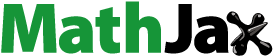
Abstract
The Superheated steam has been shown to be more effective than hot air for drying corn. Modeling studies have been carried out in fluidized bed dryers to determine the moisture and heat transfer characteristics of corn, but there are no modeling studies for a single corn kernel. Knowledge of heat and mass transfer characteristics in a single kernel would produce a better understanding of steam characteristics needed for specific drying rates. The study sought to determine the influence of steam temperature and velocity on the drying rate of a corn kernel. It used computational fluid dynamics (CFD) to model the transfer of heat and moisture between the corn kernel and superheated steam. The simulation used cone geometry to represent the corn kernel. The kernel had characteristic dimensions and 20% initial moisture content. The condition of the steam, which was typical of that found in industry, had temperatures of 120–200°C and velocities of 0.5–1.5 m/s. There was a decrease in the duration of the initial condensation phase as the temperature of superheated steam increased from 120°C to 200°C. The highest steam temperature and velocity resulted in the shortest duration for steam condensation. Contrary to what has been reported, there was no moisture loss from the corn kernel when exposed to superheated steam at 120°C, which may be due to how heat was retained in the drying chamber. Thus, the processes of tapping steam and retaining heat are important elements in the operation of industrial steam driers.
1. Introduction
Proper drying of grains is critical to reducing post-harvest losses and in attaining food security (Kumar & Kalita, Citation2017; Pranto et al., Citation2021). High moisture content in agricultural products encourages the growth of yeast, fungi, mold, and bacteria, thus reducing the shelf life (Bala, Citation2017). The most popular method of preserving grains such as maize is dehydration. Mycotoxin may be present during pre- and post-harvesting and storage of grains (Pfohl-Leszkowicz et al., Citation2002). Myotoxin has been attributed to human and animal illness and death (Afsah-Hejri et al., Citation2013). Dehydration of maize prevents mycotoxin growth. For effective storage of grains, the moisture content needs to be reduced to between 10.0% and 13.0% wet basis (w.b.) (Diarra & Amoah, Citation2019). When shelled corn is dried by the open-sun drying method, the kernels are not only exposed to solar irradiation but also color discoloration occurs.
Most maize processing companies have boilers that supply steam for production purposes. High-pressure waste steam in the range 160–180°C is normally released to the environment (Iyota et al., Citation2001). This is a huge energy loss and therefore presents a great opportunity to tap this steam and use it for drying maize and reducing processing costs. Steam drying is a suitable alternative drying method because there is drying uniformity, color, and nutritional quality is preserved (Liu et al., Citation2023; Mayor et al., Citation2011; Taechapairoj et al., Citation2003). In an existing industrial setup with good infrastructure in place for steam production, superheated steam is used as the drying medium. Studies show that the use of steam in dryers saves on the cost of fossil fuels, and the drying process does not emit noxious gases (Ma et al., Citation2021; Romdhana et al., Citation2015). Additionally, the grains are not directly in contact with hot oxygen-containing gas, thus free from product oxidation.
Superheated steam dryers are commonly used in high moisture applications because they have 50% higher drying efficiencies compared to conventional drying systems (Mujumdar, Citation1995; Pronyk et al., Citation2004). Superheated steam has higher thermal conductivity and the heat capacity compared to air resulting in higher drying rates in superheated steam driers. Danilov and Leonchik (Citation1967) showed that drying with superheated steam accelerates the drying process, making it economical and efficient at atmospheric pressure. Grain drying using superheated steam reduced the drying period compared to conventional dryers. Low-pressure superheated steam dryers are operated below atmospheric pressures in the range of 5–30 kPa (Chou & Chua, Citation2001). Since low-pressure steam dryers operate at lower pressure levels they have poor convective heat transfer and uneven drying and thus the drying process under these conditions is ineffective and inefficient (Devahastin et al., Citation2004;).
A number of variables are critical to the drying of grains. These are grain flow rate, airflow rate, as well as temperature and relative humidity of the drying fluid (Devahastin et al., Citation2004; Mujumdar, Citation1995; Quirijns, Citation2006). Research shows that these operating parameters affect the drying characteristics as well as the grain quality attributes, such as shrinkage, volume, color, and rehydration behavior (Baughman, Gerald Robert, Citation1971). Devahastin et al. (Citation2004) studied low-pressure steam drying of barley and established that the system had poor heat transfer, resulting in lower drying rates compared to hot air drying. Conventional drying techniques have several limitations compared to superheated steam dryers. These include loss of nutrients, color degradation, non-uniform product quality (Sehrawat et al., Citation2016), and a longer drying period (Leeratanarak et al., Citation2006).
In addition, Pronyk et al. (Citation2004) experimented on the steam drying of distillers’ grains and Asian nodules and observed that as steam temperatures increased, so did the drying rates. However, nutritional degradation, such as starch gelatinization as well as gumminess and adhesiveness were observed in Asian nodules. Zielinska and Cenkowski (Citation2012) studied the effect of increasing superheated steam temperatures on the drying rate of coated distillers’ grains. They established that during the initial phases of superheated steam drying, the drying rates increased with increasing steam temperatures. It was also observed that prolonged drying of corn at 160 °C caused cracking, corn darkening, and color discoloration (Baughman, Gerald Robert, Citation1971).
