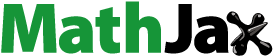
Abstract
In order to meet sustainable development goals, global policies are strongly oriented towards the recovery of local materials such as agricultural waste. In this context, a new biosourced compressed earth brick (CEB) has been produced with bio-shell aggregates of Cocos nucifera (CN) and Canarium schweinfurthii (CS), added in equal proportions for various mixtures. The main objective is to study, on one hand, the effect of CN and CS aggregates on the mechanical behaviour and the thermo-physical properties of the CEB. On the other hand, durability of CEB was investigated in terms of hydric stability, abrasion resistance and hygro-thermo-mechanical behaviour. Mixtures were prepared using earthen material and 0, 5, 10 and 15 wt% CNCS, with 8 wt% cement CEM II-B-LL 42.5, respectively. Wet and dry compressive strengths are evaluated versus compaction pressure (2.5, 5, 7 and 10 MPa). The increase of CNCS aggregate content induced a reduction of the compressive strength in both wet a\nd dry situations whatever the compaction pressure. The samples with 5 wt % CNCS and 8 wt % cement, however, offered performances close to reference samples without CNCS aggregates. The reverse trend was found for thermal conductivity: thermal conductivity of the bio-sourced CEB is below 1 W·m−2·K−1 which means good insulating performances. It is noted that the modified CEB have relatively low water absorption, which contribute to a good durability. Hygro- and thermo-mechanical tests showed however a quasi-linear decrease of compressive strength when the humidity of the environment increases but also when the temperature rises. At the end, to obtain more accurate properties by prediction, some machine learning models were used.
PUBLIC INTEREST STATEMENT
This research demonstrated that biosourced compressed earth brick (CEB) can be easily produced to solve the situation of housing in the world. In this context, the mix of earth and natural biobased by-products largely available in Cameroon seems to be a good solution for buildings. The present work is focused on the use of Cocos nucifera and Canarium schweinfurthii aggregates in CEB. Resource is estimated at 2000 tons/year. The research question concerns the effect of these bio-aggregates on mechanical, physical, thermal and durability properties of CEB. Resolutely focused on the continent’s green growth and convinced that eco-materials offer significant opportunities in terms of business creation and jobs, this study proposes to formulate and characterize bricks of compressed, stabilized and biosourced with Cocos nucifera and Canarium schweinfurthii aggregates.
1. Introduction
The earth material is considered to be an efficient response to housing problems due to its availability, its reasonable cost and its eco-friendly behaviour in front of the so-called modern materials as cement (CRAterre, Houben & Guillaud, Citation2006; Guillaud et al., Citation1995). The energy costs associated with construction and especially the building sector represent the second highest human activity after manufacturing (Omer, Citation2008). The Intergovernmental Panel on Climate Change (IPCC) for the UN member countries indicates that the building sector is responsible for almost 9.18 Gt of CO2 greenhouse gas (GHG) emissions, i.e. about one-fifth of the total emissions of all other sectors combined. This is also the second highest energy expenditure (Smith et al., Citation2009). Therefore, the development of sustainable building practices is essential, not only to comply with current greenhouse gas emission reduction targets but also to limit energy consumption on a global scale (Ahn et al., Citation2013; Akadiri, Citation2011; Akadiri & Olomolaiye, Citation2012; Bunz et al., Citation2006; Häkkinen & Belloni, Citation2011).
In this context of economic and sustainable housing availability, the development of building materials that are energy efficient but also have low environmental impacts on resource depletion appears to be a promising challenge for the present millennium. The earth material is currently positioning itself as a sustainable solution for both residential and office buildings (Reeves et al., Citation2006).
One of the main reasons for using earth as a building material is that it is usually locally available (Adam and Agib Citation2017; Gallipoli et al., Citation2017; Molina, Citation2016; Mostafa, Citation2016; Sore et al., Citation2018; Taallah et al., Citation2014), it requires low energy to manufacture and it can be fully recycled (CRAterre, Houben & Guillaud, Citation2006; Fontaine & Anger, Citation2010), depending on the additions (Adam and Agib Citation2017; Moussa et al., Citation2019; Saidi et al., Citation2018; Sore et al., Citation2018; Taallah et al., Citation2014).
Several standards are proposing the compression strength as a reference for the classification of CEB, even if many other tests as flexural test, water absorption, abrasion and erosion, are valuables. The minimum required values of the compressive strength are 2 and 1 MPa, in dry and wet states, respectively, according to most of the standards (Figure ). For a given standard, if the mechanical criterion is not met, then it is necessary to stabilize the earthen material.
Figure 1. Minimal values of dry and wet compressive strengths of CEBs according to different standards: IS 1725 (India), NBR (Brazil), ARSO (Africa), NT (Tunisia), NZS (New Zealand), KS 02–1070 (Kenya), XP P13-901 (France), NTC 5324 (Colombia), UNE 41410 (Spain), SLS 1382 (Sri Lanka), ASTM E2392M-10 (USA), NMAC 14.7.4 (New Mexico); inspired byCid-Falceto et al. (Citation2012).

Stabilization is usually necessary to improve the mechanical and hygroscopic performances of CEB (CRAterre, Houben & Guillaud, Citation2006). It should be noted that the average content of stabiliser varies according to the mineralogical properties of the earth; it is generally between 8% and 10% for both lime and cement (Nagaraj et al., Citation2014; Nshimiyimana et al., Citation2019, Citation2018; Saidi et al., Citation2018).
The earthen material can be enhanced by increasing the compaction pressure, which induces an increase of the CEB density, the Young’s modulus and mechanical strength (Bruno et al., Citation2015). It, however, induces the decrease of hydrophilic potentials and insulating properties (Guettala et al., Citation2016). Regardless of the type of material, there is a quasi-linear variation of the Young’s modulus and the compressive strength as a function of the density of the CEB (Bruno et al., Citation2015). Densification of the material also has the advantage of reducing the rate of water absorption, which promotes its durability (Guettala et al., Citation2016). Some research works are also proposing a granular correction to improve the performance of CEB. For a plastic earth, it is advisable to add sand; for a non-plastic earth, it is advisable to add fine particles (Fontaine & Anger, Citation2010). The work of Izemmourena and Guettala (Citation2014) indicates the improvements of the compressive strength by 13%, 28%, 30%, and 32% with addition of 10%, 20%, 30% and 40% of sand, respectively, in a CEB stabilized with 5% cement for a compaction pressure of 10 MPa (O. Izemmourena & Guettala, Citation2014).
Cement and lime are also widely used to enhance the performances of brick. Usually, at least 5% to 6% cement is needed to obtain satisfactory results (CRAterre, Houben & Guillaud, Citation2006; Meukam, Citation2004). Houben et al. (Citation1998) proposed a threshold percentage of 8% to satisfy both mechanical and economic conditions (Houben et al., Citation1998), they observed an increase of the mechanical strength, the thermal conductivity and a decrease of the water absorption. These are due to the densification of the material by adding cement fines.
For stabilization, Guillaud (Citation1995) recommends the use of lime for less clayey soils with fraction of clay less than 20% (Guillaud et al., Citation1995). Le Roux (Citation1969) also proved that the mechanical resistance of kaolinite-type clays increases with 5% of lime content, while for illite and smectite-type clays, the ratios are chosen between 3% and 12% of lime content. The bulk densities of CEB were reduced by about 10%, while the mechanical resistance increased by about 50% (Le Roux, Citation1969).
