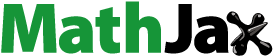
Abstract
In this work, an empirically derived multi-domain model of a light-emitting diode (LED) luminaire is proposed. The optical, electrical, and thermal characteristics of LEDs are obtained from the data sheets provided by manufacturers. Both transient and steady-state performance of LED luminaire were realized theoretically and validated against experimental findings from earlier investigations. The difficulties encountered in creating an ideal LED luminaire are discoursed and examined. Most of the studies on LED luminaires described in prior works ignored the influence of luminaire housing and optics on the thermal management of the luminaire. Knowledge of thermal time constants of LED luminaires is important, as they decide the rate of luminous flux decay when LED lighting systems are used for long periods of operation. Thermal time constants also decide the time taken by the LED junction to reach steady state with its surrounding. From the study it is inferred that the increase in junction temperature and deterioration of luminous flux is controlled by the product of the single most dominant thermal resistance from the junction to a point along the heat conduction path and sum of all the downstream thermal capacitances. This is true as in most cases LED device thermal capacitance is very less related to the thermal capacitance of the heat-sink, hence the product of single most dominant thermal resistance and thermal capacitance of heat-sink decide the rate of rising of junction temperature or luminous flux deterioration.
Public interest statement
In this work, a method to examine the performance of a light-emitting diode (LED) luminaire using in-situ measurements is discussed. The methodology discussed in this work allows comparing different LED products using a standard metric. LED is a semiconductor device packaged in an integrated circuit. Light is emitted by LEDs from their junction. LED junction is subjected to thermal stress during operation. LEDs convert only a fraction of the applied electrical power to light and the rest needs to be dissipated as heat into the ambient. Hence, the thermal resistance along the heat conduction path from the junction to the ambient must be minimum. Higher the thermal resistance higher will be the operating junction temperature, resulting in derating of device performance and causing accelerated aging and higher failures. Semiconductor manufacturers provide thermal metrics at the device level. For a complete luminaire, the thermal metric needs to be estimated experimentally.
1. Introduction
Light-emitting diodes (LEDs) operate in optical, electrical, and thermal domains simultaneously. Understanding their multi-domain operation is key to improve overall LED luminaire design. The multi-domain operation of a LED luminaire provides a valuable understanding of its lifetime performance and reliability. This work discusses the theory behind the multi-domain operation of a LED luminaire. Prior works in literature have discussed multi-domain operations of LEDs and their products using mathematical models and tools (Alexeev et al., Citation2019; Górecki & Ptak, Citation2020, Citation2021; Ibrahim et al., Citation2021; Janicki et al., Citation2020; Ke et al., Citation2016, Citation2018; Pohl et al., Citation2020; Poppe, Citation2012, Citation2015; Poppe et al., Citation2009). Most of these studies have analyzed LED luminaires during their prototyping phase. This study proposes methods to empirically model multi-domain operations of LED luminaires available in the market to relate their rated characteristics to the multi-domain theory of LED lighting systems. The lifetime performance of LED products is affected by thermal stress (van Driel et al., Citation2011). Studies have reported the effect of thermal stress on light output. A rise in junction temperature causes the luminous flux to fall (Farkas et al., Citation2005; Liang et al., Citation2017; Rencz et al., Citation2005). The structure and material of LED devices also affect the magnitude of luminous flux drop with temperature. Uncontrolled thermal stress is responsible for accelerated aging and undesired color characteristics (Keppens et al., Citation2010). The scale of electron-hole recombination inside the light-emitting layer of the LED is defined as “Luminescent efficiency” (Baureis, Citation2005; Bergman & McHale, Citation2012; Li, Citation1993; Ralston & Lorimor, Citation1977; Williams & Hall, Citation1978). During radiative recombination, an electron in the conduction band recombines with a hole in the valence band and surplus energy during this process is emitted in the form of light. The luminescent efficiency of LEDs depends on the radiative recombination rate. Thermal issues affect the luminescent efficiency of LEDs. There are many other ways in which electron-hole pairs can recombine, all these processes adversely affect LED luminescent efficiency. Maximizing the radiative recombination rate and minimizing all other recombination rates improves luminescent efficiency. Luminescent efficiency drops with increasing junction temperature.
Mathematical representation of optical, electrical, and thermal characteristics of LEDs explains their interdependence on each other. Junction temperature measurement is a key stride in the thermal characterization of LED lighting products. Junction temperature cannot be measured directly, but it can be measured indirectly through forward voltage measurements (Xi & Schubert, Citation2004). Forward voltage based junction temperature measurement is limited to DC-driven LED lighting products. In this work, junction temperature estimation by measuring either luminous flux or power consumed is discussed. This method is independent of the type of power supply driving the LED luminaire. Knowledge of thermal time constants of LED products is essential as they establish the rate of light output decay when LED luminaires operate for prolonged periods. Thermal time constants are also accountable for influencing the time taken by the LED junction to reach a steady state with the ambient. Prior studies have reported the thermal characterization of LED devices using advanced equipment that works on the principle of transient forward voltage measurement and structure functions (Poppe, Citation2012, Citation2015). However, studies on thermal characterization at the LED luminaire level are rare. In this work, the principle behind the working of a LED luminaire is examined. This theory is employed to design experiments for characterizing the multi-domain operation of a LED luminaire empirically.
In past researches, the steady-state junction temperature of a LED lighting system was calculated based on the thermal resistance value from junction-to-ambient and power to be dissipated into the ambient. This work proposes the experimental determination of junction-to-ambient thermal resistance and junction temperature. Modern LED luminaires have high power ratings, there is a need for validating their design. This work proposes a methodology to assess the final design of a LED lighting product independent of its internal structure and design details. This study examines methods to obtain a multi-domain representation of a LED luminaire empirically. Steps to obtain an internal thermal RC network that links the junction to the ambient of a LED luminaire are studied. The proposed methodology is useful in obtaining multi-domain models of LED products, these models can be used in software tools for creating LED luminaires for different lighting applications. In this work, a multidomain empirical model of a LED luminaire for design confirmation as well as for realization in simulation programs is proposed. The light output is projected reliably from the thermal and electrical operating conditions of the luminaire.
2. Electro-thermal (
) model of a LED luminaire
A driver (current or voltage regulator) controls the power requirements of a LED lighting system. LED drivers play an important role in preventing damage to LEDs by regulating their forward voltage () which changes with temperature, thereby avoiding thermal runaway while delivering a constant current to LEDs. A typical off-line switching LED driver is shown in Figure 23, 24], is a self-contained power supply whose outputs are matched to the electrical characteristics of the LED or LEDs. LED drivers regulate the power level supplied to the LEDs as the electrical characteristics change with temperature (Bender et al., Citation2012; Chiu et al., Citation2010; Fuada et al., Citation2017; Trujillo et al., Citation2017). Most off-line LED drivers consist of a Buck, Buck-Boost, or flyback converter to convert the rectified line voltage into a required voltage for driving LEDs (Hui et al., Citation2010; Modepalli & Parsa, Citation2014, Citation2015; Shao, Citation2009). Current sensing resistance
provides necessary feedback to regulate the power requirement of the LEDs. Electrical capacitances
and
ensure a noise free stable DC voltage is made available to the LEDs. They also act as voltage buffers and reduce the ripple voltage across the LEDs.
