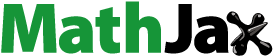
Abstract
The concrete cover is an important factor that influences the corrosion of rebar in concrete. When the specified cover depth is not achieved due to improper placement of formwork or sagging of rebars at the construction stage of RC structures, it results in a partial cover thickness. This partial cover thickness would create an electrochemical imbalance enhancing the macrocell current. This study investigated the influence of partial cover thickness, water-cement (W/C) ratio, and chloride ions on the corrosion of rebar in mortar. A special divided bar was used to make cylindrical specimens with a full cover thickness of 20 mm and partial cover thickness (20 mm and 7.5 mm) with W/C ratios of 0.30 and 0.70. Electrochemical methods such as macrocell, microcell, electric resistivity, and polarization curves were used for measurement. The corrosion current density for a partial cover thickness of 7.5 mm with a W/C of 0.30 was 3.46 μA/cm2, and for 20 mm, it was 1.85 μA/cm2. This study concludes that for a low W/C of 0.30; decreasing the cover thickness partially by 62.5% (7.5 mm) will increase the magnitude of total corrosion current density by 47%. However, for a W/C of 0.70, a partial cover thickness had no influence on the corrosion rate.
Reviewing editor:
1. Introduction
Reinforced concrete (RC) structures that are located near coastlines deteriorate prematurely, resulting in structural failure mainly due to the corrosion of steel in concrete. The service life of these structures is reduced due to corrosion, and the associated losses are an enormous burden on the economy.
The cover thickness in concrete structures serves as a first line of defense against environmental effects to protect the passive film and keep the rebar in a highly alkaline state (pH > 12 ∼ 13) (Menna Barreto et al., Citation2021) due to the cementitious matrix. The pH and electrochemical potential of the rebar both determine the electrochemical status of the rebar (Hu et al., Citation2022). The rebar may not remain in these conditions when the structures are located near the coastline, as shown in , due to the penetration of chloride ions through concrete that reaches the rebar surface and results in the destruction of the passive film (Montemor et al., Citation2003). During construction, when the allowable tolerances for the cover depth are not followed (Menna Barreto et al., Citation2018) or if chairs intended to hold rebars dislocate, then a cover with partial or varying cover thickness may be constructed. American Concrete Institute (ACI) specifies a tolerance of ±6.85 mm for a member depth upto 100 mm or less (ACI Committee, Citation2015). According to the BS EN 206-1 allowable tolerance (△c) ranges from 5 mm to 15 mm for surfaces cast against formwork and the recommended cover depth for structure exposed to chloride attack is 45 mm + △c for OPC with W/C ratio of 0.35 with an intended working life of 100 years (British Standards Institution, Citation2006). JSCE recommends allowable tolerance for cover depth based on the bar diameter in the range of ± 5 mm for stirrups and ± 20 mm for deformed bars of diameter 29 mm to 32 mm (Japan Society of Civil Engineers , Citation2007). Asamoto et al. investigated 56 construction sites in Japan, Vietnam, and Thailand and found that a 40 mm cover depth was adequate regardless of the environmental factors and age of the structures (Asamoto et al., Citation2021). Verma et al. predicted through the exponential relation of cover depth and depth of chloride ion concentration and found that in order to maintain chloride content below the threshold value, the minimum concrete cover should be more than 40 mm (Verma et al., Citation2013). Clark et al. investigated 25 sites, and at a maximum location, the specified cover depth was not achieved (Clark et al., Citation1997). The durability of RC structures throughout their service life must be maintained, and severe economic penalties cannot be tolerated when construction quality is poor and cover depth is not achieved (Ronne, Citation2005). Concrete cover thickness is one of the important factors that may delay the initiation of corrosion (Abd et al., Citation2023), such as the water-cement ratio (W/C), cement composition, cracks in concrete, relative humidity, and temperature (Lopez-Calvo et al., Citation2018).
Chloride ions penetration in the concrete surface with a partial cover thickness that results just after construction is not uniform but is high in some areas and low in others. At these locations, the cathodic and anodic areas coexist and are distributed over the surface of the steel (Mansfeld, Citation1971; Elsener, Citation2002; Nanayakkara & Kato, Citation2009; Miyazato & Otsuki, Citation2010; Miyazato & Otsuki, Citation2022), generating a microcell current. However, a macrocell current is generated when the cathodic and anodic steel areas could be clearly distinguished, and when the pH or chloride ion varies along the steel bar (Elsener, Citation2002; Nanayakkara & Kato, Citation2009; Miyazato & Otsuki, Citation2010; Miyazato & Otsuki, Citation2022). Macrocells are generated by potential variations, typically caused by gradients in the concentration of chloride ions during the penetration process. A macrocell is active steel in chloride-contaminated concrete (Gu et al., Citation2018). Due to electrochemical potential imbalance, the different concentrations of chloride ions in a single structural element activate macrocell corrosion (Nanayakkara & Kato, Citation2009). The summation of the anodic microcell and macrocell was equal to the total corrosion current density (Miyazato & Otsuki, Citation2022; Chen & Su, Citation2021). Thus, total corrosion current density measured was equivalent to the amount of steel weight loss (Miyazato & Otsuki, Citation2022). The corrosion process is initiated in the presence of oxygen and water in the form of vapors, and rust production eventually causes a radial decrease in the cross-section of the bar (Tuutti, Citation1982; Schiesl, Citation1988; Elsener, Citation2000), as shown in . In the case of chloride-induced corrosion, significant localized loss of the steel cross-section may take place prior to the development of cracks on the surface of concrete (Rodrigues et al., Citation2021). In chloride-induced corrosion of rebar embedded in mortar with a low W/C mortar of 0.30, the macrocell corrosion current was dominant; however, for a high W/C mortar of 0.70, the microcell corrosion current density was dominant (Miyazato & Otsuki, Citation2010).