Recent studies have already showed that CEB can be stabilized by using by-products (Masuka et al., Citation2018; Nshimiyimana, Citation2020; Obianyo et al., Citation2021). The main issue of adding biobased by-products (as fibers or aggregates) as a stabilizer for CEB, is the reduction of the environmental impact of brick. The improvement of compressive strength and the reduction of water absorption of CEB are reported by Masuka et al. (Citation2018) when CEB is stabilized with coal fly ash combined with lime (Masuka et al., Citation2018). Muntohar (Citation2011) found that, at an equal proportion of rice husk ash and lime, very high mechanical resistance (20.7 MPa) was obtained (Muntohar, Citation2011). The rice husk ash is therefore presented as a by-product with sufficient pozzolanic activity for industrial applications.
In the same, Nshimiyimana et al. (Citation2018), (Citation2020) promote the use of carbide residues combined with rice husk ash. With ratios of 10% and 20% of the new binder, they obtained an increase of compressive strength (1.1 to 8.3 MPa), a decrease in bulk density (2.03 to 1.61 g/cm3) and similarly a decrease in thermal conductivity (0.83 to 0.64 W/mK; Nshimiyimana et al., Citation2020, Citation2018).
Stabilization is also carried out by adding plant by-products (biosourced products) like fibres or aggregates from hard shells and cores, sugar cane molasses, fibre from plantain banana stalk, cork aggregates, etc. (Ganou Koungang et al., Citation2019a, Citation2019b; Guettala et al., Citation2016; Laborel-Préneron et al., Citation2016).
The addition of fibers and biobased aggregates leads to a reduction in the bulk density of the brick. This is because their densities (400–1600 kg·m-3; Laborel-Préneron et al., Citation2016) are lower than that of soil (~2600 kg·m-3; Reeves et al., Citation2006). Algin showed approximately 29% decrease in density for an addition of 40% by volume of cotton fiber residue (Algin & Turgut, Citation2008). The work of Al Rim et al. (Citation1999) demonstrated a linear decrease of the density of CEBs loaded with woodchips and stabilized with cement, and the density decreased by 63% when the percentage of chips varied from 10% to 50% (Al Rim et al., Citation1999). Since porosity is closely related to the absorption capacity of materials, CEB becomes more hydrophilic with the addition of fibers/aggregates. Taallah and Guettala (Citation2016) also showed that increasing the fiber content of date palms by 0.2% led to a 10.5% increase of the water absorption. This work was carried out with cement stabilization and at compaction pressure of 10 MPa (Taallah & Guettala, Citation2016). For mechanical performance, Abessolo et al. (Citation2020) found that the compressive strength ranges between 4.4 and 11.6 MPa, for bricks stabilized with Bambusa vulgaris fiber (0.5% for 4 cm of length; Abessolo et al., Citation2020). Likewise, Giroudon et al. (Citation2019) compared the effect of using barley and lavender branch aggregates (3% and 6%, respectively) in earth bricks. They reported a decrease in mechanical strength as well as Young’s modulus. The reduction of the mechanical strength is, respectively, 31% and 21% for barley aggregate and 23% and 19%, respectively, for lavender aggregates. The reduction was greater for Young’s moduli about 86% and 94%, respectively, for barley and 73% and 87%, respectively, for 3% and 6% lavender (Giroudon et al., Citation2019).
For the flexural strength, studies show an improvement with the addition of plant aggregates, especially when these are in the shape of fibers. Reinforcement rates of 5%, 7.5% and 10% (Stanislas et al., Citation2021) with bamboo pulp induced an increase in the modulus of rupture and conversely a decrease of the Young's modulus in flexion; they found an optimum with 7.5% content by weight. Likewise, the insertion of recycled cardboard pulp fibers improved flexural strength between 13% and 56% in the dry state. Thermal parameters are also important in the choice of construction materials for comfort purposes. It has frequently been shown that an increase in the aggregate or fiber content leads to a decrease in thermal conductivity. Al Rim et al. (Citation1999) showed a linear decrease in thermal conductivity of earth bricks with cement stabilized wood chips. The thermal conductivity varied from 0.24 to 0.08 W·m−1·K−1 when the percentage of chips varied from 10% to 50% (Al Rim et al., Citation1999).
Despite the applicability of practical methods, the limitation concerning the conditions, cost, time and direct testing equipment should be taken into account. This is the reason why indirect approaches using traditional statistical methods (Abbaszadeh Shahri et al. Citation2016, Chithra et al. Citation2016, Sitton et al. Citation2017, Ben Chaabene et al. Citation2020, Mahamat et al., Citation2021) and then the high computational power modeling techniques like artificial neural networks (ANN), multi-linear (ML) techniques or support vector machines (SVM) have been emphasized (Kahraman Citation2001, Yasar and Erdogan Citation2004, Ferentinou and Fakir Citation2017, Asheghi et al. Citation2019, Abessolo et al., Citation2020)
In this context, the mix of earth and natural biobased by-products largely available in Cameroon seems to be a good solution for buildings. The present work is focused on the use of Cocos nucifera and Canarium schweinfurthii aggregates in CEB. These agricultural by-products are available in public places as waste, estimated at 2000 tons/year (CIRAD, Citation2006). The research question addressed in this study concerns the effect of these bio-aggregates on mechanical, physical, thermal and durability properties of CEB.
2. Materials and methods
2.1. Materials: Characterization and mixes
The tested samples are stabilised and biosourced compressed earth bricks composite. The composite is a mixture of earth material, CEM II-B-LL 42.5, Cocos nucifera and Canarium schweinfurthii (CNCS) aggregates.
The kaolinite clay (Table ) with a specific sandy clayey silt earth type comes from the city of Douala (4°05, 9°74; Figure ). This city is famous for having a hot and humid climate, because of its proximity to the Atlantic Ocean and its position at the equator. Its suitability for the production of CEB is defined by using the CRAterre abacuses (Figure ; Ganou Koungang, n.d.apeu, Tchemou et al., Citation2020). This raw material is extracted on the University of Douala site at a depth of 0.5 m in the layer between 0.5 m and 10 m of the lithological structure of the site soil (Figure ). Once removed from the site, the soil is crushed to eliminate the lumps and conditioned afterwards in a ventilated oven (Memmert brand) at a temperature of 105°C. After 24 hours, a stabilization of the mass after successive weighing (NF P94-050, Citation1995) was observed. In this anhydrous state, the water content has been evaluated at 10%. This value will be considered as the initial water content of the soil sample at the beginning for all upcoming tests.
Figure 3. Sieving curve of the Douala earth in the CRAterre spindle (Ganou Koungang, n.d.apeu, Tchemou et al., Citation2020).

Table 1. Identification of earth from Douala
The biosourced mixture was produced by adding CNCS aggregates in equal-proportion (50:50 of CN and CS) based on the weight of the earthen material. The CNCS aggregates were used in a water-saturated state. The CN shells and CS cores (Figure ) are firstly collected as waste to the public place of the city of Douala. A representative quantity of the national resource of 250 kg was used. CNCS aggregates are obtained by crushing materials with a hammer mill and a 6 mm output size; the shredded materials are then sieved to obtain a 2/4 mm granular class. The aggregates were used in the proportions of 0%, 5%, 10% and 15% as an addition to the mass of the earth material.
