C for 48 h followed by an incubation of the samples at 5°C for 1 d or 5 d (Figure S5). Notably, all these samples have comparable levels of protein aggregation to that of thermally stressed samples. This result indicates that heat-induced dupilumab aggregation is irreversible, consistent with the wNMR result. Real-time structural monitoring by near-UV CD shows comparable spectral every 2 h up to 48 h at 50°C ( and Figure S6), which indicates the comparable tertiary structure of the protein during accelerated thermal stress at 50°C for 48 h regardless of the increase in protein aggregation as a short time scale response. Unlike other sensitive techniques, near-UV CD has limited ability to show differences in the overall tertiary structure of the protein, with only a relatively small percentage (~7%) of the aggregates detected via SEC, whereas the majority of the protein stays as monomer (~93%) in solution in this study.
Figure 6. Protein aggregation upon thermal stressing quantified by HPSEC. The impact of thermal stress up to 48 h at 50°C reflected by protein aggregates that were presented in high molecular weight species (HMW, dark blue). The monomer peak percentage was presented in red. Error bars represent the average assay variation for each data point. HPSEC runs were conducted at 25°C.

Figure 7. Accelerated thermal stress impact of tertiary structure on Dupixent® by near-UV CD. Near-UV CD spectra overlay (CD signal, mdeg vs. Wavelength nm) of the sample that was incubated at 50°C) for up to 48 h (time, min). Near-UV CD detected no tertiary structural conformational change for the sample upon thermal stress (50°C) during the monitored period (48 h).

Alt text. A figure exhibiting near-UV CD spectra of Dupixent® under 50°C thermal stress across 48 h, the graph communicates that the protein’s tertiary structure remains intact despite evident protein aggregation, corroborating observations from prior studies.
Samples subjected to accelerated thermal stress at 50°C for 48 h were further diluted to low concentration (1 mg/mL), and undiluted samples were injected neat (at ~150 mg/mL) prior to HPSEC analysis. Monomer percentage decrease in stressed condition vs. control sample and comparable monomer percentages in HPSEC dilution impact analyses () suggest the sample dilution before injecting for chromatography measurement may not affect the protein aggregation. It is worth noting that protein samples are mixed with elution phases in the column and therefore will be diluted. Nevertheless, the HPSEC data confirm the conclusion drawn from R2(Citation1H2O) data that after 48 h heating at 50°C, dupilumab form aggregates, which persist after the sample has been brought back to 5° or 25°C, suggesting irreversible aggregation.
Figure 8. Impact of dilution on protein aggregation detected by HPSEC. Monomer and HMW percentage plot for unstressed control sample, 50°C 48 h sample without dilution, and 50°C 48 h diluted sample. HPSEC runs were conducted at 25°C.

Alt text. A comparative graph presents percentages of protein HMW and monomer species in solution after exposure to control conditions, 50°C stress, and 50°C stress followed by dilution. The findings indicate that dilution does not affect protein aggregation beyond what thermal stress alone incurs.
Impact of F/T stress on protein stability
To evaluate the impact of F/T stress on intact Dupixent® product, one product syringe (Syringe 2) and one control syringe (emptied Syringe 1 filled with the formulation buffer) were subjected in parallel to 11 cycles of F/T stress with each cycle containing a freezing process at −25°C for 6 h and a thawing process at 5°C for 18 h. In total, there were 17 R2(Citation1H2O) measurements by wNMR, including one on unstressed sample, 11 immediately after each F/T cycle, and five on various days of storage at 5°C (3 d, 5 d, 10 d, and 17 d). The 17 R2(Citation1H2O) values are summarized in Table S1 and plotted in . The control, Syringe 1 filled with buffer, displayed slight variation of R2(Citation1H2O) throughout the 11 F/T cycles (for data, see Table S1).
Figure 9. Impact of repeated in situ F/T stress on Dupixent®. Measurements were made on syringe 2 at 5°C after the frozen syringe has thawed completely after each F/T cycle of (−25°C, 6h)/(5°C, 18 h).



Alt text. This image charts the reactions of the NMR transverse relaxation rate of water to freeze and thaw stress directly applied to syringes. The data implies the formation of reversible aggregates throughout the process.
There are two notable features of the results. First, F/T stress on the intact product caused R2(Citation1H2O) to increase, which is likely due to mAb aggregation. Repeated F/T stresses caused no or a very small additional increase in R2(Citation1H2O), suggesting that existing aggregates did not serve as nuclei to induce significant further aggregation, even when stressed again. Second, after each period of incubation for a selected number (3, 5, 10, 17) of days at 5°C, R2(Citation1H2O) decreases. After the 11th F/T cycle, R2(Citation1H2O) decreased steadily during incubation at 5°C, and after 17 d, R2(Citation1H2O) fully returned to its pre-stress level. This result suggests that during incubation at 5°C, mAb aggregates caused by F/T stresses gradually dissociate. To summarize, wNMR data suggest that F/T stress on the intact drug product causes reversible mAb aggregation and the aggregates gradually fully dissociate after storage at 5°C.
