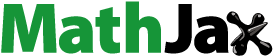
Abstract
Geopolymer cement (GPC) is a promising oil and gas well cementing alternative to Ordinary Portland Cement (OPC). OPC forms ettringite, causing water absorption and shrinkage. GPC, with lower calcium content, is expected to have lower shrinkage. This study assesses GPC's performance with elastomeric expandable additive (R-additive), up to 25 wt.%. The mixing, rheological test, free water, compressive strength, and linear expansion test were done in accordance with American Petroleum Institute Recommended Practice 10. Results demonstrated that increasing R-additive concentration reduced slurry density. Additionally, rheological properties improved, with plastic viscosity increasing from 48 to 104 cP and the yield point from 3.8 to 12.3 N/m2. The optimal R-additive concentration for ideal rheology was 20 wt.%. All formulations showed zero free water, while compressive strength and linear expansion (l/l0) varied with curing time. The study also confirmed self-healing in geopolymer cement, preventing flow through the cement sheath at varying temperatures. A 25 wt.% R-additive concentration allowed successful pumping at 4.8 barrels per minute with 100 psi pumping pressure. In a nutshell, the inclusion of R-additive between 10 to 25 wt% into geopolymer cement meets the standards of the oil and gas industry and becomes the potential replacement for OPC.
Reviewing editor:
1. Introduction
Current researchers look upon geopolymer cement (GPC) as an alternative to Ordinary Portland Cement (OPC) for oil and gas applications (Al Bakri Abdullah et al., Citation2012; Lemougna et al., Citation2016; Paiva et al., Citation2018; Pandey et al., Citation2012; Ridha et al., Citation2020; Ryu et al., Citation2013; Salehi et al., Citation2016; Wardhono, Citation2018). OPC is thought to be mechanically resistant when exposed to the high temperature and acidic environment of an oil well. On the other hand, studies by the number of researchers revealed that the OPC's structure had deteriorated when subjected to high temperatures, which is above 100 °C, and experienced strength reduction when exposed to an acidic and carbon dioxide (CO2) rich environment (Nasvi et al., Citation2012; Ridha et al., Citation2016; Ridha et al., Citation2020). Another issue with OPC as an oil well cement is the volumetric shrinkage of the OPC slurry during hydration and hardening (Reddy et al., Citation2009; Santra et al., Citation2009). The issue led to the formation of microannulus cracks that resulted from the contraction of cement's external dimensions (Nasvi et al., Citation2014; Rahman et al., Citation2020) as well as de-bonding between the cement, casing, and formation (Broni-Bediako et al., Citation2016; Nagral et al., Citation2014). As a result, the migration of gas and liquid from the formation to the surface may occur (Richhariya et al., Citation2020; Ridha et al., Citation2018) which may lead to the catastrophic event, such as blow out.
The ettringite (Al2Ca6H12O24S3) crystals that form in the hydrated OPC during the early phase of hydration as a result of the chemical reaction between calcium aluminate (CaAl2O4) and calcium sulfate (CaSO4), both of which are present in OPC, absorb water and cause volumetric shrinkage of the cement slurry (Chenevert & Shrestha, Citation1991; Merlini et al., Citation2008; Salehi et al., Citation2017). Expandable additives act as a shape-memory agent can expand before cement setting, can be mixed together with the cement slurry to prevent the shrinkage (Broni-Bediako et al., Citation2016; Nagral et al., Citation2014). At the same time, the occurrence of volumetric shrinkage can be avoided as the expandible additive possible to seal the microannulus cracks post-cement hardening (Kiran et al., Citation2017; Rahman et al., Citation2021).
The remarkable difference between geopolymer cement and OPC is that geopolymer is made up of geopolymerization process; the alkaline activators, such as sodium silicate, sodium hydroxide activate the alumino-silicate binder to form a 3D polymeric chain consisting of Si-O-Al bonds (Barlet-Gouédard et al., Citation2007; Liteanu et al., Citation2009; Salehi et al., Citation2017). The water requirement in geopolymerization is not significant as compared to the hydration of OPC, thus the volumetric shrinkage in GPC is projected to be less than that of OPC (Salehi et al., Citation2017). Due to the low calcium concentration of the aluminosilicate source, the calcium content of GPC is also significantly lower than that of OPC. Contrarily, the high calcium content in OPC causes, over time, increases in porosity and permeability over time and results in loss in the mechanical strength and integrity of the cement sheath. The high calcium content in OPC causes the calcium ions that are present in calcium-silicate-hydrate (C-S-H) to be used up in carbonation reactions to form calcium carbonate (CaCO3) (Zulkarnain et al., Citation2021). That is why, GPC will be more resistant to carbonation than OPC under conditions of high CO2 exposure in CO2-rich well settings due to its lower calcium concentration than OPC (Barlet-Gouédard et al., Citation2007; Nasvi et al., Citation2011; Uehara, Citation2010).