The wear resistance of CNCS aggregates was also characterized (Table ) by using the dynamic fragmentation protocol (NF P18-574, Citation1990). The thermal conductivity was also evaluated using the hot wire method (ASTM D5334–00, 2000). These data indicate that materials are considered as light aggregates with very low thermal conductivity and good wear resistance.
Table 2. Physical characteristics of the CNCS aggregates
The choice of cement as a stabiliser is established using the diagram crossing the clay fraction of the soil with the plasticity index (Figure ).
The codification chosen for the samples was CEB_CNCS_x-y with the coefficients x and y representing the mass proportion of cement and CNCS aggregates based on the earth mass, respectively (Table ). The manufacturing process followed several steps including preparation, mixing (Figure ), compaction and curing. The tests were carried out after 28 days of curing according to ASTM D1632–07 standard (D1632–07, Citation2007).
Table 3. Composition of CEB made with CNCS aggregates (%)
As water is an essential element of the mechanical performances of CEB, it was fixed in accordance with the results of the Proctor test (NF P94-093, Citation2014; Figure ) carried out on the 5 mixes.
The compaction pressure was varying at 2.5 MPa, 5 MPa, 7 MPa and 10 MPa and was carried out using a manually operated hydraulic press (calibrated M&O brand).
2.2. Properties of compressed earth bricks
2.2.1. Mechanical characterization
Mechanical performances were measured using an Instron Series 5585 universal mechanical testing machine. For each experiment, 10 samples were tested after 28 days of curing on the laboratory conditions (30 2°C and 90
5% RH).
2.2.1.1. Flexural test
These tests are carried out according to EN 12372 (2007) at 222°C and 70 5% RH, with a loading speed of 1 mm/min (Figure ).
The samples (220 mm × 110 mm × 40 mm) were assimilated to isotropic materials, in order to have a Young’s modulus and a bending stress of rupture considered identical in all directions of the material. The Young’s modulus (EquationEq. 1)(1)
(1) is obtained by developing the material strength equation based on the calculation of the deflection at the central point (C; Figure ).
where F: force (in N), represents the slope at the linear part of the curve F = f (Δe; Figure ).
The 3-points bending strength, on the other hand, is calculated by equation (EquationEq. 2(2)
(2) )
with Ff.max is the maximum bending load (in N; Figure ).
2.2.1.2. Dry and wet compressive strengths
The two pieces of bricks obtained after the bending test were used to measure compressive strength (Figure ) with a section of 100 × 100 mm2. The test was conducted according to the protocol of EN 196–1 (NF EN 196–1, Citation2016) with a loading rate of 2 kN/min.
The Young’s modulus in compression (EquationEq. 3)(3)
(3) is obtained from the traditional Hooke’s equation.
knowing that S represents the compressed section of the compression cubes.
with F: load (in N), represents the slope at the linear part of the curve F = f (Δe; Figure ).
In the case of “wet” compression, samples remained fully immersed for 2 hours after curing period and compression tests were carried out directly after removing.
2.2.2. Physico-thermal characterization
The asymmetric hot plane method (Mvondo Ngono et al., Citation2017; Ngohe-Ekam et al., Citation2006; Nitcheu et al., Citation2018; Nshimiyimana et al., Citation2020) is the testing tool used to evaluate the physico-thermal characteristics of CEB: this is a transient characterization approach used to obtain the apparent thermal conductivity of a material by estimating the thermal effusivity E and the volumetric heat capacity ρCP versus the test temperature Texp(t) and the modelled temperature Tmod(t).
The test devices are shown in Figure . Samples are placed on a heat sensor between two (10 cm × 10 cm × 5 cm) blocks of polystyrene. A generator is used to heat the resistor. The increase in temperature at the centre of the thermal resistance is due to a type K thermocouple which records the test temperature on the hot surface of the material. The Texp(t) were recorded using the TC08-USB Picolog acquisition module. The drop in experimental and simulated temperature obtained after modelling the test instrument was used to estimate E and ρCP.
It is important to note that the temperature above the polystyrene blocks remains in its initial state. In order to achieve this, two aluminium blocks of 10 cm × 10 cm × 4 cm are placed above and below the polystyrene blocks.
The pre-estimated value of thermal effusivity (EquationEq. 4)(4)
(4) was obtained from the 1D numerical calculation of the slope δ(t) of the linear part of the experimental curve
.
where E: thermal effusivity of the sample (J·m−2·K−1·s1/2), Epo: thermal effusivity of the polystyrene (J·m−2·K−1·s1/2), Ф: heat flux produced in the heating element (W).
It was also possible to evaluate the pre-estimated value of the volumetric heat capacity (EquationEq. 5)(5)
(5) .
where e: thickness (m), C: thermal capacity (J·m−2·K−1·s1/2), ρ: bulk density (g/cm3), po: symbol of insulating material: polystyrene, h: symbol of heating material: polystyrene, β: slope of linear part of experimental curve .
Calculations of thermal conductivity (λ) and diffusivity (a) are carried out in EquationEq. (6)(6)
(6) and EquationEq. (7
(7)
(7) ), respectively.
2.2.3. Durability analysis
2.2.3.1. Abrasion resistance
The abrasion resistance of CEB is carried out according to the experimental standard NF XP P13-901 (XP P13-901, Citation2001). The objective is to test the brick to friction with a metallic brush (Figure .a) of 2.5 cm width. The rate is 1 return per second. The abrasion coefficient (Ca; EquationEq. 8)(8)
(8) of the brick represents the loss of material linked to the brushing of the brick on the abrasion surface.
Figure 14. Abrasion resistance test.
with, Ca: brick abrasion coefficient (cm2/g); S: brick abrasion surface (cm2; Figure .b); m0: initial mass of the brick before abrasion (g); m1: mass of the brick after the abrasion test (g).
The experimental standard indicates basic coefficients allowing to classify a CEB according to its abrasion resistance.
2.2.3.2. Resistance to water
The capillary absorption test consists of registering the mass variation of a block placed in a water bath, on a support to submerge only 5 mm of the thickness of the brick. The mass in dry state is measured (m1). After 10 minutes, the blocks are removed from the bath, wiped with a small dry towel and weighed to obtain the mass after immersion m2. This operation was carried out until the trend stabilised.
The capillary absorption coefficient Cb is determined by EquationEq. (9)(9)
(9) .
With, m2: wet mass (g), m1: dry mass (g), S: submerged surface area (cm2), t: immersion time (min).
The total water absorption (TWA) after 24 hours consists of weighing the mass of the blocks in the dry state (m1) and after 24 hours’ total immersion (m4). The blocks are completely immersed into a water bath. After 24 hours, the blocks are removed from the bath and wiped with a small dry towel and weighed to obtain the mass after immersion m4.
The total water absorption coefficient WA24 is given by EquationEq. 10(10)
(10) :
2.2.4. Hydro- and thermomechanical coupling
For the hydromechanical coupling, the bricks were conditioned for 24 hours in four adiabatic enclosures consisting of hermetically sealed boxes each containing a supersaturated aqueous saline solution. The salts used are potassium hydroxide (KOH), sodium dichromate (Na2Cr2O7), sodium chloride (NaCl) and potassium sulphate (K2SO4). Table shows the estimated moisture content in the boxes as a function of the salts at a temperature of 30°C (NF X15-119, Citation1999). The humidity control is carried out by Hobo data loggers (U12-012). The samples were immediately subjected to the compression and thermal tests after removing from the conditioning boxes.
