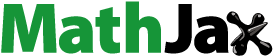
Abstract
Various amounts of chromium (Cr) were added to zinc oxide (ZnO) nanofibers (NFs) by electrospinning (ES), and pyrolysis was performed at 600 °C to form pure and Cr-doped ZnO NFs. The morphology, structure and optical properties of the NFs were characterized using scanning electron microscopy (SEM), X-ray diffraction (XRD) and ultraviolet-visible spectroscopy (UV-Vis). It was found that the structure of the NFs became rougher, and the diameter decreased with the increase of the Cr content. The maximum diameter of 150 nm was observed for 4 w% Cr-doped ZnO NFs. The bandgap energy decreased as the doping concentration increased. The gas-sensing properties of the Cr-doped ZnO NFs were investigated by measuring their response to acetone vapor. The results indicate that among all the samples, the 4 w% Cr-doped ZnO NFs exhibited the best sensing response to acetone vapor, with a response of 88.65–50 ppm acetone vapor. The response and recovery times were approximately 80 s and 55 s, respectively. Further, the Cr-doped electrospun NFs showed exceptional selectivity and stability, indicating their potential for high-performance gas sensor fabrication. This work reports an intriguing cost-effective lab designed gas sensor to investigate the sensing properties of pure and Cr-doped ZnO NFs.
1. Introduction
In the present world, one-dimensional-based metal oxide nanostructures have gained considerable attention owing to their potential applications (Chen et al., Citation2022). They have been used in many areas, such as wound dressing (Norouzi et al., Citation2021), drug delivery (Zhao et al., Citation2013), tissue engineering (Sridhar et al., Citation2015), gas sensors (Chow et al., Citation2013), perovskite solar cells (Mahmood et al., Citation2018) and supercapacitors (Pant et al., Citation2018). Among these, nanofibers (NFs) have become attractive materials owing to their properties such as large surface-to-volume ratio, high compact size, directional strength and good flexibility. Therefore, they provide functional and mechanical properties to the required material systems (Ghafari et al., Citation2017). Owing to their large surface-to-volume ratio, NFs have the potential to be used in a variety of applications where high porosity is desirable (Prabhu et al., Citation2022).
Zinc oxide (ZnO) is a chemically and thermally stable n-type semiconductor with a large bandgap (3.37 eV) and a high exciton binding energy (60 meV). Owing to its multifunctional properties, it has been extensively used in various applications (Jian et al., Citation2021; Liu et al., Citation2016). ZnO has received considerable interest for gas-sensing applications. There are various methods for synthesizing ZnO, that include precipitation (Ghorbani et al., Citation2015), hydrothermal (Agarwal et al., Citation2019), solvothermal (Y. Li et al., Citation2016) and electrospinning (ES) (Lee et al., Citation2019). Among these, ES is a useful technique for manufacturing 1D NFs because it is versatile, easy and inexpensive and allows continuous fibers to be obtained with the properties required for gas sensing.
One of the essential requirements of a gas sensor is to improve its sensitivity and operating temperature. Some of the ways that can be used to improve these properties are through doping, surface functionalization and tailoring the structure. Doping is an effective method for increasing the gas sensitivity of metal-oxide nanostructures. Doping is a process which is used to enhance the sensitivity of the sensing response. The most common type of dopant is chemical mixing, which is typically used in the fabrication of ZnO NFs. The addition of dopants to ZnO generates new active centers and plays a vital role in altering the structural properties. Transition metals such as nickel (Ni), chromium (Cr), cobalt (Co) and copper (Cu) are typically used as dopants in ZnO nanomaterials (N. H. Al-Hardan et al., Citation2013; Hsu et al., Citation2021; Maswanganye et al., Citation2017; L. Zhang, Dong, Xu, et al., Citation2017; X. Zhang, Dong, Liu, et al., Citation2017). These dopants help to improve the active sites, modify the ZnO resistance, lower the sensing temperature and enhance the selectivity and stability of the sensor (Bhati, Ranwa, Fanetti, et al., Citation2018; Bhati, Ranwa, Rajamani, et al., Citation2018). The addition of dopants changes the morphology of ZnO nanomaterials (Iqbal et al., Citation2013). The boundary interaction between the dopant and the host material frequently restricts crystallite formation, resulting in smaller grain sizes in doped ZnO NFs than in pure ZnO (Maciel et al., Citation2003).