Other benefits of GPC's material properties over OPC, in addition to low shrinkage and resistance to carbonation, have been extensively studied in previous studies. These benefits include high mechanical strength, high pumpability, low permeability, low Young’s modulus, resistance to acid attacks, resistance to alkali-aggregate reaction, resistance to freeze-thaw cycles, stability at high temperatures, and tolerance to contamination with oil-based mud (Khalifeh et al., Citation2014; Rahman et al., Citation2021; Yang et al., Citation2009). Moreover, adopting GPC as an alternative to OPC offers a more affordable and environmentally friendly option because it requires less energy to manufacture, emits less carbon dioxide (0.184 tonnes per tonne of GPC generated vs. 1.0 tonnes per tonne of OPC produced), and costs less (He et al., Citation2019; Rahman et al., Citation2021; Yang et al., Citation2009).
In light of the limited research on the application of geopolymer cement for well cementing, as evidenced by a sparse body of studies (Barlet-Gouédard et al., Citation2007; Kiran et al., Citation2017; Liteanu et al., Citation2009; Rahman et al., Citation2021), this research seeks to contribute significantly to the field. The central focus of our study is to explore the impact of the elastomeric expandable additive (R-additive) on enhancing the performance of fly-ash-based strength-enhanced geopolymer cement (GPC) samples. By applying R-additive at various concentrations and subjecting the samples to curing at a temperature of 60 °C, we aim to investigate its influence comprehensively. Moreover, the introduction of slag cement into the GPC samples is intended to act as a strength enhancer in synergy with the R-additive. This study systematically examines key parameters including mixability, free water content, and slurry density. Additionally, we assess the nuanced effects of R-additive concentration on crucial properties, such as compressive strength, linear expansion, and fluid flow characteristics of the GPC samples. Through this research, we aim to not only fill a gap in existing literature but also provide valuable insights into optimizing the performance of geopolymer cement for well-cementing applications.
2. Materials and Methods
2.1. Materials
Geopolymer cement (GPC) was prepared using the fly ash Class F, following the ASTM C618-19 specifications (API, Citation2019c). Class F fly ash was obtained from the Tanjung Bin power plant in Johor, Malaysia. The reported total oxide content (SiO2 + Al2O3 + Fe2O3) of Class F fly ash is above 50%, and CaO content is below 18%. The composition of the Class F fly ash is summarized in .
Table 1. The chemical composition of the Tanjung Bin fly ash.
The alkaline activators used in the study were Sodium hydroxide (NaOH) and sodium silicate (Na2SiO3). The NaOH was purchased from Merck KGaA in liquid form, with a concentration of 8 M. The Na2SiO3 on the other hand, was purchased from R&M Chemical and had a solids content ranging from 51 to 54 wt.%. To enhance the strength of the cement to obtain the desired strength range for well cementing, slag cement was added to the sample as a strength enhancer, with a concentration of 10% of the weight of the fly ash. Furthermore, R-additive, which is an elastomeric expandable additive that consists of styrene-butadiene rubber with a specific gravity of 0.945. Its purpose was to induce expansion in the cement while preventing volumetric shrinkage of the cement sample.
2.2. Methods
2.2.1. Preparation of GPC samples
A total of four formulations, namely R10, R15, R20, and R25, were prepared. All the formulations consisted of a solid blend comprising 65% of the mixture, which includes fly ash, slag cement, and R-additive. Additionally, 35% of the formulation was an alkaline-activator solution composed of 8M NaOH and Na2SiO3 in a ratio of 0.25 Na2SiO3 to NaOH. The concentrations of the R-additive were varied across the formulations. R10 contained 10% by weight of the solid blend, R15 contained 15%, R20 contained 20%, and R25 contained 25%. To maintain a fixed percentage of the solid blend at 65% by weight of the mixture, the concentrations of fly ash and slag cement were adjusted accordingly. summarizes the specific adjustments made for each formulation.
Table 2. Concentrations of each constituent of the solid blend for each mix formulation.
Cement slurry with a 600 ml volume were prepared for each formulation. The slurry mixing process was carried out using the Constant Speed Mixer, Model 3260, developed by Ametek Chandler Engineering in Tulsa, OK, USA. Initially, the mixer was set to rotate at 4000 rpm for 15 s after pouring in all the solid constituents. Subsequently, the rotational speed was increased to a final value of 12,000 rpm for 35 s. The mixing procedure followed the guidelines outlined in API RP 10B-2 (API, Citation2019a), which also specified the procedures for conducting free water and rheology tests on the slurry.
The density of the slurry plays a crucial role in controlling the pumping of the slurry through the wellbore. During operations, the adopted density value is the equivalent circulating density (ECD), which combines the density of the slurry and the annular pressure loss. In this research, the density (ρ) of the slurry sample was measured in g/cm3 using a pressurized mud balance.
The free water test was conducted by pouring the slurry into a 250 ml measuring cylinder and then leaving the slurry undisturbed for two (2) hours. Subsequently, the water that was present on top of the cement was collected to determine the amount of free water.