Computational fluid dynamics (CFD) has modeled the drying of corn with superheated steam, which involves multi-phase fluid flows and mass transfer processes. The first corn drying models were semi-empirical, describing just the relationship between moisture content and drying time. Malekjani and Jafari (Citation2018) simulated the superheated steam drying of corn in a spouted bed dryer. They noted that this model was not practical for batch drying because of large computation time. They used the Eulerian technique to create a CFD model for superheated steam drying using a Triangular Spouted Bed Dryer (TSBD). The model was useful as a qualitative design guide and could not account for the annulus section’s plug flow velocity profile. Previous studies (Malekjani and Jafari, Citation2018: Mujumdar, Citation1995; and Zielinska and Cenkowski, Citation2012) focused on the overall effect of drying bulk of corn using superheated steam; however, documentation on the single element approach is very rare. The drying kinetics are greatly affected by the spatial and temporal variation in moisture content and temperature distribution within the corn kernel (Ramachandran et al., Citation2018).
The main aim of this study was to develop a model capable of predicting the temporal and spatial variation of moisture content and temperature variation during the drying of corn using waste superheated steam from industrial processes. The model was used to determine the influence of steam temperature and velocity on the drying rate of a corn kernel. The instantaneous moisture profiles obtained from the simulation gave a detailed insight into the complex drying processes, which included initial condensation, constant drying, and falling rate stages. An understanding of temperature variations with simultaneous moisture reduction in a single corn kernel are important in determining the optimum conditions for drying a collection of corn kernels.
2. Materials and methods
2.1. Numerical modeling
The study entailed a two-phase problem namely; a solid-phase region (corn pellet) and a fluid-phase region (drying medium). The Eulerian–Eulerian approach was used to model multiphase flow because the fluid phase and the solid phase were described as an interpenetrating or interacting continuum (Hamzehei, Citation2011).
2.1.1. Fluid-phase governing equations
The major governing equations of fluid flow and heat transfer are dependent on mass, momentum, and energy conservation equations. The equations are presented below;
2.1.2. Continuity equation
Mass conservation of the fluid is expressed as follows (Erriguible et al., Citation2006; Messai et al., Citation2015; Xiao et al., Citation2013);
where ρss, t and are density of superheated steam (kg/m3), time (s) and velocity component(m/s), respectively.
2.1.3. Conservation of momentum
Momentum conservation of the fluid is expressed as follows (Bernard & Wallace, Citation2002; Ramachandran et. al., Citation2019):
where is the average velocity,
is average pressure, ϑ is the eddy (turbulent) viscosity (kg/ms), and g is the acceleration due to gravity (m/s2).
2.1.4. Energy equation
The differential equation for energy balance for this study is expressed as follows (Kittiworrawatt & Devahastin, Citation2009):
and
where and
in EquationEquation 4
(4)
(4) ) are the operating reference steam temperature for each experiment and the latent heat of vaporization at
respectively,
is the specific heat capacity of superheated steam. H in EquationEquation 3
(3)
(3) ) is the enthalpy in the control volume of superheated steam concerning
is the thermal conductivity of superheated steam and T is steam temperature.
The superheated steam flow was modeled by solving Reynolds-Averaged Navier—Stokes (RANS) EquationEquation 1(1)
(1) -EquationEquation 3
(3)
(3) ).
2.1.5. Solid-phase governing equations
The study utilized the solid phase governing equation, which uses the solution of fluid medium transport equations as its initial guess for its boundary condition. Hence, the solid domain was first modeled using the transport equation of superheated steam to describe the initial boundary condition for heat transfer. The following assumptions were taken into account when developing a mathematical model for the solid domain (Sitompul & Istadi Citation2000; Zhang et al., Citation2013).
The corn is homogeneous, isotropic and non-deformable.
No mass transport resistance in the vapor phase.
The internal temperature and moisture of corn is evenly distributed at the beginning of the drying process.
No shrinkage effects (constant product volume during the entire drying process).
Internal heat and mass transport is described by diffusion only.
The effects of radiation are neglected.
For spherical-shaped solids, the energy and mass balances during the entire drying phase is governed by the diffusion EquationEquation 5(5)
(5) and EquationEquation 6
(6)
(6) , respectively (Prachayawarakorn et al., Citation2006; Chemkhi et al., Citation2005):
where cp is the corn pellet,is the moisture content of the corn pellet (kg water/kg dry basis),
is the specific heat capacity between the Cwet and Cdry,
is the effective diffusivity coefficient,
is the temperature of the corn pellet,
is the density of the corn pellet,
is the thermal conductivity of the corn pellet, r is the instantaneous location of the thin layer of condensate and R is the radius of the corn kernel.