Half Cell Potential (HCP) has been used by many researchers to measure the potential difference between anode and cathode (Kawahigashi et al., Citation2003; Maruya et al., Citation2007; Hussain, Citation2011; Paul & van Zijl, Citation2014). Elsener.B 2002 used half-cell potential and found that short cover and the lower conductivity of electrolyte make it easier to enhance macrocell local anode (Elsener, Citation2002). The HCP value for short cover thickness decreased by 27% and 39% as a result of increasing cover depth from 20 mm to 40 mm and 65 mm (Al-Galawi et al., Citation2016). The probability of corrosion measurements by HCP decreased by 50%, when the constant short cover thickness was increased from 10 mm to 60 mm (Kim et al., Citation2014). Zhou et al., Citation2015 investigated the corrosion potential of three different cover depths of 25 mm, 45 mm, and 65 mm constant short cover thickness and found that reinforcement corrosion rate decreases with an increase in cover depth. As stated in the aforementioned and other investigations, it has been concluded that the penetration of chloride ions in concrete is faster when the thickness of the cover is constantly less throughout the cross-section of the specimen, and the corrosion rate of the reinforcement increases with a decrease in the thickness of the cover. However, the influence of partial thickness of cover along the x-section on the corrosion of rebar and permeability of material, resulting from poor construction quality still remains unclear.
Therefore, In the present study, the influence of partial cover thickness and water-cement ratio on steel corrosion in a chloride environment was investigated. To this effect, the macrocell, the microcell, integrated corrosion current density, polarization curves, and electric resistivity were measured at the age of 91 days for partial cover thickness and full cover depth samples. A unique point in this study is the quantitative measurement of the corrosion current density of partial cover thickness using the special divided bar, making it possible to quantitatively evaluate the impact of construction defects and quality control.
2. Material and methods
All the sections included in this manuscript are illustrated as per standard writing procedures (Shukla, Citation2022).
2.1. Materials
2.1.1. Raw materials
All mortar mixes were made with ordinary Portland cement (OPC) (JIS R 5210), which has a specific surface area of 3290 cm2/g and a density of 3.16 g/cm3. For the fine aggregate, natural land sand with a maximum particle size of 5 mm was used with a fineness modulus of 3.22, water absorption of 2.23%, and relative density of 2.52. Chloride ions of 15 kg/m3 were added by adding sodium chloride (NaCl) to the water used for making the mortar.
2.1.2. Mix proportions
Mortar mixes were used instead of concrete to obtain a more homogeneous material to avoid the heterogeneities caused by coarse aggregates at the steel and concrete interface. As shown in , in total, four mixes were used in the study, including the control mixes with water-cement ratios of 0.30 (0.3CM) and 0.70 (0.70CM). In considering a W/C ratio of 0.70, it was intended to reflect the fact that during the last 50 years, bridges constructed in Japan have had a W/C ratio ranging from 0.35 ∼ 0.65. All four mixes were different from each other based on the quantity of cement and chloride ion concentration. The other two 0.30CM15 and 0.70CM15 consist of 15 kg/m3 added to the required quantity of water at the mixing time. Superplasticizers were added to both the control mix of 0.30CM and 0.30CM15 at a rate of 1% by weight of the cement. The sand-to-cement ratio (S/C) for types 0.3CM and 0.30CM15 was 2.0; however, for types 0.70CM and 0.70CM15, it was 2.5. The mix proportions for the mixes are illustrated in . The target and measured compressive strength of the control mortar mixes are shown in at the age of 91 days as per ASTM C39/JIS A 1108.
Table 1. Mortar composition used in the study.
2.1.3. Specimen preparation
In order to measure the localized corrosion current densities in the rebars, a deformed steel bar (SD295 in JIS G 3112) D10 mm was cut into six small-divided bars, each 25 mm in length. The lead wires were soldered at both ends of each rebar element. The soldered part of each rebar element was protected with epoxy resin. A 180 mm long bar was fabricated by joining six steel elements together with high-insulating capacity epoxy resin, as shown in . According to Miyazato and Otsuki (Citation2010), the overall resistance of the steel bars was measured to be less than 0.5 Ω. A Ø 50 mm polyvinyl chloride (PVC) circular pipe 100 m in length was cut into two pieces, and a 180 mm long divided steel bar was placed in the center of the mold. The dimensions of the upper part of the mold were Ø 25 mm, and the length was 100 mm. Details of the dimensions of the specimens and locations of the bars are shown in . Both sections of the mold consist of D10 mm bars, so the molds are prepared to ensure 20 mm cover and 7.5 mm cover depth of mortar surrounded by deformed steel bars for each section.