Similarly, we evaluated the protein aggregation change in F/T-stressed samples using HPSEC. Protein aggregation induced by F/T stress (4 F/T (−80°C to 5°C) cycles) compared to the control samples in the syringe increased from ~1% to 1.5% in HMW species (in blue) and accordingly, the monomer percentage (in red) decreased from ~99% to 98.5% (). Intermediate conditions with samples that were stored at 5°C for various days (5C1D, 5C5D, 5C9D) were also tested to show the trend in the plot. The aggregates seem to disassemble gradually to some extent while storing at 5°C for up to 19 d (5C19D) in the vial and remains unchanged till 30 d. Based on these data, it seems that F/T stress can induce reversible mAb aggregation. These F/T-induced aggregates can slowly dissociate at 5°C. Interestingly, it took about 2 weeks to completely recover these reversible aggregations back to the base level of existing protein aggregation in the solution.
Figure 10. Impact of protein aggregation by F/T stress followed by prolonged storage at 5°C by HPSEC. HMW species percentage is in blue, and the corresponding monomer percentage is in red for all time points labeled on the x-axis. Error represents the average assay variation on the same settings for measurement.

Alt text. The figure showcases percentages of protein HMW and monomer species in solution in the wake of freeze and thaw stress and extended 5°C storage at varied intervals. The graph validates the formation of reversible aggregates, as observed through wNMR.
Other biophysical attributes of dupilumab upon thermal and F/T stress
Native static light scattering (SLS) and fluorescence, measured using an UNCLE instrument, can probe protein aggregation temperature (Tagg) and the unfolding temperatures (Tm). The emission max signal at 473 nm (BCM, nm) was monitored over a temperature ramp of 20°C to 90°C for the control (non-stressed, in syringe), F/T stressed (4×, endpoint) and thermally stressed (50°C/2D/50°C 48 h, endpoint) samples (in syringe) at their original concentration of ~150 mg/mL by UNCLE. Comparable Tagg, Tm1, and Tm2 from native SLS were observed for all stressed samples compared to the control sample (Figure S7). In summary, for the samples upon F/T and accelerated thermal stress at 50°C, no significant difference on thermal profiles was observed in dupilumab from measurements using native SLS and fluorescence. This is likely due to the large population of monomers in these samples (>92% monomers quantified by HPSEC in ).
DLS was further used to evaluate the hydrodynamic size of the protein and melting profile of the control (non-stressed, in syringe), F/T stressed (4×, endpoint) and thermally stressed (50°C/2D/50°C 48 h, endpoint) samples (in syringe) at their original concentration at ~150 mg/mL (Figure S8). The size distribution by intensity vs. main species radius (nm) plot indicates that F/T has included a small population of larger particles (peak right shift and broadening), while stressing at 50°C over 2 d results in a broad distribution of particle sizes compared to that of the control sample. DLS melting profile comparison of control (non-stressed, in syringe), F/T (4×, endpoint) and thermal stress (50°C/2D/50°C 48 h, end point) indicates that slight differences were caused by F/T and the thermal stress compared to that of the control sample in protein unfolding profile using DLS melting with the comparable unfolding temperature at ~60°C.
An overlay of near-UV CD spectra of samples exposed to F/T and thermal stress in the syringe indicates similarity in their tertiary structure independent of the stress types (Figure S9A). The overlaid IR spectra and deconvoluted protein secondary structure analyses results of these samples in syringes indicate comparability between their secondary structure independent of the stress types (Figure S9B and Figure S8C). By investigating the subvisible particles in the stressed and control samples (Figure S10), F/T and thermal stress seem to induce more, but still an insignificant number of, subvisible particles in the sample compared to the control. This conclusion is supported by the observation that the particle counts at ≥10 µm and ≥25 µm are generally in the extremely low range (<600 particle counts per mL at ≥10 µm and <100 particle counts per mL at ≥25 µm).
Comparison of in situ wNMR and ex situ analytical techniques
Alt text. The figure displays normalized experimental data from wNMR, DLS, and HPSEC to facilitate side-by-side comparison. There is general agreement between the in situ wNMR and the ex situ DLS and HPSEC in detecting stress-induced mAb aggregation and, in the case of freeze and thaw stress, gradual dissociation of mAb aggregates.
It is interesting to compare wNMR, an in situ technique, with ex situ biophysical tools when evaluating mAb aggregation under stress conditions. As illustrated in , the normalized data and corresponding fit lines from both wNMR and DLS reveal a qualitatively consistent trend of increasing water relaxation times and mAb particle sizes, respectively, upon exposure to 50°C stress. Similarly, the trends observed between wNMR and HPSEC data for the F/T cycle stress and 5°C storage studies align closely (). These comparisons underscore the capability of wNMR to effectively report protein aggregation trends during stress studies in an in situ manner.
Figure 11. Comparison of in situ wNMR and ex situ analytical techniques. (a) in situ wNMR vs. ex situ DLS in detecting heat-induced mAb aggregation. To facilitate comparison, raw wNMR and DLS data are respectively normalized by the intercept values shown in Figure 5. (b) in situ wNMR vs. ex situ HPSEC in detecting F/T-induced mAb aggregation and gradual dissociation at 5°C. To facilitate comparison, raw wNMR (Figure 9) and HPSEC (Figure 10) data are, respectively, normalized by the data of the unstressed sample. See SI for details.