For the case where, then
and
EquationEquation 7(7)
(7) -EquationEquation 8
(8)
(8) ) represents the boundary conditions for heat (; Kittiworrawatt & Devahastin, Citation2009;) and mass transfer (Bernard and Wallace, Citation2002; Ramachandran et. al. Citation2019) for initial condensation phase, respectively.
The film condensation is described by:
where is the temperature of corn pellet at a given moisture content and vapour partial pressure,
is the saturation temperature,
is the mass flux at initial condensation,
is the latent heat of water vapor,
is the dynamic viscosity of water and
is the film condensation heat transfer coefficient of superheated steam.
For the case where then
where is the mass flux at constant rate drying,
is the automatic heat transfer coefficient that is obtained from RANS equation solution in superheated steam region and
is the latent heat. EquationEquation 10
(10)
(10) and EquationEquation 11
(11)
(11) represent the boundary conditions for heat and mass transfer of the evaporated condensed steam (constant drying phase) at the corn surface (Thomkapanich et al., Citation2007).
As evaporation continues, moisture diffusion takes over. This is the falling drying rate period which is described by the boundary condition for heat balance and mass flux at the interface. In this case, and
In EquationEquation 12(12)
(12) and EquationEquation 13
(13)
(13) ,
is the net heat flux penetrating the corn pellet,
is the mass flux at falling rate drying period,
is the specific heat capacity of water vapor,
is the effective moisture diffusivity at the instantaneous time, and M is the moisture content.
The surface temperature is not easy to find and involves the solution of simultaneous heat and mass transfer equations for the solid case. An equilibrium boundary condition that binds both the equations at the fluid-solid interface was described by (Messai et al., Citation2015; Thomkapanich et al., Citation2007) as:
where is the sorption isotherm-isobar,
is the water vapor pressure and
is the saturation pressure.
2.2. Configuration
A 3D mathematical model for the superheated steam drying of a single corn pellet was developed using CFD software ANSYS 2020R2 (https://www.edx.org/course/a-hands-on-introduction-to-engineering-simulations, ANSYS Student 2022 R2 version). A single corn pellet with cone geometry and corn characteristic dimension was designed in the workbench design modeler, as shown in Figure (a). In the fluid domain (drying chamber), a cylinder with a radius of 270 mm and a length of 640 mm was used. The 3D model was positioned at the geometric center of the fluid domain with the apex of the corn facing the inlet of the dryer, as shown in Figure (b).
The computational domain was meshed using ANSYS Mesh. A finer mesh was determined by face sizing the inlet, outlet, symmetry and wall. The volume grid was generated using tetrahedral elements. To capture changes in the variables and form finer grids near the interface and wall, the resolution of the mesh near the walls was increased by adding five inflation layers with structured mesh layout. Inflation layers are additional layers that are added near the interface to assist in capturing boundary layer gradients (Ramachandran et al., Citation2019). Inflation refines the mesh closer to the solid surface and makes it possible to capture boundary layer gradients, as shown in Figure . The fluid-solid interface was given a denser inflation layer to capture inward and outward diffusion.
2.3. Initial and boundary conditions
A single corn pellet was positioned at the geometric center of a cylinder defining the drying chamber. A wet corn pellet at ambient air temperature T0 = 25 °C and moisture content M0 = 20% w.b. (kg water/kg wet solid) was subjected to convective drying by superheated steam temperatures Tss. The study utilized the temperature range of 120–200 °C at atmospheric pressure since these are the conditions for waste steam from processing industries (Taechapairojet et al., Citation2006).
The thermo-physical properties of the corn pellet were defined in ANSYS FLUENT as a function of their moisture content and temperature. Table shows the physical properties of corn at 20% moisture content.
Table 1. Physical properties of corn at 20% moisture content
The initial thermo-physical properties of the superheated steam were called from the built in IAPWS-IF97 library of ANSYS FLUENT with superheated steam temperatures ranging between 110°C and 500°C and at a pressure of 0.5 to 100 kPa. Fluid phase properties are a function of temperature and individual components are evaluated based on the polynomial curve fits of NASA type.
The initial and boundary conditions for the moisture diffusion at initial condensation are given by:
in EquationEquation (17)
(17)
(17) is the maximum moisture content for which the single corn pellet can hold. EquationEquation 16
(16)
(16) and (17) represent the minimum moisture content at the centre and at the surface of the single corn pellet.
The mass transfer boundary conditions for the evaporation of condensed steam (constant drying rate period) at corn surface is described by.
The quantity in EquationEquation 25
(25)
(25) , EquationEquation 25
(25)
(25) and (20) is the period at the end of the removal of condensate (s), whereas k is the drying constant (kg evaporated water/s. kg wet matter).
When the moisture content in the single corn pellet dropped below 13% kg/kg wet basis, further moisture removal occurred in the falling-drying-rate phase (Ramachandran et al., Citation2019). The associated boundary and initial conditions for moisture diffusion in this phase are
The quantity in EquationEquation 25
(25)
(25) , EquationEquation 25
(25)
(25) and EquationEquation 25
(25)
(25) is the drying period at the end of constant drying rate phase (s).
