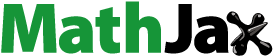
Abstract
This paper has focused on the study of flow characteristics of a steady conductive fluid through a circular pipe in the presence of an applied transverse uniform magnetic field. A numerical simulation of the fluid flow was performed using a multi-physics computational tool. The radial velocity distribution due to the effect of the tangential component of induced magnetic flux density and electric fields at various axial positions is examined in metallic and non-metallic surfaces of the circular pipe. This simulation study helps to analyse the effect of drag force on the variation in axial velocity close to the metallic and non-metallic walls. The induced electric potential is directed along the normal to the Laplacian tangential surface of the pipe. In addition to that, sigmoidal-shaped field profiles are observed when the fluid flow crosses from the upstream to downstream of the magnet edge and vice versa along the axial length of the circular pipe. Simultaneously, it is observed that the average velocity of the fluid flow decreases for a laminar and increases for turbulent Reynolds number. Streamline plots pertinent flow variable such as flow velocity are presented for non-conducting and conducting walls.
1. Introduction
The study of Newtonian fluids is important because of the crucial role they play in manufacturing processes, cutting-edge technologies, and established industries. Paper manufacturing, polymer processing, plastic film drawing, fluidization in a reactor, annealing and tinning copper wires, and metal spinning are just a few examples of the many applications of the Newtonian fluids. The effect of a magnetic field on the flow characteristics of an electrically conducting fluid through a channel is required to explore from a mathematical and engineering perspective due to its widespread applications in science and technology such as measurement of flow velocity, nuclear reactors, and blood measurements. Researchers are concentrated on the influence of magnetic field on the electrically conducting fluids flowing through conducting or non-conducing walls of the circular pipe. The flow characteristics of steady fully developed fluid through a pipe in the presence of a magnetic field have been addressed in Shercliff, (Citation1953, Citation1956). The characteristics of the induced electrical potential based on the velocity distribution of the conductive multiphase flows using a numerical simulation model are discussed in Wang et al., (Citation2006). In the study reported in Wang et al., (Citation2006), multiple virtual electrodes are arranged at various positions of the inner peripheral surface of the fluid pipe to obtain the induced potential drop. The induced potential difference across the electrodes increases with the fluid velocity and decreases sharply when the fluid flow is closer to the inner wall of the fluid pipe. For the reconstruction of the velocity profile within the pipe, the effect of different fluid particles located at different positions within the fluid pipe is to be carried out by analytical method or numerical simulation method (Wang et al., Citation2006). Malekzadeh et al. (Malekzadeh et al., Citation2011) carried out the investigations on MHD flows using various numerical schemes. Malekzadeh et al. concluded that the penetration of magnetic field on fluid flow velocity decreases by the reduction of angle between the flow direction and direction of an applied magnetic field. The pressure drop is proportional to the square of the product of the magnetic field and was reported by Fonseca et al., (Citation2021) Prasanna Jeyanthi & Ganesh, (Citation2022). When Hartmann number exceeds a critical limit (when magnetic field density is high), then the fully developed velocity profile asymptotically reaches saturation. Albets-Chico et al.,(Albets-Chico et al., Citation2011) studied the characteristics of high conductive fluid in non-conducting wall of the pipe due to the fringing magnetic field. The effect of fringing field on non-conducting wall of the pipe with high conductive fluid has been shown to transform the velocity profile to turbulent at the exit of the pipe. Jaroslav et al.,(Jaroslav, Citation2016) concentrated an analytical expression for mean flow velocity for unsteady flows in a straight cross-sectional pipe. It is evident that the new analytical expression with appropriate boundary conditions works for higher Reynolds number. Lorentz force and the pressure distribution within the core region of the fluid flow for various strengths of magnetic field for the liquid metal flow are explained in Li & Zikanov, (Citation2013). Thin and thick Hartmann layers of square and rectangular ducts (Dong et al., Citation2017; Hua & Walker, Citation1995; Pothérat et al., Citation2002; Tezer-Sezgin & Bozkaya, Citation2008) analyze the effect of strong transverse and inclined transverse magnetic field on the fluid flow. The stability of the fluid flow as examined by Hua & Walker, (Citation1995) for various Hartmann numbers. Pressure gradient is observed as a function of field is explained in (Murgatroyd, Citation1953). Ahmed et al., (Megahed et al., Citation2021) discussed the influence of the non-dimensional parameters such as magnetic parameter, viscosity and skin-coefficient on boundary layer of laminar velocity profiles under the presence of magnetic field along with heat transfer characteristics because of an uneven stretched sheet with an extensive heat flux. The