2.1.4. Mortar mixing
A hovert mixer was used to mix the ingredients of the mortar specimens in conformity with JIS R-5201 standards. The required amount of NaCl was first dissolved in the water and then added to the cement and sand mixture in the bowl for 0.30CM15 and 0.70CM15. The mixing was done in batches depending on the mortar mix type and number of specimens for each tested specimen.
2.1.5. Tested specimens
The tested specimens were divided into categories based on chloride ion concentration, cover depth, and mortar quality. 1-A/1-B represents a partial cover thickness specimen prepared with a W/C of 0.30/0.70. The bottom part of these specimens was prepared with a control mix of 0.30CM/0.70CM and 20 mm cover depth, whereas the top part was prepared with a 7.5 mm cover depth and mortar mix of 0.30CM15/0.30CM15. 2-A/2-B represents a full cover thickness specimen prepared with W/C of 0.30/0.70. The cover thicknees of 20 mm was constant throughout the specimen. The bottom part was prepared with a control mix of 0.30CM/0.70CM, whereas the top part was prepared with a mortar mix of 0.30CM15/0.70CM15. The details of different mortar mixtures and specimens are shown in . and show the actual structure where full cover thickness and partial cover thickness are subjected to the chloride environment, respectively. However, represents 2-A and 2-B, while represents 1-A and 1-B. The purpose of partial cover thickness samples was to simulate the actual situation of partial cover thickness, which arises due to poor workmanship at the construction stage. In , due to the partial cover thickness, chloride ions easily permeate, so only the right half of the mortar has chloride ions. In , similar chloride ion concentration differences are set for comparison with . Here, presents a characteristic specimen of this study.
Figure 3. (a) Structural element with full cover thickness. (b) 2-A and 2-B with full cover thickness of 20 mm; without and with 15 kg/m3 Cl− ions.

Figure 4. (a) Structural element with partial cover thickness. (b) 1-A and 1-B with the partial cover thickness of 20 mm and 7.5 mm; without and with 15 kg/m3 Cl− ions.

Table 2. Mortar mixes and specimen details
2.1.6. Casting and curing
After mixing, the fresh mortar 0.30 CM/0.70 CM was cast into the lower portion (the bottom part of the mold with a diameter of 50 mm and a length of 100 mm). The mold was filled in two steps. Three additional standard Ø50 x 100 mm cylindrical specimens were cast each to measure the mortar's electrical resistivity and compressive strength from the same batch. All the casted specimens with and without steel bars were covered with polyethylene sheets to prevent loss of moisture and then kept for 24 hours at controlled room temperature (20 °C). On the next day within the next 24 hours, the upper portion of the mold with a diameter of 25 mm and a length of 100 mm was filled with fresh mortar 0.30CM15/0.70CM15. Epoxy was applied to the specimen's top and bottom after demolding to prevent oxygen penetration. However, oxygen could only penetrate through the exposed surfaces. The specimens were cured at room temperature (20 °C ± 2 °C) and a relative humidity of more than 90% for 90 days (Emad et al., Citation2022). In total, 6 specimens with special divided bars and 12 specimens without steel were casted.
2.2. Method of Measurement
2.2.1. Corrosion current density
In this study macrocell and microcell corrosion current density was measured for all the steel elements in and by quantitative corrosion current measurement methods in sections 2.2.1.1 and 2.2.1.2.
2.2.1.1. Macrocell corrosion current density
The zero-resistance ammeter (ZRA) was connected to the adjacent steel elements, of the specimens, and the electric current was measured to determine the macrocell corrosion current (Nanayakkara & Kato, Citation2009; Miyazato & Otsuki, Citation2010; Miyazato & Otsuki, Citation2022; Otsuki et al., Citation2009). The term ‘macrocell corrosion current density refers to the flow of current between steel components. In the experimental setup is shown. The current that passed through the ends of the elements of steel was summed up. Hence, the sum of the electric current at the ends of the steel element under consideration divided by the surface area of that specific element is expressed in EquationEq. (1)(1)
(1) and termed the macrocell corrosion current density of the individual steel element.
(1)
(1)
where
is the surface area of the steel element-
(cm2),
is the macrocell corrosion current for the adjacent steel elements from
(
) and
is the macrocell corrosion current density of the steel element
A corrosion cell that consists of spatially located anode and cathode along the length of the special divided bar is macrocell current. The positive macrocell corrosion current density refers to the anodic macrocell (Fe→ Fe2++2e−); however, the negative macrocell current from the ZRA is termed the cathodic macrocell (O2 + 2H2O + 4e−→4OH−).