Table 4. Saline solutions and corresponding relative humidity value in the boxes
For the thermomechanical coupling, the bricks were conditioned for 24 hours in a ventilated oven (UF 110) at temperature ranges of 30°C, 50°C, 70°C and 90°C, respectively. The samples were immediately subjected to compression and thermal tests after removing from the oven.
2.2.5. Prediction and sensibility analysis
In this section, the aim is to predict the mechanical strength of the earth brick, as a function of the proportion of cement stabiliser, OWC, clay fraction of soil, compaction pressure and dry bulk density of the brick. The training (30% of the input data) and test (70% of the input data) data for the development of linear regression (LR), support vector machines (SVM) and neural network (NN) models were prepared from primary experimental data sets conducted during this investigation. The experiment was implemented in Jupyter Python software. Default parameters were used for the SVM and the LR models, but for NN, random setup was 2.
3. Results and discussion
3.1. Dry and wet compressive strength
As a general trend, the compressive strength increases with increasing compaction pressure for both dry and wet specimens (Figure ). Stabilised CEB samples with cement content of 8% were compacted at the pressure of 2.5 MPa, 5 MPa, 7 MPa and 10 MPa, respectively. From the lowest to the highest compacted pressure (variation of 8 MPa), a threefold increase in dry compression strength and a twofold increase in wet compression strength were observed.
Figure 15. Evolution of compressive strength as a function of compaction pressure for dry and wet CEB samples.

Figure shows that, for the same cement content, samples without CNCS (CEB_CNCS_8-0) offer the highest compressive strength but very close to the mix with 5% CNCS and 8% cement (CEB_CNCS_8-5). By increasing the aggregate proportions, the compressive strength decreases in both wet and dry samples whatever the compacted pressure used.
Figure 16. Compressive strengths of the mixes at different compaction pressures value and dry (D) and wet (W) samples.

These results are strongly influenced by the density of the samples and highly hydrophilic characteristics of CNCS aggregates.
The results remain relatively close to those of Guettala et al. (Guettala et al., Citation2016) who worked on mixes of earth, sand dune and cork aggregates at various compaction pressures (from 2.5 MPa to 10 MPa).
Taking into account the requirements defined in ASTM E2392M-10 (2 MPa for dry compressive strength and 1 MPa for wet compressive strength; ASTM E2392M-10, Citation2016), bricks compacted at 2.5 MPa will be used for further studies. The compaction pressure should be used as low as possible to facilitate manual compaction at a Terstaram manual press for construction projects, but also to obtain a less dense and more porous CEB that would guarantee better thermal performances.
Figures show the values of the flexural modulus of rupture (MoR) and the modulus of elasticity (MoE) as a function of compaction pressure in the dry state. In general, increasing the compaction pressure improves both the flexural and compressive MoE and the flexural MoR as well as compressive MoR.
The CEB_CNCS_8-0 without CNCS-aggregate presents the highest flexural and compressive MoR (1.52–8.56 MPa) and MoE (24.5–90.81 MPa for flexural test and 178.6–225.03 MPa for compression test) at any compaction pressure. In general, the addition of aggregates leads to a decrease of the MoR and MoE, and the best performance was obtained with 5% aggregate.
Compressive test results (90.81 MPa) are relatively lower than those found in the work of Mostafa and Uddin (Mostafa & Uddin, Citation2016) indicating a maximum MoE value of 175 MPa for Banana fiber reinforced CEB and stabilised at 7% of Ordinary Portland Cement.
By increasing to 10% the proportion of CNCS aggregates, a decrease of about 35%, 22%, 15%, and 11% of MoE in flexural test, respectively, to 2.5, 5, 7, and 10 MPa of compaction, is observed. In compression, the MoE is varying of about 34%, 24%, 9% and 5%, respectively, at 2.5, 5, 7, and 10 MPa compaction. At the same value of compaction, for a variation of about 10% of CNCS aggregates, there is a decrease of about 40%, 66%, 38%, and 31% at flexural MoR. A real impact with the use of cement is to be noted.
The flexural MoR (0.79 MPa) of the CEB_CNCS_8-5 is lower than the results for plant fibre reinforced CEB. Kolawole et al. (Kolawole et al., Citation2017) obtained a strength range between 0.9 and 2.25 MPa for cement-stabilized and bamboo fiber-reinforced CEB. Similarly, Tido et al. (Stanislas et al., Citation2021), with cement-stabilized CEB and cellulose nano-fibre and paper cellulose, obtained MoR between 2.04 and 2.91 MPa.
The decrease in MoR with the percentage of CNCS can be attributed to the non-cohesive of the large CNCS particles in the stabilised earth matrix. Porosities (Figure ) around the large aggregates are observed by a binocular goniometer Stemi 508. These porosities and the lack of adhesion are inducing a less efficient behaviour of the composite material.
3.2. Thermophysical properties
Figure shows that the thermal parameters logically vary according to the density of the brick. By increasing the percentage of CNCS aggregates in the bricks, the density of the brick decreases, due to the low density of the aggregates (1.30 g/cm3) compared to that of the earth (2.76 g/cm3). In general, the bricks hereabove designed are highly insulating due to their low thermal conductivity. This will be important for environments with strong thermal variation such as the coastal region in Cameroon where the earth is extracted. Thus, lightweight constructions are projected with advanced thermal comfort. Several studies have given similar conductivity results for biosourced CEB (Guettala et al., Citation2016; Nshimiyimana et al., Citation2020).
3.3. Durability
Figure shows the linear correlations between capillary absorption and the square root of time during 1 hour for CEB_CNCS_8-0, CEB_CNCS_8-5, CEB_CNCS_8-10, CEB_CNCS_8-15. The slopes were used to determine the sorptivity. This coefficient provides a qualitative assessment of the absorption rate in the capillary pores. It depends on the width of the pore (Cassagnabère et al., Citation2011).
The results show that the sorptivity is 0.046, 0.019, 0.019 and 0.02 g/cm2·min0.5, respectively, for CEB_CNCS_8-15, CEB_CNCS_8-10, CEB_CNCS_8-5 and CEB_CNCS_8-0. The addition of CNCS aggregates causes the increase in sorptivity. This makes sense because CNCS aggregates are hydrophilic and cause the presence of large porosities in the brick (Figure ).
The highest capillary absorption value is 9 g·cm−2·min0.5 for CEB_CNCS_8-15 (Figure .a). After 20 minutes, a stabilization trend is observed for all samples. These values allow to classify these CEB as weakly capillary bricks with a capillarity coefficient lower than 20% (XP P13-901, Citation2001).
The results of total water absorption (Figure .b) is obtained within 24 hours about 20.32%, 17.99%, 16.46% and 15.90%, respectively, for the mixtures CEB_CNCS_8-15, CEB_CNCS_8-10, CEB_CNCS_8-5 and CEB_CNCS_8-0. The addition of CNCS aggregates overall induces an increase in the TWA of biosourced-CEB. This is due to the increase in porosity and also to the hydrophilic nature of the CNCS aggregates. The work of Abessolo (Citation2020) also showed the increase of TWA when the fiber content of Bambusa vulgaris was increased (0.5–1%) in CEB based on lateritic soil (Abessolo et al., Citation2020). Similarly, Nshimiyimana (Citation2020) found that water absorption, for earth bricks stabilized with 10% calcium carbide residue and containing (0–1.2%) Okra fibers, increased in the range of 17–21% after 24 H (Nshimiyimana, Citation2020).