increase in thickness of boundary layer with increase in viscosity results in reduction in velocity of the fluid flow. The skin-friction coefficient of the fluid flow increases due to the presence of a magnetic field. Modelling of the boundary layer equations transforms them into a system of Ordinary Differential Equations (ODEs). Their numerical solution using the Runge-Kutta integration method is discussed by Reddy et al., (Gnaneswara Reddy & Ferdows, Citation2021) for analyzing the thickness of the boundary layer. The magnetic field opposes the liquid transfer since an increase in magnetic parameter values tends to increase the Lorentz force, which opposes fluid movement and slows the movement of fluid. The magnetic field exerting a resistive force on the boundary layer fluid can control the boundary layer. As a result, the thickness of the thermal and solutal boundary layer decreases. The decrease in viscosity of the fluid flow increases the velocity of the fluid flow. Studies reported by Ramesh et al., (Citation2021), Reddy & Kumar, (Citation2021) discussed the influence of magnetic and electrical fields on pair stress nanofluids in microperistaltic channels subject to convective and slip boundary conditions. The behaviour of MagnetoHydroDynamics (MHD) on Newtonian/non-Newtonian fluids through a parallel plate in the presence of magnetic field numerically (Bhatti, Bég et al., Citation2022; Bhatti, Doranehgard et al., Citation2022) is discussed. From all these studies, it is inferred that study of conductive fluids within metallic and non-metallic circular pipe under the transverse magnetic field has not been addressed. In addition, mutual interaction of fluid flow with the fields is required to be explored at various locations of transverse and tilted axes.
In this paper, the variation of axial velocity along the different radial positions is analyzed with a tangential component of induced fields within metallic and non-metallic wall of the circular pipe. Mutual interaction of conductive fluid flow and magnetic field helps to realize the generation of electric field (E-field) on metal and non-metallic surfaces of circular pipe. This paper also focuses on the investigation of the retention of laminar and turbulent velocity profiles after the conductive fluid passes through the source of excitation. The rest of the paper is organized as follows. Section 1 discusses three-dimensional simulation model of circular pipe with magnetic system and its analysis. Section 2 examines the velocity profiles at various locations of the fluid flow in the axial direction, the effect of tangential component of induced magnetic, and electric fields on velocity of fluid flow along the radial direction with low conductive and followed by the concluding remarks.
2. Simulation model
Modeling MHD phenomenon necessitates taking into consideration the interactions between fluid flow, current flow, and magnetic fields, making it a multiphysics challenge by its very nature. The governing equations of MHD are represented through Partial Differential Equations (PDEs) and the Finite Element (FE) method can be utilised to find solutions to these equations. Consider an incompressible conductive fluid through a circular pipe along the z direction with the -directed transverse uniform magnetization field, as shown in Figure . From the schematic view, the
penetrates to the wall of a circular a pipe and interacts with the conductive fluid results to induces the magnetic and electric fields in the fluid flow direction. The strength of the magnetization field is computed for the magnet based on the excitation current (ABB, Citation2020). The magnetization field values for various excitation currents are tabulated in Table .
Table 1. Magnetization field for various excitation currents
The characterization of the fluid flow involves fluid flow and electromagnetics physics. These physics are defined with a set of equations illustrated from Eqs. (1) to (10). The following assumptions are made for the investigation of flow characteristics:
The fluid motion is steady, fully developed, and incompressible
The pressure gradient along the cross-section of the pipe is constant
The fluid motion is in homogeneous magnetic field
The electric field, magnetic field, and velocity of a fluid flow can be obtained by solving the Maxwell’s equations in combination with the Navier-Stokes equation and the conservation of mass under specified boundary conditions. Laminar flow/low Reynolds turbulent model is used to describe the fluid dynamics. The flow physics defined by the Navier-Stokes equation is paired with the continuity equation for incompressible fluids as follows:
Where is density,
is velocity vector,
is pressure,
is volumetric force,
is dynamic viscosity of the fluid. The closed boundary conditions for fluid contained in circular pipe are defined as follows: Inlet as fully developed flow with average velocity, outlet as atmospheric pressure and, no-slip condition at the walls of circular pipe. To characterise the electric and magnetic fields inside the pipe, a system of partial differential equations is required. The set of Maxwell’s equations (from Eqs. (3) to (6)) is solved using the magnetic vector potential and scalar electric potential as dependent variables in the field interface. Magnetic and electric fields interfaces are used to compute magnetic field and current distributions. These variables help to analyze the interaction of fluid field with the dependent variables.