The rheological properties of the slurry were measured using the Atmospheric Rheometer of Model 35 manufactured by Fann Instrument Company (Houston, TX, USA). Kinematic viscosity (v) was recorded at rotational speeds of 100 and 300 rpm and averages of five readings were taken for each rotational speed to calculate plastic viscosity (PV) and yield point (YP) as per EquationEquations (1)(1)
(1) and Equation(2)
(2)
(2) .
(1)
(1)
(2)
(2)
Where PV is the plastic viscosity measured in cP, YP is the yield point measured in N/m2, and v100 and v300 are the kinematic viscosities at 100 and 300 rpm measured in cP.
2.2.2. Measurement of compressive strength and linear expansion of GPC samples
Cube samples of GPC with dimensions of 2-inch × 2-inch × 2-inch for each formulation were casted and cured at the temperature of 60 °C in a water bath at atmospheric pressure. Measurements were taken for compressive strength and linear expansion on the samples that underwent various curing durations: 1, 14, 30, and 60 days. The compressive strength measurements followed the guidelines outlined in API SPEC 10A (API, Citation2019b), utilizing a Model 4207D Digital Compressive Strength Tester manufactured by Ametek Chandler Engineering (Tulsa, OK, USA). The compressive strength (F) of the samples was determined using EquationEquation (3)(3)
(3) .
(3)
(3)
Where P is the compressive strength measured in Pa, F is the compressive load at the point of failure measured in N, A is the cross-sectional surface area of the sample measured in m2.
The procedure outlined in API RP10B-5 (API, Citation2019c) was followed to measure linear expansion. An expansion cell, as defined by EquationEquation (4)(4)
(4) , was utilized for this purpose. The slurry was subjected to conditioning for 30 min and then poured into the expansion cell. The distance between the two steel balls of the cell before expansion was recorded as the initial length (Li). Subsequently, the cell was placed into the water bath that was pre-heated at 60 °C and cured for a pre-determined curing time. After curing, the cell was removed from the water bath, and the distance between the two steel balls after expansion was recorded as the final length (Lf) (Rahman et al., Citation2020).
(4)
(4)
Where Δl/l0 is the linear expansion measured by percentage; Lf is the final length measured in mm, and Li is the initial length measured in mm.
2.2.3. Fluid flow test and 3D images
A fluid flow test was conducted to preliminary provide the proof of concept for the self-healing technique. This test does not belong to API and was conducted as an additional test to the API testing through core flood equipment. In this test, brine water flowed through the cracked cement samples at the selected test temperature. The cracked cement plug was inserted inside the Hasler sleeve. The brine or hydrocarbon oil was injected from point A at a selected constant rate of 0.5 ml/min, and the effluent exit from point B was collected, as shown in . The test was conducted until no more effluent was collected at point B. The amount of effluent collected for every 5 min and the cumulated effluent weight (weight of water collected in grams) vs. time were recorded.
3. Results and discussion
3.1. Mixability, amount of free water, and density of GPC slurries
In , the correlation between density and concentration of R-additive is depicted. The results demonstrate that all formulations exhibited similar mixability and homogeneity, remaining stable during the mixing process. Additionally, no free water was observed in any of the formulations. Analysis of the density measurements indicated that as the concentration of R-additive increased from 10 to 15, 20, and 25%, the density of the slurry decreased from 1.76 to 1.74, 1.66, and 1.64 g/cm3. Notably, the most significant reduction in density, from 1.74 to 1.66 g/cm3, occurred when the concentration of R-additive was increased from 15 to 20%.
3.2. Rheological properties of GPC slurries
illustrates the Plastic Viscosity (PV) of GPC slurry at different concentrations of R-additive. The results demonstrate that higher concentrations of R-additive lead to increased rheological properties of the slurry, including PV and Yield Point (YP). Specifically, when the R-additive concentration was raised from 10 to 15, 20, and 25%, the PV values increased from 48 to 74, 83, and 104 cP, respectively. However, it is worth noting that maintaining PV below 100 cP is advisable due to pumpability challenges, as discussed by Igbani et al. (Citation2020) and Zahid et al. (Citation2018). Therefore, it is recommended to use R-additive concentrations below 20%.
The effect of increasing R-additive concentration on YP is depicted in . When the R-additive concentration was raised from 10 to 15, 20, and 25%, the YP values increased from 3.8 to 6.7, 10.1, and 12.3 N/m2, respectively. These results align with the findings of Richhariya et al. (Citation2020), who studied the impact of dual-coated polyacrylamide (DPAM) as an additive. They concluded that higher PV values were attributed to the gelation properties of the slurry and that an optimal concentration of 16% DPAM resulted in the desired rheological characteristics. Further additions of DPAM beyond 16% did not exhibit significant variations in PV.
3.3. Compressive strength of GPC samples
illustrates the relationship between curing time and the compressive strength (P) of GPC samples. The results indicate that increasing the duration of curing led to higher values of F and Δl/l0, with varying gradients observed over periods of 1, 14, 30, and 60 days. Notably, demonstrates that the R10 formulation attained the highest P value of 15 MPa (2177 psi). Conversely, highlights that R20 and R25 achieved the greatest Δl/l0 value of 0.7625%. Across all formulations, P and Δl/l0 consistently increased as the curing period extended from 1 to 60 days, as summarized below:
R10: P increased from 4.71 MPa (683 psi) to 2177 psi and Δl/l0 increased from 0.3508 to 0.5213% as per and , respectively.