Discussion
Dupixent® stability assessed by wNMR and other biophysics techniques
Sterile liquid therapeutic protein products are subjected to physical stresses during formulation development, manufacturing, long-term and short-term storage, sample handling, and transportation.Citation41,Citation50,Citation51 These stresses, including heating, freezing, and F/T cycles, exacerbate protein aggregation in soluble and insoluble forms.Citation28,Citation29 Aggregation may be reversible or irreversible, influenced by protein sequences, aggregation stages, conformational changes, kinetics, and thermodynamics.Citation3,Citation52–55 This study investigates the impact of prolonged heating stress and F/T cycles on the mAb product Dupixent®, presented in PFS. The findings demonstrate the complexity of dupilumab’s aggregation behavior, shedding light on its time-dependent stability under stress conditions, and providing insights for optimizing storage and handling procedures to maintain therapeutic protein product quality and efficacy in clinical applications.
We conducted in situ heating stress of Dupixent® PFS for 48 h at 50°C, a temperature below the thermal unfolding point for this molecule at ~60°C (). At 50°C, there are aggregates slowly formed in the solution, which reached ~7% from the original ~1% (detected by SEC) at up to 48 h of heating (), which was reflected by the R2(Citation1H2O) value increase from about 7.307 s−1 to 7.377 s−1 (Figure S3) In addition, we have observed highly correlated growth of R2(Citation1H2O) to the average particle size (d.nm) during 48 h at 50°C by DLS (). These thermally induced aggregates are irreversible during the timeframe of our study. They do not dissociate significantly upon dilution (). Moreover, no apparent secondary nor tertiary structural changes for dupilumab under thermal stressing are detected using near-UV CD (). The aggregation is likely assembled by relatively strong non-covalent contacts between protein monomers,Citation3 and clusters with minor or no impact on their secondary nor tertiary structures, which is different from the protein–protein interaction that involves stoichiometric complexes of folded proteins.Citation3,Citation56,Citation57
F/T stress induces reversible aggregation, where aggregates immediately appear after F/T cycles but gradually disappear during storage at 5°C, as observed by both in situ wNMR () and ex situ HPSEC (). Factors that affect F/T-induced aggregation include product conditions like pH, ionic strength, and antibody concentration, and processing variables such as cooling and warming rates, multiple F/T cycles, and final temperature during thawing, drug product containers.Citation41 Dupilumab is formulated at a high concentration (150 mg/ml), which could exhibit an even higher concentration in the unfrozen fraction during freezing. The enhanced crowdedness can result in protein aggregation.Citation41,Citation58 Upon a period of recovery time at 5°C, these F/T-induced aggregates seem to disassociate into monomers.
As shown in , the comparison of wNMR, an in situ technique, with ex situ biophysical tools like DLS and HPSEC in mAb aggregation stress studies shows consistent trends in water relaxation times and particle sizes, highlighting wNMR’s effectiveness in reporting protein aggregation trends in situ during stress studies.
In situ Analyses of Drug Products Using wNMR
To implement real-time release testing (RTRT), which was defined as “the ability to evaluate and ensure the quality of in-process and final drug product based on process data, which typically includes a valid combination of measured material attributes and process controls” (ICH Q8[R2]), special attributes need to be taken into consideration, especially for any potential contamination or completion of the whole batch investigation.Citation66 The integrity of the product container and the direct measurement of protein at its original (high) concentration is the key to the in-situ characterization of the drug product without opening the container or diluting the analytes.Citation33,Citation35 However, in situ analyses of high-concentration therapeutic products using traditional release testing panel assays remain challenging due to the need for sample manipulation (transfer, dilution) and perturbation (formulation matrix modification, mobile phase impact) involved in these method protocols.Citation62,Citation67,Citation68 For example, multiple techniques, including SEC, DLS, front-face fluorescence (FFF) spectrophotometry, analytical ultracentrifugation (AUC), size-exclusion chromatography with multi-angle light scattering (SEC-MALS), HIAC, and MFI, can be used to detect protein aggregation and subvisible particle formation,Citation42,Citation43,Citation61,Citation69,Citation70 they are all ex situ measurements that either require the sample to be taken out of PFS or vials or testing probes to be inserted into these containers for analysis. Moreover, sample consumption in these “invasive” measurements can also be a concern when limited samples are available. compares several biophysical techniques used in this study with wNMR. Specifically, all these routine laboratory techniques, including DLS, in particular for kD measurement, IR featuring microfluidic modulation spectroscopy, CD, and DSC, and MFI, need dilution due to factors such as signal saturation, block of microfluidic transfer, limits on flow cell accommodation, column, and instrument limitation.
Table 1. Comparison of various biophysical techniques in the current study.