2.2.1.2. Microcell corrosion current density
In this study, the current flowing in the individual steel element is termed the microcell corrosion current. The polarization resistance derives the microcell corrosion current density (Stern & Geary, Citation1957; Andrade & González, Citation1978; Kobayashi & Miyagawa, Citation2001; Itagaki, Citation2012; Katayama, Citation2014; Paul & Adewumi, Citation2018; Andrade, Citation2019; Suryanto et al., Citation2020; Tsuru et al., Citation1979). The experimental setup is shown in . The lead wire between the adjacent steel elements was disconnected. The AC impedance method by using a frequency response analyzer (FRA); the polarization resistance on each steel element 1,2,3,4,5 and 6 of all the specimens in was measured. The target rebar element inside the mortar was surrounded by a stainless-steel plate wrapped with wet tissue (for electrolytic conditions). The amplitude of the voltage was 50 mV peak to peak with a frequency range of 5 kHz to 5 mHz. Nyquist diagram and Bode diagram were used for the calculation of polarization resistance (). The microcell corrosion current density is expressed by the equation below:
(2)
(2)
where
is the polarization resistance (Ω.cm2), the value of K is constant (0.0209 V), as proposed by Tsuru et al. (Tsuru et al., Citation1979), and
is the microcell corrosion current density of the steel element,
2.2.1.3. Maximum total corrosion current density
The total corrosion current density was calculated for each steel element of all the specimens by summation of the anodic (positive) macrocell corrosion current density measured in section 2.2.1.1 and the microcell corrosion current density measured for each element (Miyazato & Otsuki, Citation2022; Chen & Su, Citation2021) in section 2.2.1.2. There were six small steel rebars termed elements in each specimen. For specimens in , the maximum value of the total corrosion current density was evaluated.
2.2.2. Electric resistivity of mortar
Ø 50 x 100 mm cylinders casted for all types of mortar illustrated in were tested at 91 d for mortar resistivity (k.Ω/m). Stainless steel plates of 50 mm diameter were soldered with the wires of the resistor. The top and bottom of the specimen were covered with wet tissue for electrolytic conditions. An electrical circuit was formed between the ends of the cylindrical mortar specimen. As the numerical value continued to change after the connection, the average value of the fourth and fifth minutes was considered the resistance of the mortar. As all the specimens had identical surface areas, for the sake of comparison, the electrical resistivity of mortar was expressed in kΩ. The test results of three identical specimens were averaged.
2.2.3. Anodic and cathodic polarization curves
The susceptibility to localized corrosion on different iron alloys in a chlorine atmosphere was assessed using the ASTM standard G61. A three-electrode system was used to plot anodic and cathodic polarization curves. Ag/AgCl was used as a Reference electrode as shown in (ASTM G61-86,86, Citation2014). A fundamental and diagnostic technique to assess the corrosion behaviour of steel elements in mortar is a graph of the potential of rebar (mV) versus current density. shows the schematic setup of the apparatus used for measuring the polarization curve. The target rebar element inside the mortar is surrounded by a stainless-steel plate wrapped with wet tissue (for electrolytic conditions). The anodic and cathodic polarization curves were measured by connecting the lead wires of the specific rebar element, the opposite electrode plate, and the reference electrode to a computer-controlled Potentiostat (Miyazato & Otsuki, Citation2010). Potential at a rate of 1 mV/sec was applied from natural potential to ± 600 mV. The potential and the corresponding current density for all the six steel elements in each specimen were measured alternately and the anodic and cathodic polarization curves were drawn for all the elements. The 5th element of each specimen were compared in order to assess the condition of the passive film and the cathodic reaction.
3. Results and discussions
3.1. Effect of mortar quality
To investigate the effect of mortar quality, the critical specimens with a partial cover thickness was examined. The results of the macrocell corrosion current, microcell corrosion current, and total corrosion current densities of 1-A and 1-B were compared. Three specimens for each 1-A for a W/C of 0.30 and 1-B for a W/C of 0.70 were tested. The macrocell corrosion current, microcell corrosion current, and total corrosion current densities of the specimen with the highest total corrosion current value among the three specimens are presented in . In the case of a W/C of 0.30, the value of the macrocell corrosion current was 2.98 µA/cm2, and the microcell corrosion current was 0.48 µA/cm2, as shown in . However, unlike 0.30, the value of the macrocell current for W/C 0.70 was 1.80 µA/cm2, and the microcell current was 2.69 µA/cm2, as shown in . Macrocells were dominant at a lower W/C of 0.30, whereas microcells were dominant at a higher W/C of 0.70 (Nanayakkara & Kato, Citation2009; Miyazato & Otsuki, Citation2010; Otsuki et al., 2009).
Figure 8. (a) Variation of corrosion current densities for 1-A. (b) Variation of corrosion current densities for 1-B.

The difference between the maximum corrosion current density shown in for 1-A and 1-B was only 22%. Therefore, it was confirmed that in 1-B the higher corrosion current density was due to its porous matrix when subjected to similar values of chloride ions. However, contrary, to the previous studies (Kim et al., Citation2014) it has been concluded that even for a low W/C of 0.30 the current density was higher than 1 µA/cm2 which is a threshold for high corrosion rate as per Rilem recommendations (Andrade & Alonso, Citation2004). Hence, it can be concluded that for partial cover thickness, the corrosion rate will definitely be higher than for full cover thickness. The samples were broken after 91 days, and rebar elements can be visualized in . For clarity of the visualization, pictures were taken on both sides of the rebar elements. Furthermore, it can be confirmed from the graphs of that steel elements no. 4, 5 and 6 are more corroded than elements no. 1, 2, and 3; as the cover depth varies, the corrosion rate increases. Therefore, it is verified that the electrochemical corrosion current measurement results are similar to the actual corrosion situation.