At the fluid-solid interface, a zero-velocity boundary condition was assumed. The boundary condition governing the transport equation at the interface are given by (Messai et al., Citation2015; Thomkapanich et al., Citation2007) as:
2.4. Mesh sensitivity analysis
A grid independence test was done to investigate mesh sensitivity analysis to the number of elements. The unstructured grid was constructed using ANSYS-Mesh software. The results are shown in Figure . A plane was created near the grain to allow observation of velocity fluctuation with default grid elements of 61,450 and increases of the elements to 125,000, 179,859, 254,273, 354,997, 486,977, and 494,791 at a velocity of 1.0 m/s. The numerical grid was independent at 254,273 elements, beyond which the velocity remained constant.
2.5. Implementation of the model
The fluid and the solid domains were connected by a fluid-solid interface, and the fluid and solid boundary conditions were combined at the interface. The fluid domain was used to calculate temperature, pressure, and velocity flow variables for the drying chamber’s output (Faghani et al., Citation2010). A single time-step was used to solve the flow field equations. The boundary condition information was then transferred to the corn pellet (solid), where the diffusion model was consequently run for the same time step. The first time-boundary step’s information was then transferred to the flow field, which was then utilized to simulate the following time-step (Defraeye et al., Citation2012). Using the multi-configuration capability of FLUENT, the steady-state simulation results obtained were used as the initial boundary conditions for the transient-state analysis of the transient-state drying simulation (Ramachandran et al., Citation2018). Each time-step had 10 iterations and a variable time-step size as the simulation’s (drying) duration increased. The recommendation of Gulati et al. (Citation2015) was adopted by using a 1-s time step for the first 3 min, after which a 30-s time step was used as condensation was complete. A finite volume-based solver was used in solving the model equations. A mass source term was used as the initial condensation transfer equation on the fluid-solid interface. The mass taken from the superheated steam interface was added to the solid side interface in the condensate state resulting from condensation. Once the initial condensation was complete, the solid’s wall heat transfer coefficient was dependent on the wall heat transfer coefficient obtained from the solutions of the flow field turbulence model and conservation equations (Sun et al., Citation2017) rather than any empirical equation.
The boundary requirements of the transport equation for the solid domain were determined by the Navier-Stokes equations in the fluid domain (Erriguible et al., Citation2006). Data provided by the CFD code to solve conservation equations in the corn pellet for one single step were computed by the remaining boundary conditions EquationEquation 10(10)
(10) and EquationEquation 11
(11)
(11) . SIMPLEC scheme (Khawaja & Moatamedi, Citation2018) was used for pressure velocity coupling. The pressure was discretized by second order upwind scheme while, momentum, turbulent kinetic energy and turbulent dissipation rate terms were discretized using QUICK schemes (Song et al., Citation2000). The conditions for performing the simulations were as follows. The first test was carried out at 120 ºC and had three simulations at 0.5 m/s, 1.0 m/s and 1.5 m/s. The second and third test at 160 ºC and 200 ºC, respectively, also had three simulations at the same fluid velocities.
3. Results and discussion
3.1. Analysis of spatial variation of moisture content and temperature distribution
The changes in moisture content and temperature within the corn pellet were evaluated by the model. Figures show the moisture and temperature distribution in a corn kernel at T3 = 200 ºC and T2 = 160 ºC at v1 = 0.5 m/s, v2 = 1.0 m/s and v3 = 1.5 m/s of drying. The spatial variation of moisture content and temperature distribution as a result in change in velocity of superheated stem was found that moisture diffusivity of corn pellet increased with an increase in superheated steam temperature and velocity, respectively, while drying using superheated steam.
Figure 4. Moisture content profiles (A) and temperature distribution (B) for superheated steam temperature T3 = 200 ºC and superheated steam velocities v1 = 0.5 m/s (i), v2 = 1.0 m/s (ii) and v3 = 1.5m/s (iii) at 100s.

Figure 5. Moisture content profiles (A) and temperature distribution (B) for superheated steam temperature T2 = 160 ºC and superheated steam velocities v1= 0.5 m/s (i), v2 = 1.0 m/s (ii) and v3 = 1.5m/s (iii) at 100s.

The apex of the corn was geometrically positioned directly facing the inlet of the dryer, which allowed the steam to hit the apex first and is then deflected to the entire surface of the grain. The darker shades of red at the apex and center of the corn denote moisture loss to superheated steam is higher than at the sides or toward the periphery of the corn, which was attributed to the positioning of the cone. Due to the shape of the corn, moisture gradients between the superheated steam and the boundaries are higher at the apex and center, thus resulting in higher drying rates compared to the layers at the periphery or sides of the corn.
Figures also illustrate the difference in heat transfer at different drying temperatures. The variation in temperature at the corn at different locations suggests that at the apex of the corn, heat transfer was fastest for both 160ºC and 200ºC and superheated steam was less obstructed. The resulting increase in surface temperature of the grain is shown to be due to red coloration.