where is magnetic field strength (or) magnetic field intensity (H-field),
is magnetic flux density in T,
is magnetic vector potential,
is current density,
is the electric field (E-field), and
is electric potential respectively. The magnets generate a static magnetic field, so that fluid with conductivity that move across this field at a velocity experiences induced current as represented by Eq.(7). In Eq. (7) σ is electrical conductivity of fluid.
+ (
(7)
The induced current through a magnetic field results in a volumetric force which will propel the flow as given in EquationEq.(8)(8)
(8) .
EquationEq. (8)(8)
(8) represents the volumetric force (Lorentz force) applied along the
axis (which is perpendicular to the direction of fluid flow,
axis).
Where is normal unit vector. The entire developed design is bounded by air domain and the surfaces are magnetically insulated. Boundaries are magnetically and electrically insulated in Eqs. (9) and (10).
The governing equations for the steady flow of an incompressible fluid under the transverse magnetic field are analysed in multi-physics computational tool. A water is considered as conductive fluid in circular pipe having an outer diameter of 33.40 mm with thickness of 1.65 mm and a length of 301 mm. Magnets are placed at a distance of 0.3 mm from outer surface of either non-metallic or metallic circular pipes. For the simulation model, non-metallic material is Polytetrafluoroethylene (PTFE) and metallic is carbon steel (1010). Induced fields such as magnetic and electric fields in the direction of flow are analysed. The model has meshed, and the mesh size for the whole structure was defined using fine mode. The tetrahedral was employed. It is noticed that from the grid independence test, 1000 × 1000 evenly spaced grids are adequate to achieve a convergence of the simulation results with an accuracy of 10−4.
3. Results and discussions
An incompressible conductive fluid flow inside a metallic/insulating circular conduit at constant pressure is considered for the simulation studies. Additionally, there are two circular magnets positioned in the upper and lower halves of the circular conducted placed at the midsection of the conduit. With respect to the midpoint of the conduit along its length (along the -axis), there is symmetry pertaining to the positional locations of the two magnets. The applied uniform magnetic field is transverse to the direction of fluid flow within a circular pipe and the electrical conductivity of the fluid is low. Both the magnets and the current flow contribute to the formation of the magnetic field. In contrast, for the boundary conditions and material properties considered in this study, the magnetic field generated by the current flow is substantially lower than the magnetic field generated by the magnets. For the study, the electrical conductivity of the fluid is 2 mS/m. The field and velocity profiles are analysed on the cross-sectional
planes located at different distances along the axial length (directionPOSTED). A 3D view of circular pipe placed between the stationary parallel magnets is shown in Figure ). Applied magnetic field is directed along
direction and induced E-field is measured in
direction as shown in Figure ). The magnets are placed symmetrically with respect to mid-point of the pipe (along its axial position at
=150 mm) and is at a distance of 0.3 mm from outer diameter of pipe. Axial length of the magnet is 20 mm and it extends from
=140 mm to
=160 mm.
Figure 2. (a) 3D view of circular pipe with stationary coils and (b) Applied magnetic field in plane and induced E-field
plane in flow direction

A schematic view of the induced electric potential is shown in Figure for non-conducting and Figure for conducting wall of the circular pipe. The visualization of the electric potential distribution in plane at
=150 mm is explored through a plot shown in Figure ) and Figure ) respectively. Figures ) and Figure ) divulge the fact that the induced electric potential in
direction is dominant near to the source of excitation and weak at the core of the pipe. This is explicitly shown in the Figure ) at various radial positions of y along the axial length of
=150 mm (where the source of excitation).
Figure 3. Induced electric potential distribution in non-conducting wall of circular pipe (a) Slice plot of electric potential at cross-sectional area (b) Electric potential distribution in the axial direction at various radial position of
.

Figure 4. Induced electric potential distribution in conducting wall of circular pipe (a) Slice plot of electric potential at cross-sectional area (b) Electric potential distribution in the axial direction at various radial position of
.