Unstabilized bricks (CEB_CNCS_0-0) showed no durability during water absorption. They were completely destroyed (Figure ) both in the water absorption by capillarity and by total immersion.
The coefficients of abrasion for CEB_CNCS_0-0, CEB_CNCS_8-0, CEB_CNCS_8-5, CEB_CNCS_8-10 and CEB_CNCS_8-15 are 9.6, 26, 21.6; 10.7, 8.9 cm2/g, respectively. They respect the criteria of durability—above 2 cm2/g—defined by the standard XP P13-901 (XP P13-901, Citation2001).
The most sensitive composites to abrasion were the unstabilized and the stabilized CEB with 15% of CNCS aggregates, with abrasion coefficients around 9 cm2/g (Figure ). The addition of bio-aggregates reduces the abrasion resistance. The addition of 8% cement improved the strength of the brick threefold compared to the unstabilized one, in contrast to the addition of the biobased aggregates which caused a decrease of 16%, 58% and 65% for biobased additions of 5%, 10% and 15%, respectively.
Previous works (Izemmouren et al., Citation2015) on steam-cured bricks, stabilized with pozzolan-lime and compacted to 5 MPa, showed that the abrasion coefficient also increased (5.6–15 cm2/g) with the stabilizer content (0–10%) for 10% natural pozzolan. These values are relatively lower than found in this study for simply stabilized bricks. The nature of soil and stabilizer are clearly responsible for different results.
Finally, hygrometric and thermal conditions influence the mechanical characteristics of CEBs. For a fixed ambient temperature (Figure ), the increase of the humidity induces a quasi-linear reduction in compressive strength. Similarly, by setting a humidity value and increasing the ambient temperature, the compressive strength is reduced by about 42% between 50°C and 90°C. Unfortunately, in the range of 90°C, the value of the compressive strength is less than 2 MPa, which means that temperature ranges above 70°C are clearly detrimental.
A high sensitivity to temperature is therefore observed. This may be due to the use of CNCS, which are considered as engineering polymers in Ashby classification (Ganou Koungang, n.d.apeu, Tchuindjang et al., Citation2020), when approaching 90°C, there is a loss of about 7 wt % of the CN and CS materials due to the release of the physiosorbed water constituting the material. This temperature is also the starting temperature for the destruction of cellulose (Thakur et al., Citation2020) and therefore the glass transition is probably reached (Campo, Citation2008).
Subsequently, thermal conductivity increases with the relative humidity of the environment and decreases with increasing temperature. In fact, the presence of water in the material causes an almost linear increase in thermal conductivity (Mvondo Ngono et al., Citation2017). Moreover, by increasing the temperature of the environment, the molecules that are the components of the brick become excited and expand, which promotes the heat diffusion in the brick and thus causes an increase in the thermal conductivity (Noumowé et al., Citation2009).
3.4. Prediction and sensibility result
By collecting the characterisation data of the biobased aggregate earth bricks, the analysis of the impact of the main input parameters listed in Table on the mechanical strength allowed to appreciate three artificial intelligence models by comparing the sensitivity criterion being the regression coefficient R2.
Table 5. Experimental input datasets for compressive results
These results (Figure ) demonstrate that the modelling and validation tests for compressive strength were successful. The NN and MLR models showed the best R2 (~89% and ~85%, respectively), while the SVM model showed a correlation of 35%. It can be seen that the use of NN models best shows the sensitivity of the mechanical strength to the input factors considered. Subsequently, for the prediction of the Young’s modulus, the NN and MLR always presented the best R2 (~99% and ~96%, respectively), and the SVM model remains not very accurate (~66%) for this dataset. Previous research on the prediction of the behaviour of stabilised mud bricks supports the use of SVM for the prediction of compressive strength (Mahamat et al., Citation2021). They obtained better correlations with SVM compared to NN and LR for alkali-activated clay brick works.
On the other hand, the MLR model has already been converged in works such as those of Chou et al. (Citation2010). These have optimised the prediction of the compressive strength of concrete which is very sensitive to the amount of effective water, additive materials such as fly ash, blast furnace slag, superplasticizers, age and coarse and fine aggregates. This is consistent in some respects with the input parameters of the present study: proportion of water, bio-aggregate size, proportion of cement.
However, the comparison of studies in this field is governed by a large number of versatilities: the performance of the computer used, the version and program used, the numerical configurations of the models used, the nature, heterogeneity and weight of the input parameters on the response parameter.
Sensitivity as a “what if analysis” is an important issue in nonlinear geotechnical problems, because the given results only uncover the samples and won’t determine what variables have the most influence. Extraneous variables might interfere with the information and thus outcomes can be adversely impacted by the quality of the work (Afan et al., Citation2014; Asheghi et al., Citation2020; Pichery, Citation2014). However, the sensitivity analysis is beyond the scope of this work.
4. Conclusion
The objective of this study was to investigate the physical, mechanical and durability properties of compressed earth blocks (CEBs) stabilized with cement and reinforced with CNCS aggregates. The experimental program provides key information on the effect of the CNCS aggregate on the CEB composites. The following conclusions can be reached from the present investigations:
Samples without CNCS (CEB_CNCS_8-0) offer the highest compressive strength but close to the mix made with 5% CNCS and 8% cement (CEB_CNCS_8-5) in mass. The increase of biosourced aggregate content reduces the compressive strength in both wet and dry samples whatever the compaction pressure used;
The thermal conductivity is decreasing with increasing percentage of CNCS aggregates. The hereabove produced bricks can be considered as highly insulating due to their thermal conductivity lower than 1 W·m−1·K−1;
Saturation is obtained within 24 hours and the highest value of water absorption was about 20.30% for the CEB_CNCS_8-15;
For a fixed ambient temperature, the increase in humidity induces a quasi-linear reduction in compression strength.
The investigations performed in terms of mechanical and thermal performances, as well as durability tests, show that CEB stabilized with 8% of cement CEM II-B-LL 42.5 and 5% CNCS clearly fulfil requirements. The addition of 5% CNCS aggregates can improve the thermophysical properties of CEBs and achieve the required mechanical properties for CEB composites to be used as wall elements for multi-storey buildings. Durability is a point of attention, in order to guarantee long-term behaviour of buildings with CEB_CNCS_8-5, specific architectural technical details should be adapted to limit the contact with water.
For the machine learning prediction, the correlation between the input parameters and the output (mechanical compressive strength and compressive young’s modulus) displayed by the coefficient of determination R2 indicates that the NN (89% and 99% for MoR and MoE respectively) and MLR (85% and 96% for MoR and MoE respectively) models are suitable for predicting the features of this biosourced compressed earth brick dataset with cement as stabilizer.
For a more accurate understanding of these prediction models, it will be wise to extend the inputs and also consider the sustainability features of the bricks.
Acknowledgements
The authors would like to thank the Académie de Recherche et d’Enseignement Supérieur (ARES) of the Government of Wallonia-Brussels Federation (Belgium) for financial support through the exceptional doctoral fellowships program.
Disclosure statement
No potential conflict of interest was reported by the author(s).
Additional information
Funding
Notes on contributors
Ganou Koungang B.M.
Bernard Morino Ganou Koungang is a PhD holder of the Department of Architecture, Geology, Environmental and Construction, in the University of Liege in Belgium and Mechanical Engineering in the University of Douala in Cameroon. His key research activities include eco-friendly construction, effective waste management and recycling, development of innovative materials for the construction. He is specially focused on secondary raw materials for sustainable construction. He is committed to offering sustainable construction solutions with low carbon impact and energy savings.