A typical LED light engine usually comprises number of parallel LED strings, each consisting
number of series-connected identical LEDs. A driver converts input power
which is a product of supply current
and voltage
into
, which is the power required to light up the LEDs, the sum of individual powers of all LEDs connected in the strings. As seen in Figure , the total current
, supplied to the LEDs is the sum of currents flowing through the parallel strings which are expressed as
. Similarly, the total voltage
across a LED string is the sum of individual forward voltage
across each LED, which is expressed as
. Therefore total power consumed by all LEDs can be expressed as
.
Power conversion is subject to losses, power consumed by the drivers is always more than the actual power required to drive the LEDs. This fact can be represented in terms of driver efficiency as shown in EquationEquation (1)
(1)
(1) which the ratio of total LED power
to the supply power
. Driver efficiency is constant for a given load condition. Power consumed by the LEDs is proportional to power drawn from the supply as seen in EquationEquation (1)
(1)
(1) .
LED driver powered by supply voltage provides constant forward current
to the LEDs. Supply voltage is a constant. Hence EquationEquation (1)
(1)
(1) is rewritten as (2), wherein a linear relationship between supply current
and forward voltage
across LEDs is represented. The relationship between forward voltage
and junction temperature
is also linear, therefore supply current
is proportional to junction temperature as seen in EquationEquation (3)
(3)
(3) . Therefore, by measuring supply current
, junction temperature can be determined.
LED lighting products are categorized as integrated luminaires (LED bulbs) and non-integrated luminaires (downlights). These types of luminiares are shown in Figure . The points accessible for external measurements for each luminaire are also shown in Figure . In case of a non-integrated luminaire driver is external. For an integrated luminaire, the driver is inbuilt. In case of a non-integrated luminaire, in addition to supply current and supply voltage
, LED string forward voltage
represented as
and forward current
represented as
are also measurable as seen in Figure , whereas in the case of integrated luminaires especially LED bulbs, only supply voltage and current is measurable. A typical example of an integrated luminaire is a LED bulb as seen in Figure .
Figure 2. Examples of integrated and non-integrated LED luminaires with points available for measuring electro-thermal characteristics.

Dynamic measurement of supply current , in either case, will yield the nature of junction temperature rise when the LED luminaire is switched ON. In the case of non-integrated luminaire prior knowledge of
relationship (Ke et al., Citation2016; Petroski, Citation2015) will give the operating junction temperature of the LED luminaire using the measured
values (Fulmek et al., Citation2014; Niculina et al., Citation2016; Tetzlaff et al., Citation2016; Ye et al., Citation2013); however, the methodology needs to be devised to estimate junction temperature values from supply current transients in the case of an integrated luminaire as it is not possible to measure forward voltage
across the LED strings. This issue is discussed in detail in the upcoming sections.
3. Opto-electrical (Ø – Iin) and Opto-thermal (Ø – Tj) models of a LED luminaire
Radiant power (radiometric power) and luminous flux are the most important optical parameters for LED lighting systems although the spatial luminous intensity distribution is sometimes required (Nichia Corporation, Citation2016). LEDs radiate electrical power within the visible spectrum but the power is not uniformly radiated across the visible spectrum. Energy radiation is dependent on wavelength and is expressed as per EquationEquation (4)
(4)
(4) . A typical cool white LED bulb with correlated color temperature (CCT) in the range of 5000K–10,000K (Keeping, Citation2013; Roby & Aubé, Citation2017) along with CIE photopic spectral luminous efficiency function
is shown in Figure .
Figure 3. Spectral power distribution Φ of a typical cool white LED bulb with correlated color temperature (CCT) in the range of 5000K–10,000K along with CIE photopic spectral luminous efficiency function
is shown. Radiant power is weighted by spectral luminous efficiency function to express it photometrically.

Luminous flux is the photometric equivalent to radiant power and is expressed as per EquationEquation (5)(5)
(5) , luminous flux is a product of the wavelength-weighted average of the radiant flux in the visible spectrum multiplied by a scaling factor of 683
. The CIE photopic spectral luminous efficiency function popularly known as “Human eye sensitivity curve V(
)” is shown in Figure , is used to convert radiant power into its photometric equivalent of luminous flux, V(
) curve is not the same across the visible spectrum (Adrian, Citation2003; Schwiegerling, Citation2004).
The peak sensitivity of curve shown in Figure is at 555 nm. According to the definition for a candela, the luminous efficacy of radiation for a monochromatic source emitting its peak power at 555 nm is equal to 683
. Hence for light sources which are not monochromatic, radiant power at a given wavelength is converted to its luminous power by multiplying the scaling factor of 683
with corresponding
and radiant power values at that wavelength.
Wall plug efficiency as expressed in EquationEquation (6)
(6)
(6) , is a metric that relates radiant power output
to electrical power input
of a LED lighting system (Chen et al., Citation2012; Ching et al., Citation2012; Liu et al., Citation2017; Raypah et al., Citation2016; Yan et al., Citation2015). The efficiency of LED luminaires is also often discussed in terms of luminous efficacy of radiation
as expressed in EquationEquation (9)
(9)
(9) , it is the ratio of spectrally-weighted radiant power (luminous flux
) to radiant power
. Since luminous efficacy is dependent on spectral eye sensitivity curve
,
in comparison to
is an appropriate metric for comparing LED products.
Wall plug efficiency is a constant for given power rating and junction temperature hence EquationEquation (6)(6)
(6) is simplified to show a that radiant power
is proportional to input current
and is shown in EquationEquation (7)
(7)
(7) . Similarly from EquationEquations (3)
(3)
(3) and (Equation7
(7)
(7) ) radiant power is proportional to the junction temperature as shown in EquationEquation (8)
(8)
(8) .
Using EquationEquation (3)(3)
(3) , (Equation6
(6)
(6) ) is rewritten as,
The luminous efficacy of radiation measures the fraction of radiant power converted into visible light. It is obtained by dividing the luminous flux by the radiant flux as expressed in EquationEquation (9)
(9)
(9) . Radiant power emitted outside visible spectrum reduces luminous efficacy, because it contributes to the radiant power while the visible light is zero, this is illustrated in Figure , as seen in the illustration, wavelengths near the peak of the spectral eye sensitivity curve V(
) contribute more strongly than those near the edges. The maximum possible luminous efficacy which can theoretically be achieved at a wavelength of 555 nm is 683 lm/W. This assumption is purely hypothetical as 1W of radiant power cannot be converted without losses into visible light.
Radiant power distribution spread across the visible spectrum will lead to a drop in the theoretical maximum luminous efficacy. Hence, there is no single constant value for the maximum luminous efficacy of LED lighting products. The maximum luminous efficacy depends on the spectral distribution of the LED product (Chen et al., Citation2013; DIAL, Citation2016; Hung & Tsao, Citation2013; Lisitsyn et al., Citation2016; Murphy, Citation2012; Narukawa et al., Citation2010; Ohno, Citation2004).