Electric potential is induced on the surface of non-conducting wall at location of y= −16 mm and 16 mm. The electric potential is directed along the normal to the wall of pipe as shown in Figure ). Electrical potential on inner wall of the circular pipe is due to the current density along the inner wall of the pipe as shown in Figure ) at y = 0 mm. This electric potential is directed along the normal to the Laplacian tangential surface of the pipe.
The velocity profiles are examined for laminar and turbulent Reynolds numbers ( at various axial positions (in the direction of
). The se profiles are examined from entrance of the pipe to exit of the pipe on transverse sections of the pipe relative to flow direction. The fluid flow continues to experience the effect of applied magnetic field at cross sectional planes whose location is clearly outside the axial length of the magnet. The applied magnetic field that tends to influence the fluid flow is theoretically calculated using EquationEq. (11)
(11)
(11) .
where is the radius of the magnet (10 mm),
is the distance from the magnet (110 mm),
is the current (400 mA), and
is magnetic permeability of the magnet (
×10−7 H/m) and the computed magnetic flux density from EquationEq.11
(11)
(11) is 1.8650 ×10−8 T. The computed
is negligible effect at
=110 mm. Flow profile exhibits disturbance at
=110 mm onwards as shown in Figures . It takes a significant distance for the velocity profile to adapt from the fully developed to the MHD fully developed pattern under steady and uniform magnetic field. This analysis helps to understand the transition of flow pattern along the axial length of circular pipe.
Figure shows the laminar velocity profiles for Reynolds number of 2029.213 resembles to both non-conducting and conducting walls of circular pipe. The conductive fluid flow under the influence of magnetic field is examined along the - axis at various axial positions
. The results of Figure show that fully developed parabolic profile transforms to flat profile. The Lorentz force acts as resistive drag force is in
direction and it is directly proportional to the velocity of fluid. On the wall surface of the pipe, the resistive drag force is zero. This further increases the gradient of resistive drag force at the centre-line of fluid flow leading to the suppression of the velocity of axial flow along the centre stream of the pipe. The axial flow velocity near to wall of the pipe starts to increase to maintain the constant mass flow. Hence, under the influence of transverse magnetic field, the average velocity of the fluid decreases and the fluid velocity exhibits flat profile. Once the developed flow reaches the saturation, flow pattern is invariant with increase of the applied magnetic field. The flow profile remains same until the flow comes out of region of the magnetic field distribution.
Variation of axial velocity along the radial direction at different axial positions for non-conducting and conducting wall under the influence of magnetic field is shown in Figure . The flat profile of fully developed flow transforms to hyperbolic profile at = 110 mm onwards. The resistive drag force results in the development of the non-uniform force distribution between the walls of the pipe and middle of the fluid flow. The axial velocity on the walls of the pipe increases to maintain the uniform mass flow and develops the shear stress. The shear stress increases the skin friction factor of the fluid flow, which in turn increases the velocity of fluid flow along the center-line eventually resulting in its acceleration. Hence, the increase in average fluid flow velocity is clearly seen in Figure .
From the Figures , it is observed that the radial velocity distribution is not affected at axial positions of z = 0 mm, 10 mm and 60 mm. The fluid flow enters the region of magnetic field at z =110 mm and the maximum fluid flow disturbance is seen at =140 mm onwards (calculated from EquationEq.(11
(11)
(11) )), the transition of flow profile is observed till
= 260 mm.
In any xy plane throughout the axial length of circular pipe, the field and velocity profiles are observed at with variation in
covering the range of -D/2 to D/2 and y = 0 as shown in Figure , where D is diameter of the pipe. The solid and dotted lines in
cross-sectional plane show the transversal x-axis and tilted x-axis respectively along the axial length of pipe. The terminology of axial distances is depicted in Table .
Table 2. Terminology for various locations of axial length of circular pipe in cross sectional plane
Till now laminar and turbulent velocity profiles are analyzed at various axial length of circular pipe. The interaction of fluid flow with induced tangential components of magnetic flux density and E-fields is required to examine in the flow direction for both metallic and non-metallic (plastic) circular pipes. From EquationEq.(8
(8)
(8) ), the perpendicular nature of Lorentz force on the conductive fluid flow separates the positive and negative charged particles in
direction (which is perpendicular to the direction of applied magnetic field).The separation of charges establishes the electric field in both normal (
and tangential (
directions of fluid flow. The velocity profile is effected with both the induced tangential component of magnetic flux density,
and induced tangential component of E-field,
due to the resistive drag force.