Vial-to-vial or batch-to-batch variations in drug products clearly pose a risk to product quality and safety. The connection of a potential adverse event to a specific defective vial cannot be established due to the product consumption after product administration.Citation46,Citation71,Citation72 The noninvasive and quantitative inspection capability of wNMR, however, provides the opportunity to possibly inspect product images (e.g., vials, syringes, injection pen, injection device) by both pharmaceutical and clinical users like researchers, manufacturers, nurses, and patients.Citation72–74 Benchtop wNMR allows containers to be positioned directly in the instrument sample holder and analyses can then be performed either as an endpoint or changes can be monitored in real-time.Citation29,Citation32,Citation33,Citation35,Citation72,Citation74–76 Even though the absolute content cannot be detected by wNMR, the information from R2(Citation1H2O) enables the confirmation of the product content uniformity and the detection of outlier dosage units (e.g., vials, PFSs, pens).Citation72 Previous research has demonstrated the capability of wNMR to detect vial-to-vial variability in freezing susceptibility within a single carton of vaccine vials with the advantages of a fast and noninvasive measurement, to avoid any potential administration of defective products to patients in clinics.Citation71 In addition, the water proton transverse relaxation rate measured by wNMR was found to be sensitive to detecting concentration deviations (possibly caused by filling error in the manufactural process) of the insulin FlexPen® product, in which the uncertainty of the drug product quantitative inspection can be significantly reduced, if not fully eliminated.Citation46,Citation77
In this study, wNMR successfully confirmed content uniformity, detected reversible aggregation upon dilution, irreversible aggregation upon prolonged stress at 50°C, and interesting recoverable molecular association upon F/T cycles. While theoretically two syringes having identical R2(1H2O) may be very different, with one having much less protein but much higher aggregate level with two factors canceling each other, this scenario is much less likely than the two syringes indeed having identical content. It is under this premise that matching R2(Citation1H2O) values of vials and syringes from the same batch is indicative of content uniformity. R2(Citation1H2O) is thus a useful tool for assessing the content uniformity of pharmaceutical products because dosage units in a batch (e.g., vials, syringes) are made to be as identical as possible. Most importantly, the detection of protein aggregation upon stress was performed in situ in real time.
In conclusion, this study demonstrated a good correlation between the data obtained through wNMR measurements and those acquired from other biophysical tools to detect reversible and irreversible aggregations in stressed protein samples. These technical comparisons strongly support the effectiveness of wNMR as a valuable biophysical tool for assessing protein aggregation in high-concentration formulation products, without compromising the integrity of the product. Moreover, it is critical to ensure stability throughout the entire shelf life of biological products, considering protein therapeutics are fragile and susceptible to post-release physical stresses.Citation47,Citation49,Citation52,Citation78,Citation79 NMR is increasingly utilized as a sophisticated biophysical tool for exploring drug delivery and the stability of peptide and protein drugs across various formulations, including sterile solutions, suspensions, lyophilized, and frozen solutions.Citation39,Citation80–85 In our study, the noninvasive nature of wNMR makes it a promising method for obtaining crucial information about protein stability in its original container, facilitating drug development and quality assessment processes.
Supportive information
Additional methods and samples for wNMR measurement, tables of wNMR data, glycerol-water mixtures, viscosity of Dupixent®, and figures of wNMR and biophysical results for glycerol-water mixtures and stressed mAb formulations.
KMAB-2023-0157R1_supp material_final.docx
Download MS Word (1.2 MB)Acknowledgments
We appreciate Merck colleagues including Drs. Qi Gao, Gennady Khirich, Jainik P. Panchal, Adam Sutton, Richard R. Rustandi, Yogita Krishnamachari, and Lei Zhu for their scientific discussions.
Disclosure statement
MBT and YBY are coinventors of patents on wNMR technologies owned by the University of Maryland Baltimore.
Supplementary material
Supplemental data for this article can be accessed online at https://doi.org/10.1080/19420862.2024.2304624.
Additional information
Funding
References
- Wang SS, Yan YS, Ho K. US FDA-approved therapeutic antibodies with high-concentration formulation: summaries and perspectives. Antib Ther. 2021;4(4):262–15. PubMed. doi:10.1093/abt/tbab027.
- Bramham JE, Davies SA, Podmore A, Golovanov AP. Stability of a high-concentration monoclonal antibody solution produced by liquid-liquid phase separation. MAbs. 2021;13(1):1940666. From NLM. doi:10.1080/19420862.2021.1940666.
- Roberts CJ. Protein aggregation and its impact on product quality. Curr Opin Biotechnol. 2014;30:211–17. From NLM. doi:10.1016/j.copbio.2014.08.001.
- Jiskoot W, Hawe A, Menzen T, Volkin DB, Crommelin DJA. Ongoing challenges to develop high concentration monoclonal antibody-based formulations for subcutaneous administration: Quo Vadis? J Pharm Sci. 2022;111(4):861–867. doi:10.1016/j.xphs.2021.11.008.
- Shire SJ. Formulation and manufacturability of biologics. Curr Opin Biotechnol. 2009;20(6):708–14. From NLM. doi:10.1016/j.copbio.2009.10.006.
- Strickley RG, Lambert WJ. A review of formulations of commercially available antibodies. J Pharm Sci. 2021;110(7):2590–608.e2556. From NLM. doi:10.1016/j.xphs.2021.03.017.