For a given LED spectral power distribution, luminous efficacy is a constant, hence from EquationEquations (8)
(8)
(8) and (Equation9
(9)
(9) ), it can be concluded that luminous flux
is proportional to junction temperature, and expressed as per EquationEquation (10)
(10)
(10) ,
Luminous efficacy of a LED product can be normalized by the maximum possible luminous efficacy 683 lm/W to a dimensionless quantity called “luminous efficiency”, which is expressed as in EquationEquation (11)
(11)
(11) .
A typical cool white LED spectrum with a CCT of 5000K used in most domestic and office applications is shown in Figure (DIAL, Citation2016; Keeping, Citation2013; Roby & Aubé, Citation2017). For the LED spectrum, numerical integration of radiant power expression, is equal to 103W and numerical integration of spectrally-weighted radiant power expression,
is 50W. Therefore luminous efficiency of a typical cool white LED spectrum is approximately equal to 50% as shown in EquationEquation (12)
(12)
(12) ,
Hence, for a typical cool white LED products it can be generalized that,
Luminous efficacy of radiation
Luminous efficiency
Opto-electrical and opto-thermal characteristics of a LED luminaire can be summarized as EquationEquation (13)(13)
(13) ,
4. Optical-Electrical-Thermal (OET) model of a LED luminaire
A multi-domain model of a LED luminaire containing “N” identical LEDs mounted on a common heat sink is shown in Figure . It is assumed that the heat is distributed uniformly and the junction temperature of all the LEDs is approximately the same. Unlike the models discussed in literature (Hui & Qin, Citation2009; Hui et al., Citation2012; Poppe, Citation2015; Tao & Hui, Citation2012), in the proposed model, optical, electrical and thermal power are treated as the function of junction temperature. This model can be analyzed as electrical network by applying mesh or node analysis. Parameters described in the model need to be extracted experimentally.
Figure 4. Multi-domain model of a LED luminaire containing ‘N’ identical LEDs mounted on a common heat-sink.

Total electrical power applied to a array of “N” LEDs is the arithmetic sum of individual power requirement of each LED as seen in Figure . If all the “N” LED devices are placed on the same heat sink, the dynamic thermal equivalent model is shown in Figure (Hui, Citation2017; Lasance & Poppe, Citation2014), assuming that the temperature is uniformly distributed and the junction temperature of the “N” LEDs is same, the dynamic thermal equivalent circuit of Figure is simplified as shown in Figure .
The general expression for an increase in temperature of an object “T” is given by EquationEquation (14)(14)
(14) , where
is the heating power,
and
are the thermal resistance and time constant of the object respectively. Based on this, the thermal characteristics of a LED luminaire are modelled by making use of the junction temperature expression given in (15) (Stout, Citation2007).
It can be observed from EquationEquation (14)(14)
(14) that irrespective of circuit representation either in Figure (Hui, Citation2017), junction temperature expression remains the same and is dependent on heat sink temperature
.
Similarly, heat sink temperature is expressed as,
In this work, an optical-electrical-thermal (OET) model of a LED luminaire is proposed with the points for measuring OET parameters. The separate OET models are proposed for both non-integrated and integrated LED luminaires. A typical example for a non-integrated LED luminaire is a downlight shown in Figure , which has a separate LED driver hence multiple parameters can be measured as already seen in Figure . In an integrated LED luminaire, the driver is inbuilt as seen in Figure , the ideal example for this is a LED bulb.
To measure optical-electrical-thermal (OET) characteristics of typical LED luminaires two methods are proposed. For non-integrated luminaire multiple measurement points are available as seen in Figure , both input and output parameters of an LED driver can be measured as well as its case temperature . In addition to this luminous flux
and heat sink temperature
are also measurable. In the case of an integrated LED luminaire for example a LED bulb, only luminous flux, supply power, and, heat sink temperature are measurable. Ammeter
and voltmeter
in both cases measure supply power, in case of non-integrated LED luminaire ammeter
and voltmeter
measures driver output in terms of LED string forward current
and voltage
.
Figure 7. Multi-domain model of non-integrated LED luminaire with the points for measuring OET characteristics. are ammeters and
are voltmeters.

The proposed methods allow for real-time measurement of OET characteristics without the need for any laboratory setup. This type of measurement system paves way for an upcoming concept known as “connected lighting systems”. LED lighting will replace traditional lighting infrastructure in the years to come. LED products offer great potential for future connected lighting systems, that will become a data-acquisition and analytics platform to enable greater energy savings in buildings and cities. These connected lighting systems can not only significantly enhance the energy performance of lighting and other building systems, but also enable a wide array of prognostics and diagnostics services that would enhance the value of lighting systems. In the future, LED lighting will also be powered by a low voltage DC supply, one possible implementation is Power over Ethernet (PoE), whereby a single Ethernet cable is used to both provide low-voltage DC power and enable network communication. With this kind of arrangement LED luminaires can report their energy consumption and help to deliver increased energy savings over conventional lighting solutions by facilitating data-driven energy management and prognostics.
5. Method to estimate junction-to-ambient thermal resistance of a LED luminaire
Let us consider a junction node in LED luminaire, as seen in Figures , the junction temperature is dependent on incoming heating power
, heat sink temperature
, equivalent thermal resistance
and capacitance
. The heat sink temperature
is further dependent on interconnected RC network from junction to ambient and total power it receives from all the LEDs, this makes the analysis complex and a simpler solution needs to be found out.
Figure 8. Multi-domain model of integrated LED luminaire with the points for measuring OET characteristics. is ammeter and
is voltmeter.

Considering Figures , thermal resistance of the LED luminaire will charge all the thermal capacitances downstream of its position.The heating power that charges the thermal capacitances along the heat conduction path from the junction to the ambient must also pass through all the thermal resistances along the same path. Similarly, heating power received by the heat sink would have passed through thermal RC network of each LED before reaching the heat sink.It may be noted that because of this thermal time constant of the heat sink will be higher than that of the LED junction. The point to be noted is that, if the time constants of the leaf nodes along an interconnected RC network are determined experimentally, the RC network can be reconstructed using the experimental results and by applying the concept of Elmore delay (Lamdan, Citation1976; Sapatnekar, Citation1994).
The simplest form of performance evaluation for LED packages with or without heat sinks, is in terms of delay metrics that are available for interconnected RC networks. Elmore delay is a delay metric on which most explicit RC network approximations are based. For an RC ladder network comprising of “n” nodes as seen in Figure , the general expression for the time constant at the ‘n’th node is given by EquationEquation (17)(17)
(17) . For any input signal at a node, resistance at that node charges all the capacitors downstream of its position. This has to repeat for all the resistances along the path till the last node.
Figure 9. Thermal RC ladder network representing 1D heat conduction path from the first node (node 1 :junction) to the nth node (node n:Heat sink).

The primary disadvantage of the Elmore delay model is that it has limited accuracy, but still it provides useful insight into the dependence of interconnect delay with various parameters. Elmore delay model always overestimates the delay (Gupta et al., Citation1997), for this simpler version of Elmore delay model called the Fitted Elmore delay (FED) (Abou-Seido et al., Citation2004; Avci & Yamacli, Citation2010; Celik et al., Citation2002) is used. FED is obtained by approximating measured time delay data using the curve fitting approach. Elmore delay model is the basis for the functional form used for curve fitting in FED.