References
- Abbaszadeh Shahri, A., Larsson, S., & Johansson, F. (2016). Updated relations for the uniaxial compressive strength of marlstones based on P-wave velocity and point load index test. Innovative Infrastructure Solutions, 1(1), 1–30.
- Abessolo, D., Biwole, A. B., & Fokwa, D. (2020). Effets de la longueur et de la teneur des fibres de bambou sur les propriétés physicomécaniques et hygroscopiques des blocs de terre comprimée utilisés dans la construction. Afrique Science, 16(3), 161–171. http://www.afriquescience.net/PDF/16/4/2.pdf
- Adam, E. A., & Agib, A. R. A. (2017). Compressed stabilised earth block manufacture in Sudan. UNESCO.
- Afan, H. A., El-Shafie, A., Yaseen, Z. M., Hameed, M. M., Wan Mohtar, W. H. M., & Hussain, A. (2014). ANN based sediment prediction model utilizing different input scenarios. Water Resources Management, 2014 29:4. 29(4), 1231–1245. https://doi.org/10.1007/s11269-014-0870-1.
- Ahn, Y. H., Pearce, A. R., Wang, Y., & Wang, G. (2013). Drivers and barriers of sustainable design and construction: The perception of green building experience. International Journal of Sustainable Building Technology and Urban Development, 4(1), 35–45. https://doi.org/10.1080/2093761X.2012.759887
- Akadiri, O. P. (2011). Development of a multi-criteria approach for the selection of sustainable materials for building projects. university of Wolverhampton.
- Akadiri, O. P., & Olomolaiye, P. O. (2012). Development of sustainable assessment criteria for building materials selection. Engineering, Construction and Architectural Management, 19(6), 666–687. https://doi.org/10.1108/09699981211277568
- Algin, H. M., & Turgut, P. (2008). Cotton and limestone powder wastes as brick material. Construction and Building Materials, 22(6), 1074–1080. https://doi.org/10.1016/j.conbuildmat.2007.03.006
- Al Rim, K., Ledhem, A., Douzane, O., Dheilly, R. M., & Queneudec, M. (1999). Influence of the proportion of wood on the thermal and mechanical performances of clay-cement-wood composites. Cement and Concrete Composites, 21(4), 269–276. https://doi.org/10.1016/S0958-9465(99)00008-6
- Asheghi, R., Abbaszadeh Shahri, A., & Khorsand Zak, M. (2019). Prediction of Uniaxial Compressive Strength of Different Quarried Rocks Using Metaheuristic Algorithm. Arabian Journal for Science and Engineering, 44(10), 8645–8659.
- Asheghi, R., Hosseini, S. A., Saneie, M., & Shahri, A. A. (2020). Updating the neural network sediment load models using different sensitivity analysis methods: A regional application. Journal of Hydroinformatics, 22(3), 562–577. https://doi.org/10.2166/hydro.2020.098
- ASTM E2392M-10. (2016). Standard guide for design of earthen wall building systems. ASTM, 04(12), 1–10. https://doi.org/10.1520/E2392_E2392M-10R16
- Ben Chaabene, W., Flah, M., & Nehdi, M. L. (2020). Machine learning prediction of mechanical properties of concrete: Critical review. Construction and Building Materials, 260, 119889.
- Bruno, A. W., Gallipoli, D., Perlot-Bascoules, C., Mendes, J., & Salmon, N. (2015). Briques de terre crue : Procédure de compactage haute pression et influence sur les propriétés mécaniques. 33èmes Rencontres de l’AUGC, ISABTP/UPP, Anglet, 1–9. https://hal.archives-ouvertes.fr/hal-01167676
- Bunz, K. R., Henze, G. P., & Tiller, D. K. (2006). Survey of sustainable building design practices in North America, Europe, and Asia. Journal of Architectural Engineering, 12(1), 33–62. https://doi.org/10.1061/(ASCE)1076-0431(2006)12:1(33)
- Campo, E. A. (2008). Thermal properties of polymeric materials. In W. Andrew (Ed.), Selection of Polymeric Materials (pp. 103–140). Elsevier.
- Cassagnabère, F., Lachemi, M., Mouret, M., & Escadeillas, G. (2011). Caractérisation performantielle d’un liant ternaire à base de ciment, laitier et métakaolin. Canadian Journal of Civil Engineering, 38(8), 837–848. https://doi.org/10.1139/l11-043
- Chithra, S., Kumar, S. R. R. S., Chinnaraju, K., & Alfin Ashmita, F. (2016). A comparative study on the compressive strength prediction models for High Performance Concrete containing nano silica and copper slag using regression analysis and Artificial Neural Networks. Construction and Building Materials, 114, 528–535.
- Chou, J.-S., Chiu, C.-K., Farfoura, M., & Al-Taharwa, I. (2010). Optimizing the prediction accuracy of concrete compressive strength based on a comparison of data-mining techniques. Journal of Computing in Civil Engineering, 25(3), 242–253. https://doi.org/10.1061/(ASCE)CP.1943-5487.0000088
- Cid-Falceto, J., Mazarrón, F. R., & Cañas, I. (2012). Assessment of compressed earth blocks made in Spain: International durability tests. Construction and Building Materials, 37, 738–745. https://doi.org/10.1016/j.conbuildmat.2012.08.019
- CIRAD., 2006. Annual report CIRAD 2006: Growing crops in town a response to urbanization.
- CRAterre, Houben, H., & Guillaud, H. (2006). Traité de construction en terre (3rd) ed.). Éd. Parenthèses.
- D1632 - 07. (2007). Standard practice for making and curing soil-cement compression and flexure test specimens in the laboratory (Withdrawn 2016). ASTM International, 1–15. https://doi.org/10.1520/D1632-07
- D5334 - 00. (2000). Standard test method for determination of thermal conductivity of soil and soft rock by thermal needle probe procedure. ASTM International.
- Ferentinou, M., & Fakir, M. (2017). An ANN approach for the prediction of uniaxial compressive strength of some sedimentary and igneous rocks in eastern KwaZulu-Natal. Proc Eng, 191, 1117–1125.
- Fontaine, L., & Anger, R. (2010). Bâtir en terre, du grain de sable à l’architecture. Belin/Cité des sciences et de l’industrie.
- Gallipoli, D., Bruno, A. W., Perlot, C., & Mendes, J. (2017). A geotechnical perspective of raw earth building. Acta Geotechnica, 12(3), 463–478. https://doi.org/10.1007/s11440-016-0521-1
- Ganou Koungang, B. M., Ndapeu, D., Tchemou, G., Messan, A., Njeugna, E., & Courard, L. (2020). Challenge to enhance the value of the Cameroonian coastal earth : Physical tests and mechanical characterization of earth material. SN Applied Sciences, 2(July), 11. https://doi.org/10.1007/s42452-020-3141-1
- Ganou Koungang, B. M., Ndapeu, D., Tchemou, G., Njeugna, E., & Courard, L. (2019a). Formulation des briques de terre biosourcées a charges de granulats de Canarium schweinfurthii et Cocos nucifera. In M. A. Etoh & G. Tchiete (Eds.), 4ème Conférence scientifique des Doctorants et Jeunes chercheurs des Universités d’Etat/ Instituts privés l’Enseignement supérieur au Cameroun de Douala (pp. 15). Université de Douala.