shows the electrical resistivity of mortar for different mixtures with and without chloride ions for mixes with W/C ratios of 0.30 and 0.70. It was observed that the resistivity of mortar with a W/C of 0.30 and without chloride ions was highest among all other types of mixes. Resistivity is a property depending on the concrete porous system and its degree of moisture, and then from its values in saturated conditions. The diffusivity and concrete resistivity are related to the mortar microstructure. The relatively high resistivity of the mortars, as shown in , depicts their high resistance to corrosion (Andrade & Alonso, Citation2004). The durability of mortar in terms of the content of water and diffusion of ions could be indicated by the resistivity of mortar. Furthermore, the distribution of current in the mortar is influenced by its resistivity (Rodrigues et al., Citation2021). Therefore, the resistivity of mortar or concrete is an important factor to be determined for assessing corrosion rate. The resistivity of the mortar/concrete can be used as a performance parameter (corrosion indicator) (Andrade & d’Andrea, Citation2010). The mortar with high quality was more resistive to corrosion compared with the mortar with poor quality of W/C of 0.70 with a value of electrical resistivity.
The results of the anodic and cathodic polarization curves are represented in and , respectively. shows the results of the anodic polarization curves for element no. 5 in 1-A and 1-B. As seen from these polarization curves, the tendency of the curve towards the left means that the anodic and cathodic reactions are less likely to proceed. That is, the passivation film is not destroyed, and the amount of oxygen supply is small. These facts can be used to consider whether the corrosion rate was higher or smaller. In both type of specimens, the cover depth was 7.5 mm, which was too small, and the chloride ion content of 15 kg/m3 was higher, so the passive film was broken and more oxygen penetrated through the surface and reached the rebar in both specimens. This confirms the influence of sectional property that was cover thickness in this case over material property which is the strength of the mortar.
3.2. Effect of cover thickness
To investigate the influence of a partial cover thickness, the results of the macrocell, microcell and total corrosion current densities for 1-A and 2-A were compared for a W/C of 0.30, whereas for a W/C of 0.70, 1-B and 2-B were compared. The results of four different scenarios can be seen in . Here, show the variation in the macrocell, microcell and total corrosion current densities along the steel elements for full cover depth, 2-A and 2-B, respectively. It is evident from that specimens with partial cover thickness (1-A and 1-B) has a higher value of maximum current density compared with specimens with a full cover thickness (2-A and 2-B). However, the decrease in the maximum current density of the mortar with a W/C of 0.30 was greater than that of the specimen with a W/C of 0.70. Moreover, it can also be observed that in the specimens with low W/C of 0.30 mortar, the value of the total corrosion current density significantly decreases with cover depth from 3.46 µA/cm2 to 1.85 µA/cm2, which is approximately 47%. In the case of 1-B and 2-B for a W/C of 0.70, the change in the maximum current density is only 1.12%, which is negligible. Hence, in the case of a W/C of 0.70, the cover depth has no influence on the rate of corrosion of the rebar.
Figure 14. Effect of full cover thickness (2-A & 2-B) and partial cover thickness (1-A & 1-B) on total corrosion current density w.r.t W/C of 0.30 and 0.70, respectively.

Figure 15. (a) Variation of corrosion current densities for 2-A. (b) Variation of corrosion current densities for 2-B.

Similarly, the rebar elements that were broken after 91 days can be visualized in . It can be confirmed from the graphs in and and the pictures in and that element no. 5 of the partial cover thickness is more corroded than elements no. 4 and 6 of the full cover depths. However, for W/C of 0.70, the corrosion rates for both the partial cover thickness and full cover depth in the graphs of and pictures of and are almost the same. Hence, after visual observations and the value of maximum corrosion current densities of steel elements, it is confirmed that for a low W/C of 0.30, the cover depth significantly affects the rate of corrosion.
shows the comparison of the anodic polarization curve of steel element no. 5 embedded in the upper part of specimens 1-A, 1-B and 2-A and 2-B. It was observed that among all the specimens, 2-A with a full cover thickness of 20 mm and W/C of 0.30 was the best performing specimen against corrosion when subjected to chloride ions. The anodic polarization curves determine the status of the passive film. The current density for W/C 0.30 at every potential increased in the order of 1-A > 2-A. This confirms the effect of cover thickness on the corrosion of rebars for low W/C mortar. While in the case of W/C 0.70; both the anodic polarization curves of 1-B and 2-B have almost the same slope. It is pertinent to mention that due to partial cover thickness, in 1-A with W/C 0.30, the anodic curve is the same as 1-B with high W/C 0.70. Hence, concluded that the material property of the mortar has been suppressed by the sectional property that is thickness of the cover in this study. Similarly, the cathodic polarization curve can be seen in . It shows the penetration of O2 that facilitates cathodic reaction due to high relative humidity and matrix of the mortar. The tendency of the pattern of polarization curves of W/C 0.70 may be due to the porous matrix of the weak mortar. However, in the case of W/C 0.30, the effect of the cover depth can be clearly confirmed from the polarization curves of steel embedded in 1-A and 2-A.