R15: P increased from 6 MPa (871 psi) to 10.49 MPa (1522 psi) and Δl/l0 increased from 0.1432 to 0.3101% as per and , respectively.
R20: P increased from 5.14 MPa (745 psi) to 10.82 MPa (1569 psi) and Δl/l0 increased from 0.7625 to 0.9903%, as per and , respectively.
R25: P increased from 1.72 MPa (249 psi) to 10.33 MPa (1498 psi) and Δl/l0 increased from 0.7625 to 0.9985%, as per and , respectively.
and illustrate the impact of increasing the concentration of R-additive on P and Δl/l0 for samples cured over different time periods (1, 14, 30, and 60 days). With the exception of the 1-day cured samples, P decreased as the concentration of R-additive increased across all other samples. By referring to , it can be observed that when the concentration of R-additive was raised from 10 to 15%, P increased for the 1-day cured samples, remained constant for the 14-day cured samples, and exhibited a significant decline for the 30 and 60-day cured samples. Conversely, demonstrates that Δl/l0 decreased as the concentration of R-additive increased from 10 to 15%. Subsequently, as the concentration of R-additive was further increased from 15 to 20%, l/l0 showed a steeper decline compared to the previous decrease. However, the change in l/l0 resulting from an additional addition of R-additive from 20 to 25% was negligible.
The formulations employed in this study, namely R10, R15, R20, and R25, were specifically tailored to suit the requirements of the oil and gas industry and aligned with the recommendations put forth by Abbas et al. (Citation2013), Broni-Bediako et al. (Citation2016), Mao et al. (Citation2020), and Richhariya et al. (Citation2020). Before finalizing the formulations that fulfilled the API criteria, several trial mixes were formulated and evaluated. In , the failure modes of the samples during compressive strength testing are illustrated, revealing that the samples did not undergo complete crushing upon reaching the ultimate load. Consequently, the samples exhibited the ability to bear the load even after experiencing cracking.
3.4. Fluid flow test and 3D images
The cracked cement plug using elastomer at 25% (R25) was selected and used for this study and was inserted into the Hasler sleeve. The amount of effluent collected every 5 min and the cumulative weight of the collected water (measured in grams) over time were plotted in and , respectively. The injection fluid was maintained at a constant rate of 0.5 ml/min, and the maximum differential pressure was monitored to ensure it did not exceed 1500 psi, which is the compressive strength limit.
In the first 100 min of the test, the amount of effluent collected increased gradually, ranging from 0.5 to 3.5 g. It remained constant for the next 300 min before decreasing to zero after 450 min. The cumulative weight of water collected over time, as shown in , remained stable after the 450-min mark. This indicates that the self-healing process effectively prevented flow through the cement sheath.
Another test was conducted using preheated brine and a preheated cement sample, both maintained at a temperature of 60 °C before the test. The effluent collected and the cumulative effluent were plotted in and , respectively. The results demonstrated that temperature had a significant impact on the self-healing acceleration process in the cement sheath. The time required for self-healing decreased from 410 min at 27 °C () to 310 min at 60 °C (). This finding aligns with a study conducted by Reddy et al. (Citation2009), which highlighted the role of higher temperatures in accelerating the self-healing process.
and depict the cumulative effluent of hydrocarbon oil preheated to 60 °C. The effluent collected during the first 50 min of the test increased, followed by a period of constant effluent for the next 110 min. It then decreased to zero after 175 min, indicating a nearly 50% shorter time compared to the brine fluid test. The reaction of hydrocarbon oil in healing gaps or cracks in the cement sheath was observed to be much faster than that of the brine fluid, similar to the results observed in the expansion or swellable test.
Preliminary or proof of core flow concept testing demonstrates that the inclusion of self-healing additives effectively seals cracks. Using 3D imaging, we can observe the pore within the cement sheath before and after the core flood testing, as depicted in and . To obtain a detailed view of the cement plug, we utilized a high-resolution Micro CT Scanner, capturing 3D images before and after fluid flow testing. The porosity area and flow path across the cement sheath are indicated in blue. Notably, the porosity measurement, expressed as a percentage, decreased from 15 to 4.2%, while the permeability measurement in millidarcy (mD) decreased from 2.83 to 0.4 mD in the cement samples.
For comparative purposes, and show images of the original crack-free cement plug. In this scenario, the porosity and permeability measurements for design R25 are 3.16% and 0.28 mD, respectively. For design R20, the corresponding measurements are 4.78% and 0.2 mD.