- Ganou Koungang, B. M., Ndapeu, D., Tchemou, G., Njeugna, E., & Courard, L. (2019b). Comportement hydromécanique des BTC avec granulats de Canarium schweinfurthii et Cocos nucifera: Analyse de durabilité. In Colloque international des 4Oaires de l’Enset de Douala (Enset). Raiffet:. 10.
- Ganou Koungang, B. M., Ndapeu, D., Tchuindjang, J. T., Wenga Ntcheping, B., Tchemou, G., Bistac, S., Njeugna, E., & Courard, L. (2020). Influence of temperature on the creep behaviour by macroindentation of Cocos nucifera shells and Canarium schweinfurthii cores (bio-shellnut wastes in Cameroon). Materials Research Express, 7(10), 1–14. https://doi.org/10.1088/2053-1591/abbebb
- Giroudon, M., Laborel-Préneron, A., Aubert, J. E., & Magniont, C. (2019). Comparison of barley and lavender straws as bioaggregates in earth bricks. Construction and Building Materials, 202, 254–265. https://doi.org/10.1016/j.conbuildmat.2018.12.126
- Guettala, S., Bachar, M., & Azzouz, L. (2016). Properties of the compressed-stabilized earth brick containing Cork granules. Journal of Earth Science and Climatic Change, 7(5). https://doi.org/10.4172/2157-7617.1000353
- Guillaud, H., Joffroy, T., Odul, P., & CRATerre-EAG, (1995). Compressed earth blocks- manual of design and construction: Volume II (2nd) Eschborn: Deutsches Zentrum für Entwicklungstechnologien - GATE in: Deutsche Gesellschaft für Technische Zusammenarbeit (GTZ) GmbH in coordination with BASIN. https://craterre.hypotheses.org/1457
- Häkkinen, T., & Belloni, K. (2011). Barriers and drivers for sustainable building. Building Research & Information, 39(3), 239–255. https://doi.org/10.1080/09613218.2011.561948
- Houben, H., Boubekeur, P., Doat, S., D’Ornano, A., Douline, P., Garnier, H., Guillaud, T., Joffroy, & Rigassi, V. (1998). Compressed Earth Blocks: Standards. CDI & CRAterre.
- Izemmourena, O., & Guettala, A. (2014). Amélioration de la durabilité des BTC à base d’un sol de la région de Biskra. MATEC Web of Confrences, 11, 1–5. https://www.matec-conferences.org/articles/matecconf/pdf/2014/02/matecconf_cmss2013_02001.pdf
- Izemmouren, O., Guettala, A., & Guettala, A. (2015). Mechanical properties and durability of lime and natural pozzolana stabilized steam-cured compressed earth block bricks. Geotechnical and Geological Engineering, 33(5), 1321–1333. https://doi.org/10.1007/s10706-015-9904-6
- Kahraman, S. (2001). Evaluation of simple methods for assessing the uniaxial compressive strength of rock. Int J Rock Mech Min Sci, 38(7), 981–994.
- Kolawole, F. O., Olugbemi, O. M., Kolawole, S. K., Owa, A. F., & Ajayi, E. S. (2017). Fracture toughness and strength of bamboo-fiber reinforced laterite as building block material. Universal Journal of Materials Science, 5(3), 64–72. https://doi.org/10.13189/ujms.2017.050302
- Laborel-Préneron, A., Aubert, J. E., Magniont, C., Tribout, C., & Bertron, A. (2016). Plant aggregates and fibers in earth construction materials: A review. Construction and Building Materials, 111, 719–734. https://doi.org/10.1016/j.conbuildmat.2016.02.119
- Le Roux, A. (1969). Contribution à l’étude du traitement à la chaux des matériaux argileux. Université des Sciences d’Orsay.
- Mahamat, A. A., Boukar, M. M., Ibrahim, N. M., Stanislas, T. T., Linda Bih, N., Obianyo, I. I., & Savastano, H. (2021). Machine learning approaches for prediction of the compressive strength of alkali activated termite mound soil. Applied Sciences (Switzerland), 11(11), 1–13. https://doi.org/10.3390/app11114754
- Masuka, S., Gwenzi, W., & Rukuni, T. (2018, January). Development, engineering properties and potential applications of unfired earth bricks reinforced by coal fly ash, lime and wood aggregates. Journal of Building Engineering, 18, 312–320. https://doi.org/10.1016/j.jobe.2018.03.010
- Meukam, P., 2004. Valorisation des briques de terre stabilisées en vue de l’isolation thermique des bâtiments. Thèse en co-tutelle entre l’Université de Cergy-Pontoise et l’Université de Yaoundé I. https://www.theses.fr/2004CERG0287
- Molina, B. E. S. (2016). Raw-earth housing in Antioquia, Colombia. Eco-Architecture VI: Harmonisation between Architecture and Nature, 161, 181. https://www.witpress.com/books/978-1-78466-111-3
- Mostafa, M. (2016). Sustainable construction with Green Compressed Earth Block (GCEB). The University of Alabama at Birmingham.
- Mostafa, M., & Uddin, N. (2016). Experimental analysis of Compressed Earth Block (CEB) with banana fibers resisting flexural and compression forces. Case Studies in Construction Materials, 5, 53–63. https://doi.org/10.1016/j.cscm.2016.07.001
- Moussa, H. S., Nshimiyimana, P., Hema, C., Zoungrana, O., Messan, A., & Courard, L. (2019). Comparative study of thermal comfort induced from Masonry made of stabilized compressed earth block vs conventional cementitious material. Journal of Minerals and Materials Characterization and Engineering, 07(6), 385–403. https://doi.org/10.4236/jmmce.2019.76026
- Muntohar, A. S. (2011). Engineering characteristics of the compressed-stabilized earth brick. Construction and Building Materials, 25(11), 4215–4220. https://doi.org/10.1016/j.conbuildmat.2011.04.061
- Mvondo Ngono, R. R., Meukam, P., Jeong, J., Meneses, D. D. S., & Nkeng, E. G. (2017). Influence of water content on the mechanical and chemical properties of tropical wood species. Results in Physics, 7, 2096–2103. https://doi.org/10.1016/j.rinp.2017.06.025
- Nagaraj, H. B., Sravan, M. V., Arun, T. G., & Jagadish, K. S. (2014). Role of lime with cement in long-term strength of Compressed Stabilized Earth Blocks. International Journal of Sustainable Built Environment, 3(1), 54–61. https://doi.org/10.1016/j.ijsbe.2014.03.001
- NF EN 1097-6. (2014). Essais pour déterminer les caractéristiques mécaniques et physiques des granulats - Partie 6 : Détermination de la masse volumique réelle et du coefficient d’absorption d’eau. Afnor. https://www.boutique.afnor.org/fr-fr/norme/nf-en-10976/essais-pour-determiner-les-caracteristiques-mecaniques-et-physiques-des-gra/fa192181/321395
- NF EN 196-1. (2016). Méthodes d’essais des ciments - Partie 1 : Détermination des résistances. AFNOR, 1–22. https://www.boutique.afnor.org/fr-fr/norme/nf-en-1961/methodes-dessais-des-ciments-partie-1-determination-des-resistances/fa184622/57803
- NF P18-574. (1990). Granulats - Essai de fragmentation dynamique. AFNOR. https://www.boutique.afnor.org/fr-fr/norme/p18574/granulats-essai-de-fragmentation-dynamique/fa021246/56257