3.3. Discussion on permeability of oxygen in partial cover thickness specimens
shows a graphical explanation and comparison of an adequate or full enough cover depth of 20 mm and a partial cover thickness of 7.5 mm. For a low W/C of 0.30, the value of the total current density of 2-A was 47% less than that of 1-A for the same amount of chloride ions due to a full cover thickness of 20 mm against a partial cover thickness of 7.5 mm, as shown in . The only reason for this reduction in corrosion rate was the partial cover thickness and dense matrix of the low W/C mortar that resisted the penetration of O2. Hence, for a low W/C of 0.30, the partial cover thickness significantly influences the corrosion rate. As stated earlier, macrocells are dominant in low W/C mortar, and 86% of the corrosion current density was part of the macrocell. Due to full cover thickness, this value decreased by 45-50%. As macrocells are more dangerous, causing pitting of steel bars (Miyazato & Otsuki, Citation2010; Miyazato & Otsuki, Citation2022; Bohni, Citation2005; Aslam et al., Citation2023) was effectively reduced by half. For the high W/C mortar of 0.70, the total corrosion current density for both 2-B and 1-B was almost the same with very little difference, as shown in and . The reason may be the penetration of O2 through the porous matrix of the high W/C 0.70 mortar. The corrosion rate increased in the following order for all the specimens; 1-B∼2-B > 1-A > 2-A.
4. Conclusions
In this study, the influence of partial cover thickness on the rate of corrosion of a special divided bar in a chloride environment was measured by applying quantitative corrosion measurement methods; macrocell current density, microcell current density, Polarization curves, and Electric resistivity for mortar with W/C ratios of 0.30 and 0.70. Based on the experimental results of the electrochemical methods the following conclusions were drawn;
For a low W/C mortar of 0.30 (1-A), the corrosion current density for partial cover thickness of (20 mm and 7.5 mm) is 47% greater than that of a full cover specimen of 20 mm (2-A); indicating the effect of cover depth on current density. Higher magnitude of macrocells are formed in 1-A.
For a high W/C mortar of 0.70, the total current density is the same for partial cover thickness and full cover thickness. Hence, there is no influence of partial cover thickness for high W/C mortar.
The total current density for 1-A (W/C = 0.30) is high contrary to previous studies that low W/C mortar has a low corrosion rate and high W/C mortar has a high corrosion rate, indicating the effect of cover thickness and quality control on the corrosion rate. The only difference is 22% in the current density of 1-A and 1-B, for both specimens the current density is higher than 1µA/cm2.
The order (1A >2-A and 1-B∼2-B) of the magnitude of the corrosion current density in the cathodic polarization curves confirms the increase in current density at every potential due to partial cover thickness. An increased current density represents a high corrosion rate.
Based on the result of this experimental study, the partial cover thickness enhances macrocells and causes pitting of steel, and spalling of concrete, which will eventually affect the performance of RC structures. Hence, care should be taken during the construction of RC structures near the coastline despite the high strength of concrete.
This research work was limited to mortar specimens as a basic study for concrete, however, keeping in view, the significant results of this study and the RC structures exposed to different circumstances, further studies can be carried out based on field testing.
Disclosure statement
No potential conflict of interest was reported by the author(s).
Data availability statement
All the data will be available upon request.
Additional information
Funding
Notes on contributors
Muhammad Afaq Khalid
Muhammad Afaq Khalid earned his bachelor’s degree in civil engineering and Master of Science in Structural Engineering from the University of Engineering & Technology, Peshawar, Pakistan. He is currently pursuing his doctoral studies at Kanazawa Institute of Technology, Nonoichi, Japan, focusing on research Concrete Durability and infrastructure maintenance management.
Miyazato Shinichi
Miyazato Shinichi received his B.S. in Civil Engineering from the Tokyo Institute of Technology, Tokyo, Japan, in 1994, and his PhD from the Tokyo Institute of Technology, Tokyo, Japan, in 1998. He is a Professor in the Department of Civil and environmental engineering, Kanazawa Institute of Technology, Ishikawa, Japan, where he is carrying out research on the Social infrastructure, Civil engineering materials and maintenance management.
References
- Abd, S. M., Mhaimeed, I. S., Tayeh, B. A., Najm, H. M., & Qaidi, S. (2023). Investigation of the use of textile carbon yarns as sustainable shear reinforcement in concrete beams. Case Studies in Construction Materials, 18, e01765. https://doi.org/10.1016/j.cscm.2022.e01765
- ACI Committee. (2015). Specifications for tolerances for concrete construction and materials and commentary. American Concrete Institute (ACI 117-10).
- Al-Galawi, NM, Al-Tameemi, AA, & Al-Jarrah, SH (2016). Effect of age and concrete cover thickness on steel reinforcement corrosion at splash zone in reinforced concrete hydraulic structures. International Journal of Scientific & Technology Research, 5(9), 129–133.
- Andrade, C. (2019). Propagation of reinforcement corrosion: principles, testing and modelling. Materials and Structures, 52(1), 2. https://doi.org/10.1617/s11527-018-1301-1
- Andrade, C., & Alonso, C. (2004). Test methods for on-site corrosion rate measurement of steel reinforcement in concrete by means of the polarization resistance method. Materials and Structures, 37(9), 623–643. https://doi.org/10.1007/BF02483292
- Andrade, C., & d’Andrea, R. (2010). Electrical resistivity as microstructural parameter for the modelling of service life of reinforced concrete structures [Paper presentation]. 2nd International Symposium on Service Life Design for Infrastructure (pp. 379–388).