3.5. Geopolymer cement field trial
The geopolymer cement slurry made up of fly ash, alkaline activator, and elastomer at 25% (R25) has been tested in the yard scale at the massive volume of 40 bbl. The rheology measurement has been taken at the surface and well condition and the results are presented in . It was observed that the 300 rpm reading for both measurements are below 275 cp, while the readings for 3 rpm are above 5 cp. The PV and YP measurements resulted in 21.84 cp and 3.42 lb/100ft, respectively, which in the acceptable range as per previous researchers (Igbani et al., Citation2020; Zahid et al., Citation2018). At the same time, shows the 10-min gel strength for both surface and well conditions are 10 and 13 lb/100ft, respectively. The rheology and gel strength measurements for the geopolymer cement slurry are within acceptable range of the operating company standard.
The geopolymer cement slurry was successfully pumped with the pumping rate of 4.8 barrel per minute as shown in with the pumping pressure of 0.69 MPa (100 psi). Meanwhile, the geopolymer cement slurry managed to achieve 7.76 MPa (1125 psi) in 24 h and 10.34 MPa (1500 psi) in 48 h for the destructive compressive strength test which passed the requirement for drilling the next hole section as required by the operating company (PETRONAS, Citation2016).
4. Conclusions
The research study involved the production of fly ash-based strength-enhanced GPC samples utilizing slag cement as the strength enhancer. These samples were created with varying concentrations of R-additive and subjected to curing at 60 °C. Several parameters were investigated, including mixability, amount of free water, density of slurries, compressive strength, and linear expansion. All formulations exhibited stability during mixing and demonstrated satisfactory mixability and homogeneity. The collected data indicated that the presence of R-additive did not result in any free water. The slurry density decreased from 1.76 to 1.64 g/cm3 and the rheological characteristics increased as the concentration of R-additive increased from 10 to 25%. The PV rose from 48 to 104 cP and the YP increased from 3.8 to 12.3 N/m2.
Based on the rheological properties, an optimal R-additive concentration of 20% was identified. The P and l/l0 exhibited varying slopes as the cure time extended. The maximum P of 15 MPa (2177 psi) was reached when the R-additive concentration was 10%, whereas the highest l/l0 of 0.7625% was obtained when the R-additive concentrations were between 20 and 25%. The flow test results demonstrated the efficacy of the self-healing process in preventing flow through the cement sheath, and the impact of temperature on the acceleration of this process was significant. The R25 formulation exhibited favorable properties for field conditions, as it was successfully pumped at a rate of 4.8 barrels per minute with a pumping pressure of 100 psi. Moreover, the formulations tested in this study, which included R-additive concentrations ranging from 10 to 25%, met the standards set by the oil and gas industry. Thus, this research significantly contributes to the practical advancement of geopolymer cement applications in the petroleum industry, particularly in well cementing.
The exploration of the elastomeric expandable additive (R-additive) and its influence on fly-ash-based strength-enhanced geopolymer cement (GPC) samples addresses a notable gap in current literature, offering valuable insights into optimizing cement formulations for enhanced performance in petroleum well environments. This research is poised to inform industry practices, offering a nuanced approach to designing geopolymer cement formulations tailored for the demanding conditions encountered in petroleum well operations. By establishing a foundation for improved cementing materials, this study paves the way for more resilient and efficient cementing practices, contributing to the overall integrity and longevity of petroleum well infrastructure. As the petroleum industry continues to evolve, the practical implications of this research are evident in its potential to enhance operational efficiency, reduce environmental impact, and ensure the long-term sustainability of well structures.
Acknowledgments
The authors are thankful to the technologists of Universiti Teknologi PETRONAS and PETRONAS Research Sdn. Bhd. for the commitment and support to carry out various activities related to experimental work in the laboratory.
Disclosure statement
No potential conflict of interest was reported by the author(s).
Data availability statement
All data used for this research is included in this manuscript.
Additional information
Funding
Notes on contributors
Siti Humairah A. Rahman
Dr. Siti Humairah Abd Rahman is currently working in management position at PETRONAS’ Research entity under Carbon Capture, Utilization, and Storage (CCUS) Department. The author has completed PhD from Universiti Teknologi PETRONAS (UTP) in Petroleum Engineering, majoring in Well Cementing. She did her first degree in Biochemical Engineering and obtained her Master’s degree in Chemical Engineering. Throughout her 20 years of experience in Oil and Gas industry, she cultivated a diverse range of experience from new technology development, scale up, consultation until commercialization of the technology, involve in forging mutually beneficial collaboration and strategic partnership alliance, negotiation and maximizing profitability from technology sales to the organization. Her area of interest includes but not limited to commercialization, CCUS and circular economy.
Afif Izwan Abd Hamid
Afif Izwan Abd Hamid is currently Research Scientist at Petroleum Engineering Department, Universiti Teknologi PETRONAS (UTP), Malaysia. He obtained his Bachelor’s degree in Petroleum Engineering, majoring in Drilling Engineering in 2015. He also earned his MSc in Petroleum Engineering at the same institution in 2018. His areas of interests are oilwell cementing, enhanced oil recovery, production enhancement and circular economy.