The influence of tangential component of induced magnetic flux density, on the fluid flow is examined in Figure along the transverse x-axis and
tilted x-axis along axial length of pipe for both metallic and non-metallic circular pipe. The results are observed at the excitation of 400 mA with laminar
of 2029.213. The induced magnetic flux density
in non-conducting circular pipe is high since the penetration of magnetic field is more compared to the case of metal pipe. Velocity of fluid flow is decreased at
= 250 mm, as observed from the results of Figure ) and Figure ) respectively. The average velocity of the fluid flow decreases both in non-conducting and conducting ducts due to Lorentz force as represented in EquationEq.(8)
(8)
(8) .
Figure 8. Induced magnetic flux density on radial velocity of fluid flow in non-conducting and conducting duct at various axial position of
for Reynolds number of 2029.213.

The reduced penetration of magnetic flux density into the metal pipe is due to the small eddy currents developed on the surface of the pipe. The tangential component of induced magnetic flux density is high on the surface of the metal pipe compared to the plastic pipe. The tangential component of is more at
= 150 mm as compared to the other locations since the effect of tangential field is more as compared to the radial effect. Hence, the average velocity of fluid flow decreases as compared to the velocity at upstream and downstream of magnetic locations along both transverse
-axis and
tilted
-axis as shown in Figure ) and Figure ). Similarly, the impact of tangential component of an induced electric field,
on the radial velocity of fluid flow is observed at various axial positions of pipe as shown in Figure .
Figure 9. Induced E-field on radial velocity of fluid flow in non-conducting and conducting in duct at various axial position of
for Reynolds number of 2029.213.

The effect of on velocity of fluid is similar that due to
at location of
= 250 mm in plastic and metal pipe as seen in Figure ) and Figure ). As shown in the results of Figures ), the disturbance of fluid flow is seen in along both transverse x-axis and
tilted
-axis at z =150 mm. The
accelerates the fluid flow in plastic as compared to metal pipes. The effect of the tangential component of induced magnetic flux density,
and induced E-field,
on axial velocity is continued for turbulent flows in both metallic and non-metallic circular pipes. The tangential components of induced magnetic flux density,
and induced E-field,
at a distance of
=150 mm on metal and plastic surfaces of circular pipe with an excitation of 400 mA at a Reynolds number of 2029.213 are shown in Figures respectively.
Figure 10. Radial Induced magnetic flux density, distribution in non-conducting and conducting in duct at various axial positions of
, when
= 2029.213, and I = 400 mA.

Figure 11. Radial Induced E-field distribution in non-conducting and conducting in duct at various axial positions of
, when
= 2029.213, and I = 400 mA.

Figure ), at the transverse -axis, the magnetic flux density,
is observed to be positive near the pipe wall upstream of the magnet edge (
=50 mm) due to the magnetic flux lines’ convergence in the fluid flow direction. Hence the
is inclined from positive to negative within the inner wall of circular pipe. This accelerates the fluid flow. At the downstream of the magnet edge, z = 250 mm, the direction of the magnetic flux density,
, reverses with respect to that at the location of z = 50 mm due to the convergence of flux lines opposite to the direction of fluid flow. Maximum value of
is observed on the surface of metallic circular pipe due to induced circulating currents on it. The magnetic flux density,
gradually reduces to minimum value inside the circular pipe due to the generated eddy currents opposing the fluid flow. This continues for various locations of
along 45° tilted
-axis as shown in Figure ). From the results of Figure ) and Figure ), at
=150 mm (where the magnet is placed at mid-point along the length of the pipe and is symmetrical on either side with respect to referred mid-point), the magnetic flux density,
is diminished at center part of the fluid flow for both plastic and metal pipe. This holds for both along the transverse x-axis and
tilted
- axis.