- Badkar AV, Gandhi RB, Davis SP, LaBarre MJ. Subcutaneous delivery of high-Dose/Volume biologics: Current status and prospect for future advancements. Drug Des Devel Ther. 2021;15:159–70. doi:10.2147/dddt.S287323. From NLM.
- Bittner B, Richter W, Schmidt J. Subcutaneous Administration of Biotherapeutics: an overview of Current challenges and opportunities. BioDrugs. 2018;32(5):425–40. From NLM. doi: 10.1007/s40259-018-0295-0.
- Harris RJ, Shire SJ, Winter C. Commercial manufacturing scale formulation and analytical characterization of therapeutic recombinant antibodies. Drug Dev Res. 2004;61(3):137–154. doi:10.1002/ddr.10344.
- Shire JS, G W, Bechthold-Peters K, Andya J. Current trends in monoclonal antibody development and manufacturing. New York: Springer AAPS Press; 2010. doi:10.1007/978-0-387-76643-0.
- Warne NW, Mahler HC. Challenges in Protein Product Development. AAPS Adv Pharm Sci Ser. 2018. doi:10.1007/978-3-319-90603-4.
- Treuheit MJK, Andrew A, Brems, David N. 2002. Inverse relationship of protein concentration and aggregation. Pharm Res. 19(4):511–16. doi: 10.1023/A:1015108115452.
- Holstein M, Hung J, Feroz H, Ranjan S, Du C, Ghose S, Li ZJ. Strategies for high-concentration drug substance manufacturing to facilitate subcutaneous administration: A review. Biotechnol Bioeng. 2020;117(11):3591–3606. doi:10.1002/bit.27510.
- Wang W, I AA, Ohtake S, Yang TC, Warne NW, Mahler H-C. Introduction to high-concentration proteins. Challenges in protein product development. AAPS-Springer. 2018;38:99–123.
- Yoneda S, Torisu T, Uchiyama S. Development of syringes and vials for delivery of biologics: current challenges and innovative solutions. Expert Opin Drug Deliv. 2021;18(4):459–70. From NLM. doi:10.1080/17425247.2021.1853699.
- Shire SJ, Shahrokh Z, Liu J. Challenges in the development of high protein concentration formulations. J Pharm Sci. 2004;93(6):1390–1402. doi:10.1002/jps.20079.
- Scherer TM. Cosolute effects on the Chemical Potential and interactions of an IgG1 monoclonal antibody at high concentrations. J Phys Chem B. 2013;117(8):2254–2266. doi:10.1021/jp3091717.
- Stetefeld J, McKenna SA, Patel TR. Dynamic light scattering: a practical guide and applications in biomedical sciences. Biophys Rev. 2016;8(4):409–27. From NLM. doi: 10.1007/s12551-016-0218-6.
- Wang L, Kendrick B, Ma E. Enhanced Protein Structural Characterization Using Microfluidic Modulation Spectroscopy. Spectroscopy. 2018;33:46–52.
- Ivancic VA, Lombardo HL, Ma E, Wikström M, Batabyal D. Advancing secondary structure characterization of monoclonal antibodies using microfluidic modulation spectroscopy. Anal Biochem. 2022;646:114629. doi:10.1016/j.ab.2022.114629.
- Kelly SM, Price NC. The use of circular dichroism in the investigation of protein structure and function. Curr Protein Pept Sci. 2000;1(4):349–84. From NLM. doi:10.2174/1389203003381315.
- Siligardi G, Hussain R, Patching SG, Phillips-Jones MK. 2014. Ligand- and drug-binding studies of membrane proteins revealed through circular dichroism spectroscopy. Biochimica et Biophysica Acta (BBA) - Biomembr. 1838(1, Part A):34–42. doi: 10.1016/j.bbamem.2013.06.019.
- Wei Y, Wahome N, Kumar P, Whitaker N, Picking WL, Middaugh CR. Effect of phosphate ion on the structure of lumazine synthase, an antigen presentation system from Bacillus anthracis. J Pharm Sci. 2018;107(3):814–823. doi:10.1016/j.xphs.2017.10.013.
- Chennamsetty NV, Vladimir K, Veysel T, Bernhardt L. 2009. Design of therapeutic proteins with enhanced stability. Proc Natl Acad Sci U S A. 106(29):11937–42. doi: 10.1073/pnas.0904191106.
- Allec N, Choi M, Yesupriya N, Szychowski B, White MR, Kann MG, Garcin ED, Daniel MC, Badano A. 2015. Small-angle X-ray scattering method to characterize molecular interactions: proof of concept. Sci Rep. 5(1):12085. doi: 10.1038/srep12085.
- Altkorn RZ, Richard N. 1984. Effects of saturation on laser-induced fluorescence measurements of population and polarization. Ann Rev Phys Chem. 35(1):265–89. doi: 10.1146/annurev.pc.35.100184.001405.
- Winters A, Cheong FC, Odete MA, Lumer J, Ruffner DB, Mishra KI, Grier DG, Philips LA. Quantitative differentiation of protein aggregates from other subvisible particles in viscous mixtures through holographic characterization. J Pharm Sci. 2020;109(8):2405–12. From NLM. doi:10.1016/j.xphs.2020.05.002.