There are two time constants and
at the LED junction and heat sink are, respectively, given by EquationEquations (18)
(18)
(18) and (Equation19
(19)
(19) ). The time constant
, reflects the delay along the heat conduction path from the junction to ambient, similarly the time constant
reflects the delay from the heat sink to ambient.
In this work, the concept of FED is used to fit the junction temperature and heat sink temperature measurement data to obtain time constants of junction-to-heatsink and heatsink-to-ambient as expressed by EquationEquations (18)(18)
(18) and (Equation19
(19)
(19) ), respectively. As seen Figure , the LED junction-to-ambient thermal resistance
charges through thermal capacitance
and heat sink thermal capacitance
downstream of its position. Similarly, heat sink thermal resistance charges only through heat sink capacitance, but the delay at the heat sink includes delay at the junction. For “N” LEDs charging happens in parallel hence time constant of one LED can be considered in addition to the time constant of a heat sink. The expected plots of junction temperature, heat sink temperature, and power consumed are shown in Figure , these measurements are used to obtain FED RC models for the LED luminaire under test.
6. Discussion
The snapshot of the process explained in this work is depicted in Figure . The steps are described below in detail.
Figure 11. Process flowchart depicting the multi-domain empirical modelling of LED luminaires using real-time measurements.

The measurement set-up portrayed in Fig. or Fig. is made according to the device-under-test (DUT), whether it is either a integrated or a non-integrated luminaire.
The luminous flux
, heat-sink temperature
, supply current
and input power
are recorded from the instant of switching ON the DUT till the steady state is reached. Steady-state is reached when the difference between two readings recorded 30 minutes apart is less than 0.5%.
3. Time constants is derived from heat-sink temperature rise plot and
is obtained either from luminous flux decay plot or input current plot as both
and
are proportional to each other.
Thermal capacitance
of heat-sink is very much greater than LED device thermal capacitance
(Janicki et al., Citation2019; Poppe & Szalai, Citation2014; Tao & Hui, Citation2012); hence,
in EquationEquation (18)
(18)
(18) is simplified to
.
If heat-sink thermal resistance
is known or can be estimated approximately, then junction-to-heat-sink thermal resistance
is estimated using the two thermal constants
and
as shown in the flow chart.
If heat-sink thermal resistance
is not known or cannot be estimated approximately, then electrical thermal resistance (Rainer & Thomas, Citation2019) of heat-sink is calculated by dividing the temperature rise
of the heat-sink by applied electrical power
.
Once the junction-to-heat-sink thermal resistance
is calculated either thermally (real) or electrically, steady-state junction temperature
can be estimated using the formulas given in the flow chart.
For estimating junction temperature
using “real” thermal resistance value of
, ”Luminous efficacy of radiation” (
) of LEDs is required. Otherwise,
can be estimated using “electrical” thermal resistance of
and electrical power input, as shown in the flow chart.
The junction-to-ambient thermal resistance (
) is the most commonly reported thermal metric for semiconductor and IC packages (Edwards & Nguyen, Citation2016). Junction-to-ambient thermal resistance is a gauge of the thermal performance of a LED luminaire. The junction-to-ambient thermal resistance
is a metric, which allows comparison of relative thermal performances of different LED products. Thus, the thermal performance of a LED luminaire can be compared to a product from another company.
is used by luminaire designers to estimate junction temperatures (hottest temperature of the LED junction) of LEDs when used in their luminaires. Thermal performance assessment is required to assess whether LEDs in a luminaire are operated within the safe limits prescribed by the manufacturers. Manufacturers specify operating junction temperature limits within which the LED needs to be worked continuously for reaching the designated performance levels over their designed lifetime. The performance of the LED degrades if the device operates above recommended safe temperature limits. Sometimes the LEDs may fail to perform at high undesired junction temperatures. Hence it is very important to assess the thermal performance of a LED product to ensure designated lifetime performance levels.
6.1. Validating proposed theory using experimental results from prior studies
In an prior study (Wang, Citation2014) done at Department of Marine Engineering of National Taiwan Ocean University, temperatures were recorded using thermocouples at designated points on the surface of a LED luminaire during its operation. The luminaire had 24 LED chips mounted on a aluminum metal core printed circuit board (Al-MCPCB). The luminaire had a heat-sink made of die-casting aluminum upon which the LEDs on Al-MCPCB were mounted. The objective of (Wang, Citation2014) was to examine the thermal performance of a high power LED module and optimize heat-sink weight and its fin geometries to reduce LED junction temperature. Temperatures were recorded for different input power. Temperatures were recorded using 16 T-type thermocouples made of copper and nickel with an error range of .One of the thermocouple was attached to the LED chip surface to measure its temperature. The LED chip surface temperature is the maximum temperature that can be measured using direct measurements, because it is not possible to measure LED junction temperature directly using thermocouples (Lee et al., Citation2018). Hence, LED chip surface temperature acts as a proxy for its junction temperature. The heat-sink fin temperatures were also recorded using multiple thermocouples. One thermocouple measured the ambient temperature.
The measured junction-to-heat-sink thermal resistance was
C/W and that of heat sink
was
C/W, this includes convection resistance as well. Further in the context of the current study the temperature rise profile is required to validate the proposed theory. However, there are some drawbacks with this dataset for obtaining thermal model parameters. The limitations in the dataset highlights the accuracy required for experimentally determining LED luminaire model parameters. The temperature rise recorded at the LED chip-surface and at the heat-sink fin for the LED luminaire examined in (Wang, Citation2014) for different power ratings (16W, 20W and 24W) is shown in Figure . There are two major drawbacks in this data set that renders it unsuitable for modelling LED luminaire performance. First, the data set does not represent steady-state performance of the luminaire, because difference between the last two readings taken 10 minutes apart at 50th and 60th minute is greater than 2%. This is indicated by enclosing the end of the temperature profiles by a ellipse as shown in the Figure . Steady state is reached when the difference between any two readings measured 30 minutes apart is less than 0.5%. Second, as seen from the temperature profile LED chip surface temperature rises sharply to almost 70% of its maximum recorded value within 30 seconds of switching ON. The data set fails to capture temperature profile from 0 to 30 seconds (this shown in the figure by enclosing the temperature profile by an ellipse), as this information is crucial for determining the time constants for deriving thermal resistance and capacitance values. The time interval for data acquisition during the initial few minutes of the luminaire operation must be as small as possible preferably in seconds, so that time constants can be estimated precisely.
Figure 12. Measured electro-thermal characteristics of the LED luminaire studied in (Wang, Citation2014). The rise time of chip surface temperature is much faster compared to heat-sink fin temperature. This establishes the fact that junction temperature reaches steady state earlier than heat-sink temperature as proposed in Equationequations (18)(18)
(18) and (Equation19
(19)
(19) ). It must be noted that rise time decreases with increased electro-thermal stress.