- NF P94-050. (1995). Sols : Reconnaissance et essais - Détermination de la teneur en eau pondérale des matériaux - Méthode par étuvage. AFNOR, 1–7. https://www.boutique.afnor.org/fr-fr/norme/nf-p94050/sols-reconnaissance-et-essais-determination-de-la-teneur-en-eau-ponderale-d/fa038799/11081
- NF P94-093. (2014). Sols : Reconnaissance et essais - Détermination des références de compactage d’un matériau - Essai Proctor Normal - Essai Proctor modifié. AFNOR, 1–22. https://www.boutique.afnor.org/fr-fr/norme/nf-p94093/sols-reconnaissance-et-essais-determination-des-references-de-compactage-du/fa185491/43924#:~:text=Norme%20Annul%C3%A9e-,Sols%20%3A%20reconnaissance%20et%20essais%20%2D%20D%C3%A9termination%20des%20r%C3%A9f%C3%A9rences%20de%20compactage%20d,normal%20et%20optimum%20Proctor%20modifi%C3%A9
- NF X15-119. (1999). Mesure de l’humidité de l’air - Générateurs d’air humide à solutions salines pour l’étalonnage des hygromètres. AFNOR. https://www.boutique.afnor.org/fr-fr/norme/nf-x15119/mesure-de-lhumidite-de-lair-generateurs-dair-humide-a-solutions-salines-pou/fa017368/16406
- Ngohe-Ekam, P. S., Meukam, P., Menguy, G., & Girard, P. (2006). Thermophysical characterisation of tropical wood used as building materials : With respect to the basal density Thermophysical characterisation of tropical wood used as building materials : With respect to the basal density. Construction and Building Materials, 20(9), 929–938. https://doi.org/10.1016/j.conbuildmat.2005.06.017
- Nitcheu, M., Meukam, P., Damfeu, J. C., & Njomo, D. (2018). Thermomechanical characterisation of compressed clay bricks reinforced by thatch fibres for the optimal use in building. Materials Sciences and Applications, 9(12), 913–935. https://doi.org/10.4236/msa.2019.912066
- Noumowé, A., Siddique, R., & Ranc, G. (2009). Thermo-mechanical characteristics of concrete at elevated temperatures up to 310 °C. Nuclear Engineering and Design, 239(3), 470–476. https://doi.org/10.1016/j.nucengdes.2008.11.020
- Nshimiyimana, P. (2020). Effect of the type clay earthen materials and distribution materials on the physico-mechanical properties and durability of compressed earth blocks. University of Liege and Institut 2iE.
- Nshimiyimana, P., Messan, A., & Courard, L. (2020). Physico-mechanical and hygro-thermal properties of compressed earth blocks stabilized with industrial and agro by-product binders. Materials, 13(17), 1–17. https://doi.org/10.3390/ma13173769
- Nshimiyimana, P., Messan, A., Zhao, Z., & Courard, L. (2019). Chemico-microstructural changes in earthen building materials containing calcium carbide residue and rice husk ash. Construction and Building Materials, 216, 622–631. https://doi.org/10.1016/j.conbuildmat.2019.05.037
- Nshimiyimana, P., Miraucourt, D., Messan, A., & Courard, L. (2018). Calcium Carbide Residue and Rice Husk Ash for improving the Compressive Strength of Compressed Earth Blocks. MRS Advances, 3(34–35), 2009–2014. https://doi.org/10.1557/adv.2018.147
- Obianyo, I. I., Mahamat, A. A., Anosike-Francis, E. N., Stanislas, T. T., Geng, Y., Onyelowe, K. C., Odusanya, S., Onwualu, A. P., & Soboyejo, A. B. O. (2021). Performance of lateritic soil stabilized with combination of bone and palm bunch ash for sustainable building applications. Cogent Engineering, 8(1), 1–19. https://doi.org/10.1080/23311916.2021.1921673
- Omer, A. M. (2008). Energy, environment and sustainable development. Renewable and Sustainable Energy Reviews, 12(9), 2265–2300. https://doi.org/10.1016/j.rser.2007.05.001
- Pichery, C. (2014). Sensitivity Analysis. Encyclopedia of Toxicology: Third Edition, 236–237. https://hal.ehesp.fr/hal-03708480
- Reeves, G. M., Sims, I., & Cripps, J. C. (2006). Clay materials used in construction, Engineering geology special publication. Geological Society of London.
- Saidi, M., Cherif, A. S., Zeghmati, B., & Sediki, E. (2018). Stabilization effects on the thermal conductivity and sorption behavior of earth bricks. Construction and Building Materials, 167, 566–577. https://doi.org/10.1016/j.conbuildmat.2018.02.063
- Sitton, J. D., Zeinali, Y., & Story, B. A. (2017). Rapid soil classification using artificial neural networks for use in constructing compressed earth blocks. Construction and Building Materials, 138, 214–221.
- Smith, J. B., Schneider, S. H., Oppenheimer, M., Yohe, G. W., Hare, W., Mastrandrea, M. D., Patwardhan, A., Burton, I., Corfee-Morlot, J., & Magadza, C. H. D., 2009. Assessing dangerous climate change through an update of the Intergovernmental Panel on Climate Change (IPCC)“reasons for concern”. Proceedings of the national Academy of Sciences, 106 (11), 4133–4137.
- Sore, S. O., Messan, A., Prud’Homme, E., Escadeillas, G., & Tsobnang, F. (2018). Stabilization of compressed earth blocks (CEBs) by geopolymer binder based on local materials from Burkina Faso. Construction and Building Materials, 165, 333–345. https://doi.org/10.1016/j.conbuildmat.2018.01.051
- Stanislas, T. T., Tendo, J. F., Teixeira, R. S., Ojo, E. B., Komadja, G. C., Kadivar, M., & Junior, H. S. (2021). Effect of cellulose pulp fibres on the physical, mechanical, and thermal performance of extruded earth-based materials. Journal of Building Engineering, 39, 102259. https://doi.org/10.1016/j.jobe.2021.102259
- Taallah, B., & Guettala, A. (2016). The mechanical and physical properties of compressed earth block stabilized with lime and filled with untreated and alkali-treated date palm fibers. Construction and Building Materials, 104, 52–62. https://doi.org/10.1016/j.conbuildmat.2015.12.007
- Taallah, B., Guettala, A., Guettala, S., & Kriker, A. (2014). Mechanical properties and hygroscopicity behavior of compressed earth block filled by date palm fibers. Construction and Building Materials, 59, 161–168. https://doi.org/10.1016/j.conbuildmat.2014.02.058
- Thakur, M., Sharma, A., Ahlawat, V., Bhattacharya, M., & Goswami, S. (2020). Process optimization for the production of cellulose nanocrystals from rice straw derived α-cellulose. Materials Science for Energy Technologies, 3, 328–334. https://doi.org/10.1016/j.mset.2019.12.005
- XP P13-901. (2001). Blocs de terre comprimée pour murs et cloisons, Définitions-Spécifications-Méthodes d’essais-Conditions de réception. AFNOR, 1–37. https://www.boutique.afnor.org/fr-fr/norme/xp-p13901/blocs-de-terre-comprimee-pour-murs-et-cloisons-definitions-specifications-m/fa120503/487
- Yasar, E., & Erdogan, Y. (2004). Correlating sound velocity with the density, compressive strength and Young’s modulus of carbonate rocks. Int J Rock Mech Min Sci, 41(5), 871–875.