- Demeule B, Messick S, Shire SJ, Liu J. Characterization of particles in protein solutions: reaching the limits of current technologies. AAPS J. 2010;12(4):708–715. doi:10.1208/s12248-010-9233-x.
- Taraban MB, DePaz RA, Lobo B, Yu YB. 2019. Use of water proton NMR to characterize protein aggregates: gauging the response and sensitivity. Anal Chem. 91(6):4107–4115. doi: 10.1021/acs.analchem.8b05733.
- Taraban MB, Briggs KT, Yu YB. 2020. Magnetic resonance relaxometry for determination of protein concentration and aggregation. Curr Protoc Protein Sci. 99(1):e102. doi: 10.1002/cpps.102.
- Taraban MB, Wang Y, Briggs KT, Yu YB. Inspecting insulin products using water proton NMR. I. Noninvasive vs invasive inspection. J Diabetes Sci Technol. 0(0):19322968211023806. doi:10.1177/19322968211023806.
- Taraban MB, DePaz RA, Lobo B, Yu YB. Water proton NMR: a tool for protein aggregation characterization. Anal Chem. 2017;89(10):5494–502. From NLM. doi:10.1021/acs.analchem.7b00464.
- Taraban MB, Truong HC, Feng Y, Jouravleva EV, Anisimov MA, Yu YB. Water proton NMR for in situ detection of insulin aggregates. J Pharm Sci. 2015;104(12):4132–41. From NLM. doi:10.1002/jps.24633.
- Feng Y, Taraban MB, Yu YB. 2015. Water proton NMR—a sensitive probe for solute association. Chem Commun. 51(31):6804–07. doi: 10.1039/C5CC00741K.
- Taraban MB, Truong HC, Ilavsky J, DePaz RA, Lobo B, Yu YB. 2017. Noninvasive detection of nanoparticle clustering by water proton NMR. Transl Mater Res. 4(2):025002. doi: 10.1088/2053-1613/aa7838.
- Solomon TL, Delaglio F, Giddens JP, Marino JP, Yu YB, Taraban MB, Brinson RG. Correlated analytical and functional evaluation of higher order structure perturbations from oxidation of NISTmAb. MAbs. 2023;15(1):2160227. doi:10.1080/19420862.2022.2160227.
- Yu YB, Feng Y, Taraban MB. Water proton NMR for noninvasive chemical analysis and drug product inspection. American Pharma Rev. 2017;20:34–39.
- Arbogast LW, Brinson RG, Marino JP. 2015. Mapping monoclonal antibody structure by 2D 13 C NMR at natural abundance. Anal Chem. 87(7):3556–3561. doi: 10.1021/ac504804m.
- Phyo P, Zhao X, Templeton AC, Xu W, Cheung JK, Su Y. 2021. Understanding molecular mechanisms of biologics drug delivery and stability from NMR spectroscopy. Adv Drug Deliv Rev. 174:1–29. doi:10.1016/j.addr.2021.02.007.
- Meiboom S, Gill D. Modified spin-echo method for measuring nuclear relaxation times. Rev. Sci. Instr. 1958;29:688–691.
- Kueltzo LA, Wang W, Randolph TW, Carpenter JF. Effects of solution conditions, processing parameters, and container materials on aggregation of a monoclonal antibody during freeze-thawing. J Pharm Sci. 2008;97(5):1801–12. From NLM. doi:10.1002/jps.21110.
- Muneeruddin K, Thomas JJ, Salinas PA, Kaltashov IA. Characterization of small protein aggregates and oligomers using size exclusion chromatography with online detection by native electrospray ionization mass spectrometry. Anal Chem. 2014;86(21):10692–99. From NLM. doi:10.1021/ac502590h.
- Philo JS. Is any measurement method optimal for all aggregate sizes and types? AAPS J. 2006;8(3):E564–71. From NLM. doi: 10.1208/aapsj080365.
- Ruesch MN, Benetti L, Berkay E, Cirelli DJ, Frantz N, Gastens MH, Kelley WP, Kretsinger J, Lewis M, Novick S, et al. Strategies for setting patient-centric commercial specifications for biotherapeutic products. J Pharm Sci. 2021;110(2):771–784. doi:10.1016/j.xphs.2020.09.048.
- Hills BP, Takacs SF, Belton PS. The effects of proteins on the Proton Nmr Transverse Relaxation-Times of water .1. Native Bovine Serum-Albumin. Mol Phys. 1989;67(4):903–18. doi:10.1080/00268978900101531.
- Briggs KT, Taraban MB, Wang W, Yu YB. Nondestructive quantitative inspection of drug products using benchtop NMR relaxometry-the case of NovoMix® 30. Aaps Pharm Sci Tech. 2019;20(5):189. From NLM. doi:10.1208/s12249-019-1405-0.
- Allmendinger A, Mueller R, Huwyler J, Mahler HC, Fischer S. 2015. Sterile filtration of highly concentrated protein formulations: impact of protein concentration, formulation composition, and filter material. J Pharm Sci. 104(10):3319–3329. doi: 10.1002/jps.24561.