Apart from the shortcomings in the data set, the data set is still good enough to corroborate the findings of proposed in EquationEquations (18)(18)
(18) and (Equation19
(19)
(19) ) of this study. As shown in Figure and stated in the equations, junction temperature attains steady-state faster than the heat-sink temperature. Both, LED chip surface temperature and heat-sink fin temperature rise with increasing electro-thermal stress. Moreover, for the given power ratings from 16W to 24W, the electrical thermal resistance of the heat-sink fin estimated using its temperature rise and applied electrical power was approximately between
C/W and
C/W which closer to the physically measured value of
C/W as reported in (Wang, Citation2014). From the electrical thermal resistance of the heat-sink fin, the junction-to-heat-sink thermal resistance was estimated as approximately between
C/W and
C/W for tested power ratings, which is closer to the physically measured value of approximately
C/W as reported in (Wang, Citation2014). There is a close agreement between the theory proposed in this work and the experimental results published in (Wang, Citation2014), thereby corroborating the proposals of this work.
An ensemble of studies (Baran, Leśko et al., Citation2019, Baran, Różowicz et al., Citation2019, Citation2020) on LED luminaire performance analysis, jointly done by Rzeszow University of Technology, Poland and Kielce University of Technology, Poland has been recently published. These studies present thermal modelling of LED luminaire using computational fluid dynamics (CFD) software. Simulation studies were done to assess impact of junction temperature on light parameters during actual operating conditions. Simulation studies to examine the effect of luminaire housing, heat sink geometry, printed circuit boards, and ambient conditions on the luminaire performance. For the purpose of numerical calculations using CFD software, the multi-domain characteristics and thermal parameters of the LED source were obtained using sophisticated equipment in the laboratory under standard test conditions. Simulation results published in (Baran, Leśko et al., Citation2019, Baran, Różowicz et al., Citation2019) were confirmed in (Baran et al., Citation2020) by real measurements on the fabricated LED panel prototype. An error of 5% was reported between the temperature readings obtained from simulation in (Baran, Leśko et al., Citation2019, Baran, Różowicz et al., Citation2019) and actual measurements in (Baran et al., Citation2020). The difference in lighting parameters obtained from simulation and real measurements did not exceed 5% thereby corroborating the findings of simulations.
In the context of the current work, to validate the theoretical proposals made, a brief analysis is presented about the relevance of findings reported in these prior studies (Baran, Leśko et al., Citation2019, Baran, Różowicz et al., Citation2019, Citation2020). Luminous flux and spectral properties of the LED device used in the luminaire were measured for different driving currents and junction temperatures using a integrating sphere in (Baran et al., Citation2020). The LED device was mounted on a Peltier cooler for temperature control. The relative spectral power of the LED device is seen in Figure . The “Luminous efficacy of radiation (LER - )” is estimated using EquationEquation (12)
(12)
(12) discussed earlier. LER helps in estimating heat emitted (Rainer & Thomas, Citation2019) by the LEDs. Power consumed by the LED under different driving currents and junction temperatures is shown in Figure . As discussed in EquationEquations (2)
(2)
(2) and (Equation3
(3)
(3) ), the input power is proportional to junction temperature under constant current. Hence as proposed in this work, power consumption
transient indicates junction temperature rise. Further it must be noted that luminous flux is also proportional to consumed power and junction temperature under constant current conditions. This is illustrated in Figure . As per theory proposed earlier, luminous flux transient also indicates junction temperature rise. Luminous flux and power consumed decreases with increasing junction temperature. Hence, measuring either power consumed or light emitted helps to determine the operating junction temperature.
Figure 13. Relative spectral power distribution, electro-thermal and opto-thermal characteristics of the LED source used in Baran, Leśko et al. (Citation2019, Baran, Różowicz et al., Citation2019, Citation2020). Electro-thermal characteristics represent power consumption for different forward currents at different junction temperatures. Opto-thermal characteristics represent light output for different drive currents at different junction temperatures.

A LED luminaire prototype was created for analysis purpose in Baran et al. (Citation2020). Twelve LEDs were soldered to a common MCPCB printed circuit board and mounted on common heat sink. The heat sink was made of Al5052 aluminum alloy, more information on its geometry and construction are available in Baran, Różowicz et al., (Citation2019). Heating power was calculated using EquationEquation (20)(20)
(20) .
where luminous flux emitted by the LED product,
is the LER and
is power consumed by the device. For obtaining real-time temperatures, seven K-type thermocouples were placed on the different surfaces of the LED luminaire. Two important measurement points of interest are, one on the MCPCB at a 1 mm distance from the LED device and other on the fin protrusion. The recorded temperature profile during the LED luminaire operation is recreated from (Baran et al., Citation2020) and shown in Figure . As seen from the temperature profiles the temperature of MCPCB rises relatively faster in comparison with the heat sink temperature. This is already explained theoretically and with necessary equations in Sec.5. Hence, the results of experimentation corroborate the proposed theory.
Figure 14. Measured temperatures on the MCPCB (measured closer to the LED junction) and heat-sink fin. It is observed that rise times for both MCPCB and heat-sink fin temperatures are almost same. The derived thermal model of the LED luminiare is shown. This model was obtained using the experimental and simulation data available in (Baran et al., Citation2020). The transient temperature plot and thermal model is already discussed theoretically and shown in Figure .

As discussed in the EquationEquations (18)(18)
(18) , (Equation19
(19)
(19) ) and in the flowchart shown Figure , the time constants of temperature profiles help in obtaining thermal model RC parameters. Thermal resistance values of LED junction-to-case and MCPCB were obtained through measurements in (Baran et al., Citation2020). However, the thermal resistance value of the heat sink was not mentioned. Thermal resistance for the heat sink was calculated based on its temperature rise and the total heating power emitted by all LEDs. The thermal resistance of the heat sink was calculated as
C/W. When worked backwards, the temperature rise of the MCPCB was estimated using the total heating power and its thermal resistance. The calculated value of temperature rise of MCPCB module was
C whereas the measured by thermocouples was nearly
C, thereby supporting theoretically. A thermal model for the LED luminaire is developed using the experimental results in (Baran et al., Citation2020) and shown in Figure . Based on the thermal resistance value of the LED junction-to-case and its emitted heating power, junction temperature is estimated as
C and the corresponding total lumen output calculated based on the characteristics in Figure was 3696 lumens. The luminous flux measured using real time measurements was 3570 lumens and that with simulation results was 3670 lumens. Both these lumen outputs point to a junction temperature of around 58 to
C as per (Baran et al., Citation2020).
This analysis has helped to corroborate the theory discussed in this work. It can be summarized that LED luminaire can be characterized using real-time measurements. The two minimal measurements that are required for empirical LED luminaire modelling are luminous flux decay and heat sink temperature rise. The process is explained in flowchart shown in Figure . In the absence of known thermal parameters values, coefficients can be extracted by curve fitting the transient measurements of luminous flux and heat sink temperature. As already discussed the all thermal capacitances other than that of heat sink are negligible and can be ignored in the analysis, thereby simplifying the “Elmore delay” equations. The process depicted in the flowchart is validated using the experimental results from independent prior studies.
7. Summary
LED products for general lighting purposes are choice between designing either a complete product based on LEDs or an LED-based lighting module meant to be installed into an existing luminaire. A complete new luminaire design is preferred over the retrofit lamp as the latter constrains design. It is up to the designer to choose between the total system performance of a new luminaire or the convenience of a retrofit lamp is more important in the target application. A typical LED lighting system operates in the electro-thermal domain. It comprises a constant current drive, LEDs, and a thermal management system. The dynamic thermal model of a LED luminaire comprises thermal resistances and thermal capacitances arranged in the form of an RC network. The behavior of the thermal system is analyzed in analogy with an electrical circuit.