The colors of the corn from the apex to the periphery varied with shades from dark to light red and shades of blue from turquoise. Moreover, the varied superheated steam temperatures of 160ºC and 200ºC showed different colors and intensities. This suggests that heat transfer is not uniform. Comparing the color intensity, dark red shades were more evident at 200 ºC than at 160 ºC in the corn. This was a clear indication that the amount of heat transferred to and absorbed by the corn at T3 = 200ºC was comparatively higher than at T2 = 160ºC. Therefore, heat and mass transfer increased as the superheated steam flow rate increased. The effect of velocity on diffusivity was more predominant for the drying at higher superheated steam temperatures 200°C than at lower superheated steam temperature 160°C Figures . A similar trend in diffusivity with temperature and velocity of drying medium was reported by other researchers (Golpour et al., Citation2017; Koukouch et al., Citation2017; Uddin et al., Citation2016).
3.2. Effect of superheated steam temperatures on drying rates
The study sought to show the spatial variation of moisture content and temperature distribution at different superheated steam temperatures; T1 = 120°C, T2 = 160°C and T3 = 200°C at superheated steam velocities (v1 = 0.5 m/s, v2 = 1.0 m/s and v3 = 1.5 m/s). The drying rates at different time intervals were predicted as shown in Figures .
Figure 6. Predicted moisture content and drying temperature at superheated steam velocities at 200 ºC.

Figure 7. Predicted moisture content and drying temperature at superheated steam velocities at 160 ºC.

Figure 8. Predicted the moisture content and drying temperature at superheated steam velocities at 120 °C.

The result in Figure depicts a drying curve consisting of three phases: the initial heating phase (a), the constant drying rate phase (b), and the falling rate phase (c). The study established the drying times for reducing the moisture content to 13% w.b. as approximately 70s, 97s and 130s for superheated steam velocities of 1.5 m/s,1.0 m/s and 0.5 m/s, respectively, at superheated steam temperature 200ºC. However, at superheated steam temperatures of 200 ºC the drying period for steam with velocity v3 = 1.5 m/s was shortest in moisture reduction to the desired level compared to 1.0 m/s and 0.5 m/s. According to Figure , as the superheated steam temperature approaches 100ºC (the boiling point), the desired drying rates were achieved for all superheated steam velocities (1.5 m/s, 1.0 m/s and 0.5 m/s). Therefore, drying rate increased with increase in steam velocity in the constant drying rate phase. The results for the initial phase showed a rapid increase in moisture content from 20 to 24.5% w.b. at v1= 0.5 m/s, 20 to 22 % w.b. at v2= 1.0 m/s and 20 to 21 % w.b. at v3= 1.5 m/s at various time intervals of 0-40s, 0-30s, and 0-25s, respectively.
The study also aimed to establish the effect of superheated steam temperature T2 = 160ºC on the drying behavior of corn at varying superheated steam velocities (1.5 m/s, 1.0 m/s and 0.5 m/s). The result in Figure was characterized by a rapid increase in moisture content from 20 to 26.5% w.b. at v1 = 0.5 m/s, 20 to 25.1 % w.b. at v2= 1.0 m/s and 20 to 23.5 % w.b. at v3 = 1.5 m/s at time intervals of 0–63 s, 0–58 s and 0–51 s, respectively, describing the initial phase (a).
A rapid heat transfer at superheated steam velocity of 1.5 m/s stimulated a quick loss of moisture, followed by 1.0 m/s and finally 0.5 m/s. In the constant drying rate phase (b), at superheated steam velocity of 1.5 m/s the accumulation of heat in the corn is null, and all sensible heat transferred to the solid from superheated, heated steam causes evaporation of moisture. This was not the case for superheated steam velocities of 1.0 m/s and 0.5 m/s. As superheated steam temperature approached 100 ºC (boiling point) the desired drying rates was achieved for superheated steam conditions 160 ºC at 1.5 m/s.
Finally, Figure illustrates the drying behavior for corn drying at a superheated steam temperature of 120 ºC and different flow velocities (1.5 m/s, 1.0 m/s and 0.5 m/s). It was established that the rate of water loss from the corn was quite low due to the large quantity of water vapor in the chamber. The results in Figure show a rapid increase in moisture content from 20 to 29.1% w.b. at v1= 0.5 m/s, 20 to 27.99% w.b. at v2 = 1.0 m/s and 20 to 25.1% w.b. at v3 = 1.5 m/s at various time intervals of 0–80 s, 0–83 s and 0–91 s, respectively, describing the initial phase (a). Nevertheless, it was observed that the drying rate period for superheated steam with velocity v3 = 1.5 m/s was faster in reducing moisture but not to the desired level. It was further established that the desired drying rates were not achieved for all the operating conditions. However, this also depends on the solid’s structure and drying period.
Figure shows the predicted points of moisture content (continuous lines) of a single corn kernel of this study compared with the experimental points of moistures content of a distiller’s spent grain coated with soluble (dotted lines) using superheated steam of Ramachandran, (Citation2019). Due to different drying conditions, the data sets obtained had wide variation across the database. As such, the data were normalized using logarithm to transform the data sets to be on a similar scale to ensure uniformity and comparison of the two data sources. The results obtained showed that the simulated model had similar trend to the results obtained while conducting an experiment using superheated steam.