- Grasso R, Musumeci F, Gulino M, Scordino A, Khodarahmi R. 2018. Exploring the behaviour of water in glycerol solutions by using delayed luminescence. PloS ONE. 13(1):e0191861. doi: 10.1371/journal.pone.0191861.
- Segur JB, Oberstar HE. 1995. Viscosity of glycerol and its aqueous solutions. Ind Eng Chem. 43(9):2117–20. doi: 10.1021/ie50501a040.
- Manning MC, Chou DK, Murphy BM, Payne RW, Katayama DS. Stability of protein pharmaceuticals: an update. Pharm Res. 2010;27(4):544–75. From NLM. doi: 10.1007/s11095-009-0045-6.
- Singh SK, Kolhe P, Mehta AP, Chico SC, Lary AL, Huang M. Frozen state storage instability of a monoclonal antibody: aggregation as a consequence of trehalose crystallization and protein unfolding. Pharm Res. 2011;28(4):873–85. From NLM. doi: 10.1007/s11095-010-0343-z.
- Wu H, Kroe-Barrett R, Singh S, Robinson AS, Roberts CJ. Competing aggregation pathways for monoclonal antibodies. FEBS Lett. 2014;588(6):936–41. From NLM. doi:10.1016/j.febslet.2014.01.051.
- Banks DD, Latypov RF, Ketchem RR, Woodard J, Scavezze JL, Siska CC, Razinkov VI. Native-state solubility and transfer free energy as predictive tools for selecting excipients to include in protein formulation development studies. J Pharm Sci. 2012;101(8):2720–32. From NLM. doi:10.1002/jps.23219.
- Roberts CJ. Non-native protein aggregation kinetics. Biotechnol Bioeng. 2007;98(5):927–38. From NLM. doi:10.1002/bit.21627.
- Caflisch A. Computational models for the prediction of polypeptide aggregation propensity. Curr Opin Chem Biol. 2006;10(5):437–44. From NLM. doi:10.1016/j.cbpa.2006.07.009.
- Bou-Abdallah F, Terpstra TR. The thermodynamic and binding properties of the transferrins as studied by isothermal titration calorimetry. Biochim Biophys Acta. 2012;1820(3):318–25. From NLM. doi:10.1016/j.bbagen.2011.07.013.
- McPherson A, Gavira JA. Introduction to protein crystallization. Acta Crystallogr F Struct Biol Commun. 2014;70(1):2–20. From NLM. doi: 10.1107/s2053230x13033141.
- Heller MC, Carpenter JF, Randolph TW. Protein formulation and lyophilization cycle design: prevention of damage due to freeze-concentration induced phase separation. Biotechnol Bioeng. 1999;63(2):166–74. acccessed 2022/10/20. doi:10.1002/(SICI)1097-0290(19990420)63:2<166:AID-BIT5>3.0.CO;2-H.
- Mirasol F. Stability testing of protein therapeutics using DLS. BioPharm International, BioPharm International. 2021;34:41–43.
- Esfandiary R, Parupudi A, Casas-Finet J, Gadre D, Sathish H. Mechanism of reversible self-association of a monoclonal antibody: role of electrostatic and hydrophobic interactions. J Pharm Sci. 2015;104(2):577–586. doi:10.1002/jps.24237.
- Fekete S, Beck A, Veuthey JL, Guillarme D. Theory and practice of size exclusion chromatography for the analysis of protein aggregates. J Pharm Biomed Anal. 2014;101:161–73. doi:10.1016/j.jpba.2014.04.011. From NLM.
- Hong P, Koza S, Bouvier ES. Size-exclusion chromatography for the Analysis of Protein Biotherapeutics and their aggregates. J Liq Chromatogr Relat Technol. 2012;35(20):2923–50. From NLM. doi:10.1080/10826076.2012.743724.
- Liu J, Nguyen MD, Andya JD, Shire SJ. Reversible self-association increases the viscosity of a concentrated monoclonal antibody in aqueous solution. J Pharm Sci. 2005;94(9):1928–40. From NLM. doi:10.1002/jps.20347.
- Sharma DK, King D, Oma P, Merchant C. Micro-flow imaging: flow microscopy applied to sub-visible particulate analysis in protein formulations. AAPS J. 2010;12(3):455–64. From NLM. doi: 10.1208/s12248-010-9205-1.
- Taraban MB, Wang Y, Briggs KT, Yu YB. Inspecting insulin products using water proton NMR. I. Noninvasive vs invasive inspection. J Diabetes Sci Technol. 2021:19322968211023806. From NLM. doi:10.1177/19322968211023806.
- Jiang M, Severson KA, Love JC, Madden H, Swann P, Zang L, Braatz RD. Opportunities and challenges of real-time release testing in biopharmaceutical manufacturing. Biotechnol Bioeng. 2017;114(11):2445–56. From NLM. doi:10.1002/bit.26383.
- Kaur H, Beckman J, Zhang Y, Li ZJ, Szigeti M, Guttman A. Capillary electrophoresis and the biopharmaceutical industry: therapeutic protein analysis and characterization. TrAC Trend Anal Chem. 2021;144:116407. doi:10.1016/j.trac.2021.116407.