The induced tangential component of electric field, observed at various locations of
along transverse
-axis and
tilted x-axis is shown in Figure with an excitation of 400 mA. From the results of Figure ) and Figure ), the induced E-field,
on the plastic surface of pipe for both downstream and upstream of magnet edge (z = 50 and 250 mm) is inverse symmetric with respect to the induced magnetic flux density,
. The
is maximum and it gradually decreases to minimum along the surface of wall and forms the sigmoidal profile. From the results of Figure ) and Figure ), at the location of z =150 mm, the tangential component of
is prominent along the centre line of the fluid flow in the plastic circular pipe and is negligible near the walls of the circular pipe, when measured along the transverse
-axis. The
field is maximum near the walls when measured along the
tilted x-axis as compared to transverse
-axis. In metallic circular pipe, the
is negligible along both transverse
-axis and
tilted
-axis due to the neutralization of charges in fluid flow.
The behaviour of mutual interaction of fluid flow with the induced magnetic and electric fields is similar even at high velocity of fluid at constant electrical conductivity of fluid. The profiles of induced magnetic flux density and E- field at Reynolds number of turbulent flow velocity resemble the results presented in Figures valid for Reynolds number of laminar flow velocity.
Streamline plots pertinent flow variables such as flow velocity as shown in Figure for the plane at
= 0 mm.
Figure 12. Streamline plots for velocity with deformation of magnetic flux density in non-conducting and conducting in duct at various axial positions of , when
= 2029.213, and I = 400 mA.

The deformation of fluid flow velocity is analyzed with the influence of magnetic flux density in the non-conducting and conducting duct as shown in Figure ) and Figure ) respectively. The effect of magnetic flux density on the velocity of fluid flow is maximum at the source of the magnet both in the non-conducting and conducting wall. In a non-conducting wall, the resistive drag force pushes the fluid flow into the middle of the duct. The resistive drag force is high at the near conducting wall as compared to the non-conducting wall hence the skin friction drag increases. The boundary layer thickness increases in the conducting duct. The velocity of fluid flow at center of the duct decreases.
4. Conclusion
This paper presented a three-dimensional simulation model of fluid flow with low electrical conductivity in metallic and non-metallic (plastic) circular pipe in the presence of applied transverse magnetic field. Disturbance of fluid flow is seen in a circular pipe where the interaction of magnetic field with the fluid flow is maximum. The average velocity of the fluid flow decreases for the low Reynolds number and increases for the high Reynolds number. However, fluid flow profile transitions are seen in a circular pipe. The profiles of tangential component of induced magnetic flux density and electric field are graphically explored at various locations of the transverse plane in the direction of fluid flow. The profiles are observed along both transverse x-axis as well as tilted
-axis. The resistive drag force within the metallic pipe increases, leading to a decrease in the induced tangential component of magnetic flux density and the E-field compared to non–metallic pipe. The velocity at the centreline is maximum for metallic circular pipe. The mutual interaction of velocity with the tangential component of induced fields has facilitated better grasp and understanding of the fluid flow behaviour at various locations of transverse cross-sectional plane in metallic and non-metallic circular pipes. This paper has also highlighted the significance of eddy currents in the characterization of induced magnetic flux density and electric field to analyse the fluid flow in a pipe.
Table
Disclosure statement
No potential conflict of interest was reported by the author(s).
Additional information
Funding
References
- ABB. Electromagnetic Flow meters. https://new.abb.com/products/measurement-products/flow/electromagnetic-flowmeters:(accessed 12th March 2020)
- Albets-Chico, X., Radhakrishnan, H., Kassinos, S., & Knaepen, B. (2011). Numerical simulation of a liquid-metal flow in a poorly conducting pipe subjected to a strong fringing magnetic field. Physics of Fluids, 23(4), 047101. https://doi.org/10.1063/1.3570686
- Bhatti, M. M., Bég, O. A., Ellahi, R., & Abbas, T. (2022). Natural convection non-Newtonian EMHD dissipative flow through a microchannel containing a non-Darcy porous medium: Homotopy perturbation method study. Qualitative Theory of Dynamical Systems, 21(4), 1–15. https://doi.org/10.1007/s12346-022-00625-7
- Bhatti, M. M., Doranehgard, M. H., & Ellahi, R. (2022). Electro‐magneto‐hydrodynamic Eyring‐Powell fluid flow through micro‐parallel plates with heat transfer and non‐Darcian effects. Mathematical Methods in the Applied Sciences.