Figure 9. Predicted points of moisture content (continuous lines) of a single corn kernel compared with the experimental points of moistures content of a distiller’s spent grain (dotted lines) using superheated steam (Ramachandran, Citation2019).

4. Conclusion
The study investigated the drying characteristics of a single corn pellet using superheated steam with the aid of fluid dynamics models, specifically the Eulerian–Eulerian technique. It was established that as superheated steam velocity increases, the drying rate also increases. At the same velocity, an increase in the temperature of superheated steam results in an increase in the drying rates. Superheated steam velocity and temperature influence both heat and mass transfer and are important in regulating the drying of corn.
5. Recommendation
The effect of superheated steam on the corn’s quality during the period of falling rates needs to be examined in more detail. It is important to compare simulation and experimentation results for different superheated steam temperatures and velocities. Additionally, since the study’s main focus was on the spatial variation of moisture content at various superheated steam temperatures, a more focused investigation should be conducted to determine the effective moisture content’s value, methodology, and application in CFD models. Future modeling and simulation should take into account a study on the change in the corn’s physical dimensions and geometric position at various angles to the direction of the flow of superheated steam.
Nomenclature
= | Surface area (m2) | |
= | Specific heat of solid particle (J Kg−1 K−1) | |
= | Specific heat capacity of steam (J Kg−1 K−1) | |
= | Specific heat capacity of water (J Kg−1 K−1) | |
Cp | = | Specific heat capacity (J Kg−1 K−1) |
D | = | Moisture diffusivity (m2 s−1) |
g | = | Acceleration due to gravity (m s−2) |
H | = | Enthalpy in the control volume (kJ kg−1) |
= | Heat transfer coefficient (W m−2 K−1) | |
k | = | Turbulence kinetic energy |
kt | = | Thermal conductivity (W/(m K)) |
= | Mass flux (kg m−2 s−1) | |
M | = | Moisture content (kg of water/kg of dry mass) |
= | Pressure (Pa) | |
q | = | Net heat flux (W/m2) |
= | Drying time (s) | |
R | = | Universal gas constant (8.314J K−1 mol−1) |
T | = | Temperature (K) |
v | = | Velocity component (m/s). |
x | = | Distance along x axis (m) |
y | = | Distance along y axis (m) |
z | = | Distance along z axis (m) |
Greek Symbols | = | |
= | Dissipation rate (-) | |
ρ | = | Density (kg/m3) |
μ | = | Dynamic viscosity (kg/(m s)) |
λ | = | Latent heat (J/kg) |
σk | = | Prandtl number in k-equation (-) |
σw | = | Prandtl number in ω-equation (-) |
∇ | = | Laplace gradient function |
ϑ | = | Eddy viscosity (kg/(m s)) |
Δ | = | Change |
Subscripts | = | |
cp | = | Corn |
cdr | = | Constant rate drying |
e | = | Equilibrium |
eff | = | Effective value |
film | = | Condensed film (water) |
fdr | = | Falling rate drying period |
ih | = | Initial condensation |
ref | = | Reference value |
ss | = | Superheated steam |
t | = | Instantaneous time |
ds | = | Solid particles |
sat | = | Saturation |
= | Vapor |
Disclosure statement
No potential conflict of interest was reported by the authors.
References
- Afsah-Hejri, L., Jinap, S., Hajeb, P., Radu, S., & Shakibazadeh, S. (2013). A review on mycotoxins in food and feed: Malaysia case study. Comprehensive Reviews in Food Science and Food Safety, 12(6), 629–16. https://doi.org/10.1111/1541-4337.12029
- Bala, B. K. (2017). Drying and storage of cereal grains (Second ed.). Wiley Blackwell.
- Baughman, Gerald Robert. (1971) . Experimental study and simulation of a concurrent-flow grain dryer. The Ohio State University.
- Bernard, P. S., & Wallace, J. M. (2002). Turbulent Flow: Analysis, Measurement and Prediction (First ed.). Wiley.
- Bhise, S., Kaur, A., & Manikantan, M. (2014). Moisture dependent physical properties of maize (PMH-1). Acta Alimentaria, 43(3), 394–401. https://doi.org/10.1556/aalim.43.2014.3.5
- Chemkhi, S., Zagrouba, F., & Bellagi, A. (2005). Modelling and simulation of drying phenomena with rheological behaviour. Brazilian Journal of Chemical Engineering, 22, 153–163.
- Chou, S. K., & Chua, K. J. (2001). New hybrid drying technologies for heat sensitive foodstuffs. Trends in Food Science & Technology, 12(10), 359–369. https://doi.org/10.1016/S0924-2244(01)00102-9
- Danilov, O. L., & Leonchik, B. I. (1967). Advantages of using superheated steam at atmospheric pressure in drying processes. Journal of Engineering Physics, 13, 157–159.