- Challener CA. Tackling the challenge of HOS determination. BioPharm International. 2014;27(2):20–24.
- Liu J, Andya JD, Shire SJ. A critical review of analytical ultracentrifugation and field flow fractionation methods for measuring protein aggregation. AAPS J. 2006;8(3):E580–89. From NLM. doi: 10.1208/aapsj080367.
- Giddings JC. Field-flow fractio nation: analysis of macromolecular, colloidal, and particulate materials. Sci. 1993;260(5113):1456–65. From NLM. doi: 10.1126/science.8502990.
- Briggs KT, Taraban MB, Yu YB. Quality assurance at the point-of-care: noninvasively detecting vaccine freezing variability using water proton NMR. Vaccine. 2020;38(31):4853–4860. doi:10.1016/j.vaccine.2020.05.049.
- Bruce Yu Y, Taraban MB, Briggs KT. All vials are not the same: potential role of vaccine quality in vaccine adverse reactions. Vaccine. 2021;39(45):6565–69. From NLM. doi: 10.1016/j.vaccine.2021.09.065.
- Yu YB, Taraban MB, Wang W, Briggs KT. Improving biopharmaceutical safety through Verification-Based Quality Control. Trends Biotechnol. 2017;35(12):1140–55. From NLM. doi:10.1016/j.tibtech.2017.08.010.
- Yu LX, Kopcha M. The future of pharmaceutical quality and the path to get there. Int J Pharm. 2017;528(1–2):354–59. From NLM. doi:10.1016/j.ijpharm.2017.06.039.
- Telikepalli SN, Kumru OS, Kalonia C, Esfandiary R, Joshi SB, Middaugh CR, Volkin DB. Structural characterization of IgG1 mAb aggregates and particles generated under various stress conditions. J Pharm Sci. 2014;103(3):796–809. From NLM. doi:10.1002/jps.23839.
- Yu YB, Briggs KT, Taraban MB, Brinson RG, Marino JP. Grand Challenges in pharmaceutical research series: ridding the cold chain for biologics. Pharm Res. 2021;38(1):3–7. From NLM. doi: 10.1007/s11095-021-03008-w.
- Rosenberg AS, Verthelyi D, Cherney BW. Managing uncertainty: a perspective on risk pertaining to product quality attributes as they bear on immunogenicity of therapeutic proteins. J Pharm Sci. 2012;101(10):3560–67. From NLM. doi:10.1002/jps.23244.
- Yu YB, Briggs KT, Taraban MB. Preventive pharmacovigilance: timely and precise prevention of adverse events through person-level patient screening and dose-level product surveillance. Pharm Res. 2023. doi:10.1007/s11095-023-03548-3.
- Chen K, Cheung JK, Kim HY, Leone A, Mallela K, Su YC. 2023. Enabling efficient design of biological formulations through advanced characterizations. Pharm Res. 40(6):1313–16. doi: 10.1007/s11095-023-03557-2.
- Li M, Falk BT, Lu X, Schroder R, McCoy M, Xu W, Yin DH, Gindy ME, D’Addio SM, Su Y. 2022. Molecular Mechanism of Antimicrobial Excipient-Induced Aggregation in Parenteral Formulations of Peptide Therapeutics. Mol Pharm. 19(9):3267–3278. doi: 10.1021/acs.molpharmaceut.2c00449.
- Li M, Reichert P, Narasimhan C, Sorman B, Xu W, Cote A, Su Y. 2022. Investigating Crystalline Protein Suspension Formulations of Pembrolizumab from MAS NMR Spectroscopy. Mol Pharm. 19(3):936–952. doi: 10.1021/acs.molpharmaceut.1c00915.
- Li M, Koranne S, Fang R, Lu X, Williams DM, Munson EJ, Bhambhani A, Su Y. 2021. Probing Microenvironmental Acidity in Lyophilized Protein and Vaccine Formulations Using Solid-state NMR Spectroscopy. J Pharm Sci. 110(3):1292–1301. doi: 10.1016/j.xphs.2020.11.017.
- Du Y, Li J, Xu W, Cote A, Lay-Fortenbery A, Suryanarayanan R, Su Y. 2023. Solid-State NMR Spectroscopy to Probe State and Phase Transitions in Frozen Solutions. Mol Pharm. 20(12):6380–6390. doi: 10.1021/acs.molpharmaceut.3c00764.
- Chen Y, Ling J, Li M, Su Y, Arte KS, Mutukuri TT, Taylor LS, Munson EJ, Topp EM, Zhou QT. 2021. Understanding the impact of protein-excipient interactions on physical stability of spray-dried protein solids. Mol Pharm. 18(7):2657–68. doi: 10.1021/acs.molpharmaceut.1c00189.
- Mutukuri TT, Ling J, Du Y, Su Y, Zhou QT. Effect of Buffer salts on physical stability of lyophilized and spray-dried protein formulations containing bovine serum albumin and trehalose. Pharm Res. 2023;40(6):1355–1371. doi:10.1007/s11095-022-03318-7.