- Dong, S., Liu, L. S., & Ye, X. M. (2017). Instability of MHD Flow in the duct with electrically perfectly conducting walls. Journal of Applied Fluid Mechanics, 10(5), 1293–1304. https://doi.org/10.18869/acadpub.jafm.73.242.27310
- Fonseca, W. D. S., Araújo, R. C., Silva, M. D. O. E., & Cruz, D. O. D. A. (2021). Analysis of the Magnetohydrodynamic Behavior of the Fully Developed Flow of Conducting Fluid. Energies, 14(9), 2463. https://doi.org/10.3390/en14092463
- Gnaneswara Reddy, M., & Ferdows, M. (2021). Species and thermal radiation on micropolar hydromagnetic dusty fluid flow across a paraboloid revolution. Journal of Thermal Analysis and Calorimetry, 143(5), 3699–3717. https://doi.org/10.1007/s10973-020-09254-1
- Hua, T. Q., & Walker, J. S. (1995). MHD flow in rectangular ducts with inclined non-uniform transverse magnetic field. Fusion Engineering and Design, 27(1–2), 703–710. https://doi.org/10.1016/0920-3796(94)00161-Y
- Jaroslav, Š. (2016). Contribution to investigation of turbulent mean-flow velocity profile in pipe of circular cross-section. In AIP Conference Proceedings (p. 20010). AIP Publishing LLC.
- Li, Y., & Zikanov, O. (2013). Laminar pipe flow at the entrance into transverse magnetic field. Fusion Engineering and Design, 88(4), 195–201. https://doi.org/10.1016/j.fusengdes.2013.01.087
- Malekzadeh, A., Heydarinasab, A., & Dabir, B. (2011). Magnetic field effect on fluid flow characteristics in a pipe for laminar flow. Journal of Mechanical Science and Technology, 25(2), 333–339. https://doi.org/10.1007/s12206-010-1223-5
- Megahed, A. M., Reddy, M. G., & Abbas, W. (2021). Modeling of MHD fluid flow over an unsteady stretching sheet with thermal radiation, variable fluid properties and heat flux. Mathematics and Computers in Simulation, 185, 583–593. https://doi.org/10.1016/j.matcom.2021.01.011
- Murgatroyd, W. (1953). CXLII. Experiments on magneto-hydrodynamic channel flow. The London, The London, Edinburgh, and Dublin Philosophical Magazine and Journal of Science, 44(359),1348–1354.
- Pothérat, A., Sommeria, J., & Moreau, R. (2002). Effective boundary conditions for magnetohydrodynamic flows with thin Hartmann layers. Physics of Fluids, 14(1), 403–410. https://doi.org/10.1063/1.1423287
- Prasanna Jeyanthi, M., & Ganesh, S. (2022). Numerical solution of steady MHD duct flow in a square annulus duct under strong transverse magnetic field. International Journal of Ambient Energy, 43(1), 2816–2823. https://doi.org/10.1080/01430750.2020.1778085
- Ramesh, K., Reddy, M. G., & Souayeh, B. (2021). Electro-magneto-hydrodynamic flow of couple stress nanofluids in micro-peristaltic channel with slip and convective conditions. Applied Mathematics and Mechanics, 42(4), 593–606. https://doi.org/10.1007/s10483-021–2727-8
- Reddy, M. G., & Kumar, K. G. (2021). Cattaneo-Christov heat flux feature on carbon nanotubes filled with micropolar liquid over a melting surface: A stream line study. International Communications in Heat and Mass Transfer, 122, 105142. https://doi.org/10.1016/j.icheatmasstransfer.2021.105142
- Shercliff, J. A. (1953). Steady motion of conducting fluids in pipes under transverse magnetic fields. In Mathematical Proceedings of the Cambridge Philosophical Society (pp. 136–144). Cambridge University Press.
- Shercliff, J. A. (1956). The flow of conducting fluids in circular pipes under transverse magnetic fields. Journal of Fluid Mechanics, 1(6), 644–666. https://doi.org/10.1017/S0022112056000421
- Tezer-Sezgin, M., & Bozkaya, C. (2008). Boundary element method solution of magnetohydrodynamic flow in a rectangular duct with conducting walls parallel to applied magnetic field. Computational Mechanics, 41(6), 769–775. https://doi.org/10.1007/s00466-006-0139-5
- Wang, J., Gong, C., & Liu, Y. (2006). Characteristics of Induced Potential of Electromagnetic Flow Meter. In 2006 IEEE International Conference on Automation Science and Engineering.