In this study, a multi-domain representation of a LED lighting product is deliberated. Data-sheets provided by manufacturers serve to obtain opto-electrical and opto-thermal characteristics of LEDs and their products. Both transient and steady-state responses of LED products are obtained theoretically. The theoretical findings are corroborated using experimental outcomes from earlier studies. The complexities involved in creating a best LED product are discoursed and scrutinized. Earlier studies on LED luminaire analysis dealt with challenges during initial stages of product development. Most of the earlier studies have disregarded the influence of luminaire covering and optics on the heat transfer from the luminaire to the ambient. This study focuses more on “Black box” testing; one of the aims of this work is to suggest experimental methods to examine multi-domain characteristics of a LED lighting system and link it theoretically. Thermal time constants of LED products are key as they influence the rate of light output decay when luminaires are working for prolonged periods. Thermal time constants are also accountable for defining the time taken by the junction temperature to stabilize with the ambient.
Popular “Photo-Electro-Thermal (PET) theory”, extensively discussed in earlier studies suggests challenging equations for modelling transient and steady-state response of LED products. In this work, simple equations founded on the theory of “Elmore delay” are proposed to comprehend growth of junction and heat-sink temperature. Elmore delay is a proxy to the delay through an RC network in an electronic circuitry. A thermal RC network of an LED luminaire is comparable to an electric circuit. The theory of Elmore delay is representative for timing investigation and knowing light output degradation with time. Simulations and experimental results corroborate the applicability of Elmore time constants in transient and steady-state analysis of LED luminaires. Elmore delay is easy to estimate and reasonably accurate. Even where it is inaccurate, it is usually honest, in the sense that reducing Elmore delay will end in decreasing real delay, making it valuable in optimization. From this work, it can be inferred that the junction temperature rise and luminous flux decay are influenced by the product of single most dominant thermal resistance from the junction to a point along the heat conduction path and the sum of all the downstream thermal capacitances till that point. LED device thermal capacitance is very less when compared to the thermal capacitance of the heat-sink; therefore, the product of single most dominant thermal resistance and thermal capacitance of heat-sink decide the rate of rise of junction temperature or luminous flux decay.
document.bib
Download Bibliographical Database File (27 KB)cover-image-main.tex
Download Latex File (2.9 KB)fig-flowchart.tex
Download Latex File (4.3 KB)Disclosure statement
No potential conflict of interest was reported by the author(s).
Supplementary material
Supplemental data for this article can be accessed online at https://doi.org/10.1080/23311916.2023.2288423.
Additional information
Notes on contributors
Shailesh K R
Shailesh K R received B.E. degree in Electrical and Electronics engineering from Mangalore University, India, in 1997 and M.Tech. degree in illumination technology from Mangalore University, India, in 1999. He received Ph.D degree for the thesis titled ”Assessment of light-emitting diode luminaire performance using virtual test bench’’ from Manipal Academy of Higher Education, India in 2019. He is the author of more than 40 technical papers published in reputed international journals and international conference proceedings. His notable contributions include a new method of gonio-photometry known as ”Light Sieve Photometry” and a non-invasive method to measures junction temperature of LEDs in a LED luminaire. His research interests are in the area virtual instrumentation for light and light-based technologies. This work brings together the knowledge provided by LED manufacturers, academicians and software developers in creating compact multi-domain models of light-emitting diode luminaires.
References
- Abou-Seido, A. I., Nowak, B., & Chu, C. (2004, July). Fitted Elmore delay: a simple and accurate interconnect delay model. IEEE Transactions on Very Large Scale Integration (VLSI) Systems, 12(7), 691–20. https://doi.org/10.1109/TVLSI.2004.830932
- Adrian, W. (2003). Spectral sensitivity of the pupillary system. Clinical and Experimental Optometry, 86(4), 235–238. https://doi.org/10.1111/j.1444-0938.2003.tb03111.x
- Alexeev, A., Onushkin, G., Linnartz, J.-P., & Martin, G. (2019). Multiple heat source thermal modeling and transient analysis of LEDs. Energies, 12(10), 1860. https://doi.org/10.3390/en12101860
- Avci, M., & Yamacli, S. (2010). An improved Elmore delay model for vlsi interconnects. Mathematical and Computer Modelling, 51(7), 908–914. 2008 International Workshop on Scientific Computing in Electronics Engineering (WSCEE 2008) https://doi.org/10.1016/j.mcm.2009.08.024
- Baran, K., Leśko, M., Wachta, H., & Różowicz, A. (2019). Thermal modeling and simulation of high power led module. AIP Conference Proceedings, 2078(1), 020048.
- Baran, K., Różowicz, A., Wachta, H., & Różowicz, S. (2020). Modeling of selected lighting parameters of led panel. Energies, 13(14), 3583. https://doi.org/10.3390/en13143583
- Baran, K., Różowicz, A., Wachta, H., Różowicz, S., & Mazur, D. (2019). Thermal analysis of the factors influencing junction temperature of led panel sources. Energies, 12(20), 3941. https://doi.org/10.3390/en12203941
- Baureis, P. (2005, September 16). Compact modeling of electrical, thermal and optical led behavior. In Proceedings of 35th European Solid-State Device Research Conference, 2005. ESSDERC 2005. (pp. 145–148).
- Bender, V. C., Cardoso, A. S., Flores, G. C., Rech, C., and Marchesan, T. B. (2012, October 25–28). Electrothermal feedback of a led lighting system: Modeling and control. In IECON 2012 - 38th Annual Conference on IEEE Industrial Electronics Society, (pp. 4545–4550).
- Bergman, L., & McHale, J. L. (2012). Handbook of luminescent Semiconductor materials. CRC Press.
- Celik, M., Pileggi, L., & Odabasioglu, A. (2002). The Elmore Delay. Springer US.
- Chen, H. T., Tao, X. H., & Hui, S. Y. R. (2012, April). Estimation of optical power and heat-dissipation coefficient for the photo-electro-thermal theory for led systems. IEEE Transactions on Power Electronics, 27(4), 2176–2183. https://doi.org/10.1109/TPEL.2011.2165736
- Chen, Y., Zhang, M., & He, G. (2013, October). Comments on “maximum white luminous efficacy of radiation versus color rendering index and color temperature: Exact results and a useful analytic expression”. Journal of Display Technology, 9(10), 859–860. https://doi.org/10.1109/JDT.2013.2279275
- Ching, C. P., Lee, Z. Y., Lee, S. Y., and Devarajan, M. (2012, September 19–21). Analysis on optical properties for various types of light emitting diode. In 2012 10th IEEE International Conference on Semiconductor Electronics (ICSE), Kuala Lumpur, (pp. 388–391).
- Chiu, H. J., Lo, Y. K., Chen, J. T., Cheng, S. J., Lin, C. Y., & Mou, S. C. (2010, February). A high-efficiency dimmable LED driver for low-power lighting applications. IEEE Transactions on Industrial Electronics, 57(2), 735–743. https://doi.org/10.1109/TIE.2009.2027251
- DIAL. (2016, June). Efficiency of LEDs: The highest luminous efficacy of a white LED. DIALux Academy. https://www.dial.de/en-GB/projects/efficiency-of-leds-the-highest-luminous-efficacy-of-a-white-led
- Edwards, D. and Nguyen, H. (2016). Semiconductor and IC package thermal metrics. Texas Instruments Application Report.