Nurul Nazmin Zulkarnain
Nurul Nazmin Zulkarnain works as a researcher at PETRONAS Research Sdn. Bhd. She earned her Bachelor's degree in Petroleum Engineering from Universiti Teknologi PETRONAS (UTP) in 2014, specializing in drilling engineering. Currently, she is pursuing her doctoral studies at the same university, concentrating on refining the calibration method for well cement measurement. Her interests encompass green technology, analytical chemistry, and well engineering.
Muhammad Aslam Md Yusof
Muhammad Aslam Md Yusof obtained a PhD in Petroleum Engineering from Universiti Teknologi PETRONAS (UTP), Perak, Malaysia and MEngSc in Petroleum Engineering from University of New South Wales, Australia. He is currently working as a lecturer/consultant in in Department of Petroleum Engineering at UTP. He has about than 12 years of experience in industry and academic. Currently, a member of the Centre of Research in Enhanced Oil recovery (COREOR), Institute of Hydrocarbon Recovery, UTP which effectively provide consultancy, professional training and focus research on formation damage, carbon sequestration and drilling fluid.
References
- Abbas, G., Irawan, S., Kumar, S., & Elrayah, A. A. I. (2013). Improving oil well cement slurry performance using hydroxypropyl methylcellulose polymer. Advanced Materials Research, 787, 222–227. https://doi.org/10.4028/www.scientific.net/AMR.787.222
- Abid, K., Gholami, R., Choate, P., & Nagaratnam, B. H. (2015). A review on cement degradation under CO2-rich environment of sequestration projects. Journal of Natural Gas Science and Engineering, 27, 1149–1157. https://doi.org/10.1016/j.jngse.2015.09.061
- Al Bakri Abdullah, M. M., Kamarudin, H., Abdulkareem, O. A. K. A., Ghazali, C. M. R., Rafiza, A. R., Norazian, M. N. (2012). Optimization of alkaline activator/fly ash ratio on the compressive strength of manufacturing fly ash-based geopolymer. Applied Mechanics and Materials, 110–116, 734–739. https://doi.org/10.4028/www.scientific.net/AMM.110-116.734
- API (2019a). API RP-10B2: Recommended practice for testing well cements (2nd ed.). American Petroleum Institute (API). 2019.
- API (2019b). API SPEC 10A: Cements and materials for well cementing. American Petroleum Institute (API).
- API (2019c). API RP-10B5: Recommended practice on determination of shrinkage and expansion of well cement formulations at atmospheric pressure. American Petroleum Institute (API).
- Barlet-Gouédard, V., Rimmelé, G., & Porcherie, O. (2007). Well technologies for CO2 geological storage: CO2-resistant cement. Oil & Gas Science and Technology, 62(3), 325–334. https://doi.org/10.2516/ogst:2007027
- Broni-Bediako, E., Joel, O. F., & Ofori-Sarpong, G. (2016). Oil well cement additives: A review of the common types. Oil & Gas Research, 112, 10000112. https://doi.org/10.4172/2472-0518.1000112
- Chenevert, M. E., & Shrestha, B. K. (1991). Chemical shrinkage properties of oilfield cements. SPE Drilling Engineering, 6(1), 37–231. https://doi.org/10.2118/16654-PA
- Diaz, E. I., & Allouche, E. N. (2010). Recycling of fly ash into geopolymer concrete: Creation of a database. 2010 IEEE Green Technologies Conference, Grapevine, TX, USA. https://doi.org/10.1109/GREEN.2010.5453790
- He, Z., Zhu, X., Wang, J., Mu, M., & Wang, Y. (2019). Comparison of CO2 emissions from OPC and recycled cement production. Construction and Building Materials, 211, 965–973. https://doi.org/10.1016/j.conbuildmat.2019.03.289
- Hewayde, E., Nehdi, M., Allouche, E., & Nakhla, G. (2006). Effect of geopolymer cement on microstructure, compressive strength and sulphuric acid resistance of concrete. Magazine of Concrete Research, 58(5), 321–331. https://doi.org/10.1680/macr.2006.58.5.321
- Igbani, S., Appah, D., & Ogoni, H. A. (2020). The application of response surface methodology in Minitab 16, to identify the optimal, comfort, and adverse zones of compressive strength responses in ferrous oilwell cement sheath systems. International Journal of Engineering and Modern Technology, 6(3), 20–39.