- Andrade, C., & González, J. A. (1978). Quantitative measurements of corrosion rate of reinforcing steels embedded in concrete using polarization resistance measurements. Materials and Corrosion, 29(8), 515–519. https://doi.org/10.1002/maco.19780290804
- Asamoto, S., Sato, J., Okazaki, S., Chun, P. J., Sahamitmongkol, R., & Nguyen, G. H. (2021). The cover depth effect on corrosion-induced deterioration of reinforced concrete focusing on water penetration: Field survey and laboratory study. Materials, 14(13), 3478. https://doi.org/10.3390/ma14133478
- Aslam, F., Zaid, O., Althoey, F., Alyami, SH, Qaidi, SM, de Prado Gil, J., & Martínez‐García, R. (2023). Evaluating the influence of fly ash and glass waste on the characteristics of coconut fibers reinforced concrete. Structural Concrete, 24(2), 2440–2459. https://doi.org/10.1002/suco.202200183
- ASTM G61-86. (2014) Standard test method for conducting cyclic potentiodynamic polarization measurements for localized corrosion susceptibility of iron-, nickel-, or cobalt-based alloys. ASTM International.
- Bohni, H., (2005). Corrosion in reinforced concrete structures (pp. 75–76). England, UK: CRC Press LLC.
- British Standards Institution. (2006). Concrete–complementary British Standard to BS EN 206-1: Specification for Constituent Materials and Concrete. England, UK: BSI.
- Chen, L., & Su, R. K. L. (2021). Corrosion rate measurement by using polarization resistance method for microcell and macrocell corrosion: Theoretical analysis and experimental work with simulated concrete pore solution. Construction and Building Materials, 267, 121003. https://doi.org/10.1016/j.conbuildmat.2020.121003
- Clark, L.A., Shammas-Toma, M.G.K., Seymour, D.E., Pallett, P.F., Marsh, B.K., 1997. How can we get the cover we need? Structural Engineering, 75, 289–296.
- Elsener, B. (2000). Corrosion of steel in concrete. Corrosion and Environmental Degradation, 2, 389–436.
- Elsener, B. (2002). Macrocell corrosion of steel in concrete–implications for corrosion monitoring. Cement and Concrete Composites, 24(1), 65–72. https://doi.org/10.1016/S0958-9465(01)00027-0
- Emad, W., Mohammed, A. S., Kurda, R., Ghafor, K., Cavaleri, L., Qaidi, S. M. A., Hassan, A. M. T., & Asterics, P. G. (2022). Prediction of concrete materials compressive strength using surrogate models. Structures (46),1243–1267.
- Gu, X., Dong, Z., & Jin, Z. (2018). Macrocell corrosion between crossed steel rebars embedded in concrete under chloride environments. In MATEC Web of Conferences (Vol. 199, p. 04005). EDP Sciences. https://doi.org/10.1051/matecconf/201819904005
- Hu, J. Y., Zhang, S. S., Chen, E., & Li, W. G. (2022). A review on corrosion detection and protection of existing reinforced concrete (RC) structures. Construction and Building Materials, 325, 126718. https://doi.org/10.1016/j.conbuildmat.2022.126718
- Hussain, R. R. (2011). Electrochemical experimental measurement of macrocell corrosion half-cell potential replicating the re-corrosion of actual refurbished works in RC structures. International Journal of Electrochemical Science, 6(1), 199–205. https://doi.org/10.1016/S1452-3981(23)14986-8
- Itagaki, M. (2012). Principle and analytical method of impedance spectroscopy. Journal of the surface science society of Japan, 33(2), 64–68. (in Japanese).
- Japan Society of Civil Engineers (2007). Standard specifications for concrete structures “Material and construction”. (JSCE).
- Katayama H., (2014). Surface and interfacial analysis using electrochemical impedance measurement. Journal of Japan Institute of Metals and Materials, 78(11), 419–425. (in Japanese). https://doi.org/10.2320/jinstmet.JB201402
- Kawahigashi, T., Kobayashi, K., & miyagawa, T. (2003). A study of macro-cell and micro-cell corrosion of steel in concrete. Doboku Gakkai Ronbunshu, 2003(732), 1–15. https://doi.org/10.2208/jscej.2003.732_1
- Kim, Y. Y., Kim, J. M., Bang, J. W., & Kwon, S. J. (2014). Effect of cover depth, w/c ratio, and crack width on half cell potential in cracked concrete exposed to salt sprayed condition. Construction and Building Materials, 54, 636–645. https://doi.org/10.1016/j.conbuildmat.2014.01.009
- Kobayashi, K., & Miyagawa, T. (2001). Study on estimation of corrosion rate of reinforcing steel in concrete by measuring polarization resistance. Doboku Gakkai Ronbunshu, 669, 173–186. (in Japanese).