- Farkas, G., Vader, Q. V., Poppe, A., & Bognar, G. (2005, March). Thermal investigation of high power optical devices by transient testing. IEEE Transactions on Components and Packaging Technologies, 28(1), 45–50. https://doi.org/10.1109/TCAPT.2004.843197
- Fuada, T., Adiono, S., Putra, A. P., & Aska, Y. (2017). {LED} driver design for indoor lighting and low-rate data transmission purpose. Optik - International Journal for Light and Electron Optics, 4(15), –. https://doi.org/10.4108/eai.13-12-2017.153469
- Fulmek, P., Langer, G., Wenzl, F. P., Nemitz, W., Schweitzer, S., Hoschopf, H., and Nicolics, J. (2014, May 7–11). Direct junction temperature measurement in high-power LEDs. In Proceedings of the 2014 37th International Spring Seminar on Electronics Technology, Dresden, Germany, (pp. 58–63).
- Górecki, K., & Ptak, P. (2020). Thermal, photometric and radiometric properties of multi-color LEDs situated on the common pcb. Electronics, 9(10), 1672. https://doi.org/10.3390/electronics9101672
- Górecki, K., & Ptak, P. (2021). Compact modelling of electrical, optical and thermal properties of multi-colour power LEDs operating on a common pcb. Energies, 14(5), 1286. https://doi.org/10.3390/en14051286
- Gupta, R., Tutuianu, B., & Pileggi, L. T. (1997, January). The Elmore delay as a bound for rc trees with generalized input signals. IEEE Transactions on Computer-Aided Design of Integrated Circuits and Systems, 16(1), 95–104. https://doi.org/10.1109/43.559334
- Hui, R. (2017). Photo-electro-thermal theory for LED systems: Basic theory and applications. Cambridge University Press.
- Hui, S. Y. R., Chen, H., & Tao, X. (2012, November). An extended photoelectrothermal theory for led systems: A tutorial from device characteristic to system design for general lighting. IEEE Transactions on Power Electronics, 27(11), 4571–4583. https://doi.org/10.1109/TPEL.2012.2188648
- Hui, S. Y. R., Li, S. N., Tao, X. H., Chen, W., and Ng, W. M. (2010, February 21–25). A novel passive off-line light-emitting diode (led) driver with long lifetime. In 2010 Twenty-Fifth Annual IEEE Applied Power Electronics Conference and Exposition (APEC), (pp. 594–600).
- Hui, S. Y., & Qin, Y. X. (2009, August). A general photo-electro-thermal theory for light emitting diode (LED) systems. IEEE Transactions on Power Electronics, 24(8), 1967–1976. https://doi.org/10.1109/TPEL.2009.2018100
- Hung, P.-C., & Tsao, J. Y. (2013, June). Maximum white luminous efficacy of radiation versus color rendering index and color temperature: Exact results and a useful analytic expression. Journal of Display Technology, 9(6), 405–412. https://doi.org/10.1109/JDT.2012.2224638
- Ibrahim, M. S., Fan, J., Yung, W. K., Jing, Z., Fan, X., van Driel, W., & Zhang, G. (2021, May). System level reliability assessment for high power light-emitting diode lamp based on a bayesian network method. Measurement, 176, 109191. https://doi.org/10.1016/j.measurement.2021.109191
- Janicki, M., Ptak, P., Torzewicz, T., & Górecki, K. (2020). Compact thermal modeling of modules containing multiple power LEDs. Energies, 13(12), 3130. https://doi.org/10.3390/en13123130
- Janicki, M., Torzewicz, T., Ptak, P., Raszkowski, T., Samson, A., & Górecki, K. (2019). Parametric compact thermal models of power LEDs. Energies, 12(9), 1724. https://doi.org/10.3390/en12091724
- Keeping, S. (2013, April). Defining the color characteristics of white LEDs. Digi-Key Electronics.
- Ke, H.-L., Hao, J., Tu, J.-H., Miao, P.-X., Wang, C.-Q., Cui, J.-Z., Sun, Q., & Sun, R.-T. (2018, February). Lumen degradation analysis of LED lamps based on the subsystem isolation method. Applied Optics, 57(4), 849–854. https://doi.org/10.1364/AO.57.000849
- Ke, H.-L., Jing, L., Hao, J., Gao, Q., Wang, Y., Xun Wang, X., Sun, Q., & Xu, Z.-J. (2016, July). Analysis of junction temperature and modification of luminous flux degradation for white LEDs in a thermal accelerated reliability test. Applied Optics, 55(22), 5909. https://doi.org/10.1364/AO.55.005909
- Keppens, A., Ryckaert, W. R., Deconinck, G., & Hanselaer, P. (2010). Modeling high power light-emitting diode spectra and their variation with junction temperature. Journal of Applied Physics, 108(4), 043104. https://doi.org/10.1063/1.3463411
- Ke, H. L., Sun, Q., Zhao, J., Zhang, H. X., Jing, L., Wang, Y., and Hao, J. (2016, April 18–20). Junction temperature estimation for LED lamp with forward voltage method. In 2016 17th International Conference on Thermal, Mechanical and Multi-Physics Simulation and Experiments in Microelectronics and Microsystems (EuroSimE), (pp. 1–4).
- Lamdan, T. (1976, May). Calculation of ’Elmore’ delay for RC ladder networks. Proceedings of the Institution of Electrical Engineers, 123, 411–412. https://digital-library.theiet.org/content/journals/10.1049/piee.1976.0092
- Lasance, C. J., & Poppe, A. (2014). Thermal management for LED applications. Springer-Verlag.
- Lee, D., Choi, H., Jeong, S., Jeon, C. H., Lee, D., Lim, J., Byon, C., & Choi, J. (2018, December). A study on the measurement and prediction of LED junction temperature. International Journal of Heat and Mass Transfer, 127, 1243–1252. https://doi.org/10.1016/j.ijheatmasstransfer.2018.07.091
- Li, S. S. (1993). Semiconductor physical Electronics (1 ed.). Plenum Press.
- Liang, R., Zhang, J., Wang, S., Chen, Q., Xu, L., Dai, J., & Chen, C. (2017, March). Investigation on thermal characterization of eutectic flip-chip UV-LEDs with different bonding voidage. IEEE Transactions on Electron Devices, 64(3), 1174–1179. https://doi.org/10.1109/TED.2017.2656240
- Lisitsyn, V. M., Lukash, V. S., Stepanov, S. A., & Yangyang, J. (2016). White LEDs with limit luminous efficacy. AIP Conference Proceedings, 1698(1), 060008.
- Liu, H. Y., Yang, Y. C., Liu, G. J., & Huang, R. C. (2017, December). Improved light extraction efficiency of gan-based ultraviolet light-emitting diodes by self-assembled mgo nanorod arrays. IEEE Transactions on Electron Devices, 64(12), 5006–5011. https://doi.org/10.1109/TED.2017.2766639
- Modepalli, K. and Parsa, L. (2014, March 16–20). Single stage dual purpose offline HB-LED driver with power factor correction for illumination and visible light communication. In 2014 IEEE Applied Power Electronics Conference and Exposition - APEC 2014. (pp. 1388–1393).