- Defraeye, T., Blocken, B., Derome, D., Nicolai, B., & Carmeliet, J. (2012). Convective heat and mass transfer modelling at air–porous material interfaces: Overview of existing methods and relevance. Chemical Engineering Science, 74, 49–58. https://doi.org/10.1016/j.ces.2012.02.032
- Devahastin, S., Suvarnakuta, P., Soponronnarit, S., & Mujumdar, A. S. (2004). A comparative study of low-pressure superheated steam and vacuum drying of a heat-sensitive material. Drying Technology, 22(8), 1845–1867. https://doi.org/10.1081/DRT-200032818
- Diarra, M., & Amoah, R. S. (2019). Physical factors in the hermetic super grain bag and effect on the larger grain borer and aflatoxin production by aspergillus flavus during the storage of ‘Obatanpa’ maize (Zea mays L.) variety. Journal of Stored Products Research, 83, 84–91. https://doi.org/10.1016/j.jspr.2019.05.016
- Erriguible, A., Bernada, P., Couture, F., & Roques, M. (2006). Simulation of convective drying of a porous medium with boundary conditions provided by CFD. Chemical Engineering Research & Design, 84(2), 113–123. https://doi.org/10.1205/cherd.05047
- Faghani, E., Maddahian, R., Faghani, P., & Farhanieh, B. (2010). Numerical investigation of turbulent free jet flows issuing from rectangular nozzles: The influence of small aspect ratio. Archive of Applied Mechanics, 80(7), 727–745. https://doi.org/10.1007/s00419-009-0340-z
- Golpour, H., Boravelli, T., Smith, J., & Safarpour, H. R. (2017). Production of Syngas from Biomass Using a Downdraft Gasifier. International Journal of Engineering Research and Applications, 07(06), 61–71.
- Gulati, T., Zhu, H., Datta, A. K., & Huang, K. (2015). Microwave drying of spheres: Coupled electromagnetics-multiphase transport modeling with experimentation. Part II: Model validation and simulation results. Food and Bioproducts Processing, 96, 326–337. https://doi.org/10.1016/j.fbp.2015.08.001
- Hamzehei, M. (2011). CFD modeling and simulation of hydrodynamics in a fluidized bed dryer with experimental validation. International Scholarly Research Notices, 2011, 1–9. https://doi.org/10.5402/2011/131087
- Iyota, H., Nishimura, N., Yoshida, M., & Nomura, T. (2001). Simulation of superheated steam drying considering initial steam condensation. Drying Technology, 19(7), 1425–1440. https://doi.org/10.1081/DRT-100105298
- Khawaja, H., & Moatamedi, M. (2018). Semi-Implicit Method for Pressure-Linked Equations (SIMPLE) – solution in MATLAB®. The International Journal of Multiphysics, 12(4), 313–326.
- Kittiworrawatt, S., & Devahastin, S. (2009). Improvement of a mathematical model for low-pressure superheated steam drying of a biomaterial. Chemical Engineering Science, 64(11), 2644–2650. https://doi.org/10.1016/j.ces.2009.02.036
- Koukouch, A., Idlimam, A., Asbik, M., Sarh, B., Izrar, B., Bostyn, S., Bah, A., Ansari, O., Zegaoui, O., & Amine, A. (2017). Experimental determination of the effective moisture diffusivity and activation energy during convective solar drying of olive pomace waste. Renewable Energy, 101, 565–574.
- Kumar, D., & Kalita, P. (2017). Reducing postharvest losses during storage of grain crops to strengthen food security in developing countries. Foods, 6(1), 8. https://doi.org/10.3390/foods6010008
- Leeratanarak, N., Devahastin, S., & Chiewchan, N. (2006). Drying kinetics and quality of potato chips undergoing different drying techniques. Journal of Food Engineering, 77(3), 635–643. https://doi.org/10.1016/j.jfoodeng.2005.07.022
- Liu, Y., Li, M., Jiang, D., Guan, E., Bian, K., & Zhang, Y. (2023). Superheated steam processing of cereals and cereal products: A review. Comprehensive Reviews in Food Science and Food Safety, 22(2), 1360–1386. https://doi.org/10.1111/1541-4337.13114
- Malekjani, N., & Jafari, S. M. (2018). Simulation of food drying processes by Computational Fluid Dynamics (CFD); recent advances and approaches. Trends in Food Science & Technology, 78, 206–223.
- Ma, Y., Sang, S., Xu, D., Jin, Y., Chen, Y., & Xu, X. (2021). The contribution of superheated steam treatment of wheat flour to the cake quality. LWT, 141, 110958. https://doi.org/10.1016/j.lwt.2021.110958
- Mayor, L., Moreira, R., & Sereno, A. (2011). Shrinkage, density, porosity and shape changes during dehydration of pumpkin (Cucurbita pepo L.) fruits. Journal of Food Engineering, 103(1), 29–37. https://doi.org/10.1016/j.jfoodeng.2010.08.031
- Messai, S., Sghaier, J., El Ganaoui, M., Chrusciel, L., & Gabsi, S. (2015). Low-pressure superheated steam drying of a porous media. Drying Technology, 33(1), 103–110. https://doi.org/10.1080/07373937.2014.933843
- Mujumdar, A. S. (1995). Handbook of Industrial Drying (2nd ed) (pp. 1071–1086). Dekker New York.