- Lopez-Calvo, H. Z., Montes-García, P., Jiménez-Quero, V. G., Gómez-Barranco, H., Bremner, T. W., and Thomas, M. D. A., (2018). Influence of crack width, cover depth and concrete quality on corrosion of steel in HPC containing corrosion inhibiting admixtures and fly ash. Cement and Concrete Composite, 88, 200–210 https://doi.org/10.1016/j.cemconcomp.2018.01.016
- M. F. F. Menna Barreto, J. F. G. Timm, A. Passuello, D. C. C. Dal Molin, and J. R. Masuero (2021). Life cycle costs and impacts of massive slabs with varying concrete cover, Cleaner Engineering and Technology, 5, 10256.
- Mansfeld, F. (1971). Area relationships in galvanic corrosion. Corrosion, 27(10), 436–442. https://doi.org/10.5006/0010-9312-27.10.436
- Menna Barreto, M. F. F., Maran, A. P., Dal Molin, D. C. C., Masuero, J.R., (2018). Cover to steel in reinforced concrete structures and their spacers. In Concrete cover thickness and plastic spacers (1st ed.). LAP LAMBERT Academic Publishing.
- Miyazato, S., & Otsuki, N. (2010). Steel corrosion induced by chloride or carbonation in mortar with bending cracks or joints. Journal of Advanced Concrete Technology, 8(2), 135–144. https://doi.org/10.3151/jact.8.135
- Miyazato, S., & Otsuki, N. (2022). Measurement method for macrocell corrosion in concrete specimen using a segmented steel bar. Journal of Advanced Concrete Technology, 20(3), 222–235. https://doi.org/10.3151/jact.20.222
- Montemor, M. F., Simoes, A. M. P., & Ferreira, M. G. S. (2003). Chloride-induced corrosion on reinforcing steel: From the fundamentals to the monitoring techniques. Cement and Concrete Composites, 25(4-5), 491–502. https://doi.org/10.1016/S0958-9465(02)00089-6
- Nanayakkara, O., & Kato, Y. (2009). Macro-cell corrosion in reinforcement of concrete under non-homogeneous chloride environment. Journal of Advanced Concrete Technology, 7(1), 31–40. https://doi.org/10.3151/jact.7.31
- Otsuki, N., Madlangbayan, M. S., Nishida, T., Saito, T., & Baccay, M. A. (2009). Temperature dependency of chloride induced corrosion in concrete. Journal of Advanced Concrete Technology, 7(1), 41–50. https://doi.org/10.3151/jact.7.41
- P. D. Ronne (2005), “Variation in cover to reinforcement: Local and international trends,” Concrete Beton, 111, 7–13.
- Paul, S. C, & Adewumi, J. B. (2018). A review on reinforcement corrosion mechanism and measurement methods in concrete. Civil Engineering Research Journal, 5(3), 80–90.
- Paul, S. C., & van Zijl, G. P. (2014). Crack formation and chloride induced corrosion in reinforced strain hardening cement-based composite (R/SHCC). Journal of Advanced Concrete Technology, 12(9), 340–351.
- Rodrigues, R., Gaboreau, S., Gance, J., Ignatiadis, I., & Betelu, S. (2021). Reinforced concrete structures: A review of corrosion mechanisms and advances in electrical methods for corrosion monitoring. Construction and Building Materials, 269, 121240. https://doi.org/10.1016/j.conbuildmat.2020.121240
- Schiesl, P. (1988). RILEM; Technical Committee 60-CSC: Corrosion of Steel in Concrete.
- Shukla, S. K. (2022). Seven research mantras: A short guide for researchers. International Journal of Geosynthetics and Ground Engineering, 8(6), 75. https://doi.org/10.1007/s40891-022-00419-6
- Stern, M., & Geary, A. L. (1957). Electrochemical polarization: I. A theoretical analysis of the shape of polarization curves. Journal of the Electrochemical Society, 104(1), 56. https://doi.org/10.1149/1.2428496
- Suryanto, B., Kim, J., McCarter, W. J., Starrs, G., & Aitken, M. W. (2020). Assessing the performance and transport properties of concrete using electrical property measurements. Journal of Advanced Concrete Technology, 18(7), 437–455. https://doi.org/10.3151/jact.18.437
- T. Maruya, H. Takeda, K. Horiguchi, S. Koyama, and K. L. Hsu.(2007), Simulation of steel corrosion in concrete based on the model of macro-cell corrosion circuit, Journal of Advanced Concrete Technology, 5(3), 343–362. https://doi.org/10.3151/jact.5.343
- Tsuru, T., Maeda, R. and Haruyama, S., (1979). Application of A-C corrosion monitor to localized corrosion. Corrosion Engineering, 28(12), 638–644. (in Japanese) https://doi.org/10.3323/jcorr1974.28.12_638
- Tuutti, K. (1982). Corrosion of steel in concrete. Cement-och betonginst, 468, 82–84.
- Verma, SK, Bhadauria, SS, & Akhtar, S. (2013). Evaluating effect of chloride attack and concrete cover on the probability of corrosion. Frontiers of Structural and Civil Engineering, 7, 379–390. https://doi.org/10.1007/s11709-013-0223-9
- Zhou, X., Shu, J., Zhang, J., Yan, Y., Gan, W., (2015). The impact of concrete cover thickness and chemical alkalinity on reinforcement corrosion. Advanced Materials Research, 1065–1069, 1957–1963. https://doi.org/10.4028/www.scientific.net/AMR.1065-1069.1957