- Modepalli, K., & Parsa, L. (2015, January). Dual-purpose offline led driver for illumination and visible light communication. IEEE Transactions on Industry Applications, 51(1), 406–419. https://doi.org/10.1109/TIA.2014.2330066
- Murphy, T. W., Jr. (2012). Maximum spectral luminous efficacy of white light. Journal of Applied Physics, 111(10), 104909. https://doi.org/10.1063/1.4721897
- Narukawa, Y., Ichikawa, M., Sanga, D., Sano, M., & Mukai, T. (2010). White light emitting diodes with super-high luminous efficacy. Journal of Physics D: Applied Physics, 43(35), 354002. https://doi.org/10.1088/0022-3727/43/35/354002
- Nichia Corporation. (2016). Optical unit and calculation. Application Note.
- Niculina, B. D., Paul, S., and Ciprian, I. (2016, July 2). Determining the LEDs junction temperature as a function of forward voltage in given operating conditions. In 2016 8th International Conference on Electronics, Computers and Artificial Intelligence (ECAI). (pp. 1–4), June 2016. 30 June.
- Ohno, Y. (2004). Color rendering and luminous efficacy of white led spectra. In Ian T. Ferguson, N. Narendran, Teven P. DenBaars, & John C. Carrano (Eds.), Fourth International Conference on Solid State Lighting (Vol. 5530, pp. 88–98). SPIE. https://doi.org/10.1117/12.565757
- Petroski, J. (2015, March 15–19). Range and probabilities of LED junction temperature predictions based upon forward voltage population statistics. In 2015 31st Thermal Measurement, Modeling Management Symposium (SEMI-THERM). (pp. 327–331).
- Pohl, L., Hantos, G., Hegedüs, J., Németh, M., Kohári, Z., & Poppe, A. (2020). Mixed detailed and compact multi-domain modeling to describe cob LEDs. Energies, 13(16), 4051. https://doi.org/10.3390/en13164051
- Poppe, A. (2012, March 18–22). A step forward in multi-domain modeling of power LEDs. In 2012 28th Annual IEEE Semiconductor Thermal Measurement and Management Symposium (SEMI-THERM), (pp. 325–330).
- Poppe, A. (2015). Multi-domain compact modeling of LEDs: An overview of models and experimental data. Microelectronics Journal, 46(12, Part A), 1138–1151. https://doi.org/10.1016/j.mejo.2015.09.013
- Poppe, A. and Szalai, A. (2014, March 9–13). Practical aspects of implementation of a multi-domain led model. In 2014 Semiconductor Thermal Measurement and Management Symposium (SEMI-THERM). (pp. 153–158).
- Poppe, A., Zhang, Y., Wilson, J., Farkas, G., Szabo, P., Parry, J., Rencz, M., & Szekely, V. (2009, June). Thermal measurement and modeling of multi-die packages. IEEE Transactions on Components and Packaging Technologies, 32(2), 484–492. https://doi.org/10.1109/TCAPT.2008.2004578
- Rainer, H., & Thomas, Z. (2019). Package-related thermal resistance of LEDs. OSRAM Opto Application Note AN049.
- Ralston, J. M., & Lorimor, O. G. (1977, July). Degradation of bulk electroluminescent efficiency in zn, O-doped GaP LED’s. IEEE Transactions on Electron Devices, 24(7), 970–972. https://doi.org/10.1109/T-ED.1977.18862
- Raypah, M. E., Sodipo, B. K., Devarajan, M., & Sulaiman, F. (2016, January). Estimation of optical power and heat-dissipation factor of low-power SMD LED as a function of injection current and ambient temperature. IEEE Transactions on Electron Devices, 63(1), 408–413. https://doi.org/10.1109/TED.2015.2501840
- Rencz, M., Poppe, A., Kollar, E., Ress, S., & Szekely, V. (2005, March). Increasing the accuracy of structure function based thermal material parameter measurements. IEEE Transactions on Components and Packaging Technologies, 28(1), 51–57. https://doi.org/10.1109/TCAPT.2004.843204
- Roby, J., & Aubé, M. (2017, December). Lspdd: Lamp spectral power distribution database.
- Sapatnekar, S. S. (1994, June 06–10). RC interconnect optimization under the Elmore delay model. In 31st Design Automation Conference. (pp. 387–391).
- Schwiegerling, J. (2004). Field Guide to Visual and Ophthalmic Optics (pp. 12–12). SPIE Press. https://doi.org/10.1117/3.592975
- Shao, J. (2009, February 15–19). Single stage offline led driver. In 2009 Twenty-Fourth Annual IEEE Applied Power Electronics Conference and Exposition. (pp. 582–586).
- Stout, R. (2007). Linear Superposition Speeds Thermal Modeling. Power Electronics Technology, 28–33.
- Tao, X., & Hui, S. Y. R. (2012, April). Dynamic photoelectrothermal theory for light-emitting diode systems. IEEE Transactions on Industrial Electronics, 59(4), 1751–1759. https://doi.org/10.1109/TIE.2011.2109341
- Tetzlaff, T., A B, M. A., and Witkowski, U. (2016, November 28–30). Estimation of LED junction temperature based on forward voltage method for digital hardware implementation. In 2016 European Modelling Symposium (EMS). (pp. 223–228).
- Trujillo, C., Henao, G., Castro, J., & Narvaez, A. (2017, July). Design and development of a led driver prototype with a single-stage pfc and low current harmonic distortion. IEEE Latin America Transactions, 15(8), 1368–1375. https://doi.org/10.1109/TLA.2017.7994781
- van Driel, W. D., Yuan, C. A., Koh, S., & Zhang, G. Q. (2011, April 18–20). Led system reliability. In 2011 12th intl. Conf. On thermal, mechanical multi-physics simulation and experiments in microelectronics and microsystems. (pp. /1/5–5/5). Institute of Electrical and Electronics Engineers (IEEE).
- Wang, J.-C. (2014). Analyzing thermal module developments and trends in high-power LED. International Journal of Photoenergy, 2014, 1–11. https://doi.org/10.1155/2014/149520
- Williams, E. W., & Hall, R. (1978). Luminescence and the light emitting diode (1 ed.). Pergamon Press.
- Xi, Y., & Schubert, E. F. (2004). Junction–temperature measurement in gan ultraviolet light-emitting diodes using diode forward voltage method. Applied Physics Letters, 85(12), 2163–2165. https://doi.org/10.1063/1.1795351
- Yan, C., Bai, S., Nie, T., & Wang, V. (2015, November 2–4). High-power high-efficiency green LEDs. In 2015 12th China international forum on solid state lighting (SSLCHINA). (pp. 1–3). IEEE.
- Ye, H., Chen, X., van Zeijl, H., Gielen, A. W. J., & Zhang, G. (2013, September). Thermal transient effect and improved junction temperature measurement method in high-voltage light-emitting diodes. IEEE Electron Device Letters, 34(9), 1172–1174. https://doi.org/10.1109/LED.2013.2274473