- Pfohl-Leszkowicz, A., Petkova-Bocharova, T., Chernozemsky, I. N., & Castegnaro, M. (2002). Balkan endemic nephropathy and associated urinary tract tumours: A review on aetiological causes and the potential role of mycotoxins. Food Additives & Contaminants, 19(3), 282–302. https://doi.org/10.1080/02652030110079815
- Prachayawarakorn, S., Prachayawasin, P., & Soponronnarit, S. (2006). Heating process of soybean using hot-air and superheated-steam fluidized-bed dryers. Lwt-Food Science and Technology, 39(7), 770–778. https://doi.org/10.1016/j.lwt.2005.05.013
- Pranto, T. H., Noman, A. A., Mahmud, A., & Haque, A. B. (2021). Blockchain and smart contract for IoT enabled smart agriculture. Peer J Computer Science, 7, e407. https://doi.org/10.7717/peerj-cs.407
- Pronyk, C., Cenkowski, S., & Muir, W. E. (2004). Drying foodstuffs with superheated steam. Drying Technology, 22(5), 899–916. https://doi.org/10.1081/DRT-120038571
- Quirijns, E. J. (2006). Modelling and dynamic optimisation of quality indicator profiles during drying. s.N. S.l).
- Ramachandran, R. P., Akbarzadeh, M., Paliwal, J., & Cenkowski, S. (2018). Computational fluid dynamics in drying process modelling—a technical review. Food and Bioprocess Technology, 11(2), 271–292. https://doi.org/10.1007/s11947-017-2040-y
- Ramachandran, R. P., Paliwal, J., & Cenkowski, S. (2019). Computational modelling of superheated steam drying of compacted distillers’ spent grain coated with solubles. Food and Bioproducts Processing, 116, 63–77.
- Romdhana, H., Bonazzi, C., & Esteban Decloux, M. (2015). Superheated steam drying: An overview of pilot and industrial dryers with a focus on energy efficiency. Drying Technology, 33(10), 1255–1274. https://doi.org/10.1080/07373937.2015.1025139
- Sehrawat, R., Nema, P. K., & Kaur, B. P. (2016). Effect of superheated steam drying on properties of foodstuffs and kinetic modeling. Innovative Food Science & Emerging Technologies, 34, 285–301. https://doi.org/10.1016/j.ifset.2016.02.003
- Sitompul, J. P., & Istadi, I. (2000). Alternating direction implicit method for solving equations of 2-D heterogeneous model of deep-bed grain drying. Proceedings of the Institut Teknologi Bandung, 32(1), 425–433.
- Song, B., Liu, G. R., Lam, K. Y., & Amano, R. S. (2000). On a higher‐order bounded discretization scheme. International Journal for Numerical Methods in Fluids, 32(7), 881–897.
- Sun, F., Yao, Y., Xiangfang, L., & Zhao, L. (2017). Type curve analysis of superheated steam flow in offshore horizontal wells. International Journal of Heat & Mass Transfer, 113, 850–860. https://doi.org/10.1016/j.ijheatmasstransfer.2017.05.105
- Taechapairoj, C., Dhuchakallaya, I., Soponronnarit, S., Wetchacama, S., & Prachayawarakorn, S. (2003). Superheated steam fluidised bed paddy drying. Journal of Food Engineering, 58(1), 67–73. https://doi.org/10.1016/S0260-8774(02)00335-7
- Taechapairoj, C., Prachayawarakorn, S., & Soponronnarit, S. (2006). Modelling of parboiled rice in superheated-steam fluidized bed. Journal of Food Engineering, 76(3), 411–419.
- Thomkapanich, O., Suvarnakuta, P., & Devahastin, S. (2007). Study of intermittent low- pressure superheated steam and vacuum drying of heat sensitive material. Drying Technology, 205(1), 25. https://doi.org/10.1080/07373930601161146
- Uddin, Z., Suppakul, P., & Boonsupthip, W. (2016). Effect of air temperature and velocity on moisture diffusivity in relation to physical and sensory quality of dried pumpkin seeds. Drying Technology, 34, 1423–1433.
- Xiao, Z., Wu, N., & Liu, X. (2013). Experiment on flow characteristics of fluidized bed drying with superheated steam. Transactions of the Chinese Society for Agricultural Machinery, 44(7), 183–186.
- Zhang, S., Kong, N., Zhu, Y., Zhang, Z., & Chenghai, X. (2013). 3D model-based simulation analysis of energy consumption in hot air drying of corn Kernels. Mathematical Problems in Engineering, 2013(2), 1–12. https://doi.org/10.1155/2013/579452
- Zielinska, M., & Cenkowski, S. (2012). Superheated steam drying characteristic and moisture diffusivity of distillers’ wet grains and condensed distillers’ solubles. Journal of Food Engineering, 109(3), 627–634. https://doi.org/10.1016/j.jfoodeng.2011.06.017