- Khalifeh, M., Hodne, H., Saasen, A., Integrity, O., & Eduok, E. I. (2016, October 25). Usability of geopolymers for oil well cementing applications: Reaction mechanisms, pumpability, and properties. SPE Asia Pacific Oil & Gas Conference and Exhibition, Perth, Australia. https://doi.org/10.2118/182354-MS
- Khalifeh, M., Saasen, A., & Vrålstad, T. (2014, April 2). Potential utilization of geopolymers in plug and abandonment operations. SPE Bergen One Day Seminar, Bergen, Norway. https://doi.org/10.2118/169231-MS
- Khalifeh, M., Todorovic, J., Vrålstad, T., Saasen, A., & Hodne, H. (2016). Long-term durability of rock-based geopolymers aged at downhole conditions for oil well cementing operations. Journal of Sustainable Cement-Based Materials, 6(4), 217–230. https://doi.org/10.1080/21650373.2016.1196466
- Kiran, R., Teodoriu, C., Dadmohammadi, Y., Nygaard, R., Wood, D., Mokhtari, M., & Salehi, S. (2017). Identification and evaluation of well integrity and causes of failure of well integrity barriers (A review). Journal of Natural Gas Science and Engineering, 45, 511–526. https://doi.org/10.1016/j.jngse.2017.05.009
- Lemougna, P. N., Wang, K., Tang, Q., Melo, U. C., & Cui, X. (2016). Recent developments on inorganic polymers synthesis and applications. Ceramics International, 42(14), 15142–15159. https://doi.org/10.1016/j.ceramint.2016.07.027
- Liteanu, E., Spiers, C. J., & Peach, C. J. (2009). Failure behaviour wellbore cement in the presence of water and supercritical CO2. Energy Procedia, 1(1), 3553–3560. https://doi.org/10.1016/j.egypro.2009.02.149
- Liu, X., Aughenbaugh, K., Nair, S., Shuck, M., & van Oort, E. (2016, September 14). Solidification of synthetic-based drilling mud using geopolymers. SPE Deepwater Drilling and Completion Conference, Galveston, TX, USA. https://doi.org/10.2118/180325-MS
- Majidi, B. (2013). Geopolymer technology, from fundamentals to advanced applications: A review. Materials Technology, 24, 79–87. https://doi.org/10.1179/175355509X449355
- Mao, W., Litina, C., & Al-Tabbaa, A. (2020). Development and application of novel sodium silicate microcapsule-based self-healing oil well cement. Materials, 13(2), 456. https://doi.org/10.3390/ma13020456
- Merlini, M., Artioli, G., Cerulli, T., Cella, F., & Bravo, A. (2008). Tricalcium aluminate hydration in additivated systems. A crystallographic study by SR-XRPD. Cement and Concrete Research, 38(4), 477–486. https://doi.org/10.1016/j.cemconres.2007.11.011
- Nagral, M. R., Ostwal, T., & Chitawadagi, M. (2014). Effect of curing temperature and curing hours on the properties of geopolymer concrete. International Journal of Computational Engineering Research, 4(9), 1–11.
- Nasvi, M. C. M., Ranjith, P. G., & Sanjayan, J. (2012, June). Comparison of mechanical behaviors of geopolymer and class G cement as well cement at different curing temperatures for geological sequestration of carbon dioxide. 46th U.S. Rock Mechanics/GeoMechanics Symposium, Chicago, IL, USA.
- Nasvi, M. C. M., Ranjith, P. G., Sanjayan, J., & Bui, H. (2014). Effect of temperature on permeability of geopolymer: A primary well sealant for carbon capture and storage wells. Fuel, 117, 354–363. https://doi.org/10.1016/j.fuel.2013.09.007
- Nasvi, M. C. M., Ranjith, P., & Sanjayan, J. (2011). Geopolymer as well cement and the variation of its mechanical behavior with curing temperature. Greenhouse Gases Science and Technology, 2(1), 46–58. https://doi.org/10.1002/ghg.39
- Paiva, M. D. M., Silva, E. C. C. M., Melo, D. M. A., Martinelli, A. E., & Schneider, J. F. (2018). A geopolymer cementing system for oil wells subject to steam injection. Journal of Petroleum Science and Engineering, 169, 748–759. https://doi.org/10.1016/j.petrol.2018.06.022
- Pandey, B., Kinrade, S. D., & Catalan, L. J. J. (2012). Effects of carbonation on the leachability and compressive strength of cement-solidified and geopolymer-solidified synthetic metal wastes. Journal of Environmental Management, 101, 59–67. https://doi.org/10.1016/j.jenvman.2012.01.029
- PETRONAS (2016). PETRONAS technical guidelines – Cementing. PETRONAS.
- Rahman, S. H. A., Irawan, S., Shafiq, N., & Rajeswary, R. (2020). Investigating the expansion characteristics of geopolymer cement samples in a water bath and compared with the expansion of ASTM Class-G cement. Heliyon, 6(2), e03478. https://doi.org/10.1016/j.heliyon.2020.e03478
- Rahman, S. H. A., Zulkarnain, N. N., & Shafiq, N. (2021). Experimental study and design of experiment using statistical analysis for the development of geopolymer matrix for oil-well cementing for enhancing the integrity. Crystals, 11(2), 139. https://doi.org/10.3390/cryst11020139
- Reddy, B. R., Liang, F., & Fitzgerald, R. (2009, April 20). Self-healing cements that heal without dependence on fluid contact. SPE International Symposium ion Oilfield Chemistry, The Woodlands, TX, USA. https://doi.org/10.2118/121555-MS
- Reddy, B. R., Xu, Y., Ravi, K., Gray, D., & Pattillo, P. D. (2009). Cement-shrinkage measurement in oilwell cementing—A comparative study of laboratory methods and procedures. SPE Drilling & Completion, 24(1), 104–114. https://doi.org/10.2118/103610-PA
- Richhariya, G., Dora, D. T. K., Parmar, K. R., Pant, K. K., Singhal, N., Lal, K., & Kundu, P. P. (2020). Development of self-healing cement slurry through the incorporation of dual-encapsulated polyacrylamide for the prevention of water ingress in oil well. Materials, 13(13), 2921. https://doi.org/10.3390/ma13132921
- Ridha, S., Abd Hamid, A. I., Abdul Halim, A. H., & Zamzuri, N. A. (2018). Elasticity and expansion test performance of geopolymer as oil well cement. IOP Conference Series: Earth and Environmental Science, 140, 012147. https://doi.org/10.1088/1755-1315/140/1/012147
- Ridha, S., Akmaluddin, M., & Salehudin, S. S. (2016). Microstructure investigation on nano-geopolymer cement cured under HPHT condition. ARPN Journal of Engineering and Applied Science, 11(1), 144–149.
- Ridha, S., Setiawan, R. A., Pramana, A. A., & Abdurrahman, M. (2020). Impact of wet supercritical CO2 injection on fly ash geopolymer cement under elevated temperatures for well cement applications. Journal of Petroleum Exploration and Production Technology, 10(2), 243–247. https://doi.org/10.1007/s13202-019-0693-y
- Ryu, G. S., Lee, Y. B., Koh, K. T., & Chung, Y. S. (2013). The mechanical properties of fly ash-based geopolymer concrete with alkaline activators. Construction and Building Materials, 47, 409–418. https://doi.org/10.1016/j.conbuildmat.2013.05.069
- Salehi, S., Khattak, M. J., Ali, N., & Rizvi, H. R. (2016, March). Development of geopolymer-based cement slurries with enhanced thickening time, compressive and shear bond strength and durability. IADC/SPE Drilling Conference and Exhibition, Fort Worth, TX, USA. https://doi.org/10.2118/178793-MS
- Salehi, S., Khattak, M. J., Ali, N., Ezeakacha, C., & Saleh, F. K. (2017). Study and use of geopolymer mixtures for oil and gas well cementing applications. Journal of Energy Resources Technology, 140(1), 012908. https://doi.org/10.1115/1.4037713
- Santra, A., Reddy, B. R., Liang, F., & Fitzgerald, R. (2009, April 20–22). Reaction of CO2 with Portland cement at downhole conditions and the role of pozzolanic supplements. SPE International Symposium on Oilfield Chemistry, The Woodlands, TX, USA. https://doi.org/10.2118/121103-MS
- Schütz, M. K., dos Santos, L. M., Coteskvisk, P. M., Menezes, S. C., Einloft, S., Dalla Vecchia, F. (2019). Evaluation of CO2 attack in wellbore class G cement: influence of epoxy resins, composites and minerals as additives. Greenhouse Gases Science and Technology, 9(6), 1276–1287. https://doi.org/10.1002/ghg.1928
- Sedić, K., Ukrainczyk, N., Mandić, V., Gaurina-Međimurec, N., & Šipušić, J. (2020). Carbonation of Portland-zeolite and geopolymer well-cement composites under geologic CO2 sequestration conditions. Cement and Concrete Composites, 111, 103615. https://doi.org/10.1016/j.cemconcomp.2020.103615
- Uehara, M. (2010). New concrete with low environmental load using the geopolymer method. Quarterly Report of RTRI, 51(1), 1–7. https://doi.org/10.2219/rtriqr.51.1
- Van Jaarsveld, J. G. S., Van Deventer, J. S. J., & Lorenzen, L. (1997). The potential use of geopolymeric materials to immobilise toxic metals: Part I. Theory and applications. Minerals Engineering, 10(7), 659–669. https://doi.org/10.1016/S0892-6875(97)00046-0
- Wardhono, A. (2018). The effect of sodium hydroxide molarity on strength development of non-cement class C fly ash geopolymer mortar. Journal of Physics: Conference Series, 947, 012001. https://doi.org/10.1088/1742-6596/947/1/012001
- Yang, Z. X., Ha, N. R., Jang, M. S., & Hwang, K. H. (2009). Geopolymer concrete fabricated by waste concrete sludge with silica fume. Materials Science Forum, 620–622, 791–794. https://doi.org/10.4028/www.scientific.net/MSF.620-622.791
- Zahid, M., Shafiq, N., Isa, M. H., & Gil, L. (2018). Statistical modeling and mix design optimization of fly ash based engineered geopolymer composite using response surface methodology. Journal of Cleaner Production, 194, 483–498. https://doi.org/10.1016/j.jclepro.2018.05.158
- Zulkarnain, N. N., Farhan, S. A., Sazali, Y. A., Shafiq, N., Rahman, S. H. A., Abd Hamid, A. I., & Habarudin, M. F. (2021). Reducing the waiting-on-cement time of geopolymer well cement using calcium chloride (CaCl2) as the accelerator: Analysis of the compressive strength and acoustic impedance for well logging. Sustainability, 13(11), 6128. https://doi.org/10.3390/su13116128