The principal sources of indoor environmental contaminants are mainly caused due to the volatile organic compound (VOC) vapors, which are very toxic to the health (Francioso et al., Citation2008). Significant links between VOC and several types of cancer have been observed (Boeglin et al., Citation2006). More research into the detection of VOC vapors is necessary because of the severe challenges that impact health and the environment (N. H. Al-Hardan, Abdullah, Abdul Aziz, Ahmad, & Low, Citation2010; Boeglin et al., Citation2006; Rella et al., Citation2007). Gas sensors are generally used to monitor air quality in urban areas in detecting pollutants such as nitrogen dioxide (NO2), sulfur dioxide (SO2), carbon monoxide (CO) and ozone (O3) (Z. Li et al., Citation2019; Pavase et al., Citation2018; Yan et al., Citation2018). They are used to monitor harmful gases emitting from industrial facilities and hospitals (Halvorsen et al., Citation2018; Hussien et al., Citation2020; Prabhu et al., Citation2022, Citation2023). Further, gas sensors are implied in warning systems to detect gas leaks during natural calamities, such as volcanic eruptions or forest fires. Early detection helps to evacuate people and reduces the risks when exposed to hazardous gases (Bernard et al., Citation2020; Pan, Citation2020; Poland et al., Citation2020).
Qamar et al. (Citation2021) found that doping nickel (Ni) into ZnO enhances the response to visible light. They observed Nickel/ZnO/graphitic carbon nitride (NiZG)-based photocatalyst showed superior degradation and antimicrobial action compared to other photocatalysts, and it showed good stability in both dye degradation and bactericidal process. They concluded that the NiZG photocatalyst can be used as a practical solution in environmental remedies. Acetone is widely used as a solvent and an extraction reagent. Inhaling high concentrations (>10,000 ppm) can cause cephalalgia, nausea and cardiac arrest (Umeh et al., Citation2021). Furthermore, acetone is employed as a biomarker for diabetes because it can be detected in the exhaled breath of diabetic patients (Saasa et al., Citation2018). Therefore, we attempted to develop acetone sensors by spinning NFs using the ES method followed by pyrolysis. The morphology, structural properties and optical and gas-sensing properties of the Cr-doped ZnO NFs were investigated.
2. Methods and materials
2.1. Experimental details
Poly(vinylalcohol) (PVA), having a molecular weight of 115,000 g/mol, was procured from Loba chemicals Pvt. Ltd. Mumbai, India. Zinc acetate dihydrate was purchased from Sigma Aldrich. Acetone of laboratory grade and de-ionized water were used for solvent preparation. All the materials were used without further purification. A sol-gel process was employed to prepare the precursor PVA/ZnAc2 ceramic fibers. To prepare the solution, 7 g (14 w%) of PVA with a molecular weight of 115,000 was stirred for 2.5 h at 90 °C. Following that 7.5 w% of zinc acetate (ZnAc2) was then added to the stirred PVA solution, and the resulting solution was magnetically stirred overnight at 50 °C. Similarly, various amounts of Cr (1, 2, 3 and 4 w%) were added to the precursor solution and stirred for 6 h at 55 °C. The obtained solution was loaded onto a 5 ml syringe using an electrospinning setup as depicted in . The needle was connected to a voltage maintained at 18 kV, at a distance of 20 cm from the collector. The obtained fibers were dried at 50 °C for 2 h and then pyrolyzed for 2 h at 550 °C.
2.2. Characterization
The crystalline structure of the ZnO/Cr NF was investigated using powder X-ray diffraction (XRD, Rigaku MiniFlex 600, Japan) from 20° to 80° at a speed of 0.5° m−1. The morphology of the NF was studied using scanning electron microscopy (SEM; CARL, ZEISS, Germany). The optical spectra of the NF were analyzed using an ultraviolet-visible (UV-VIS) spectrometer (Shimadzu-1800, Japan) in the range of 100–900 nm. The gas sensing characteristics were tested in a chamber developed in our laboratory.
2.3. Measurement of gas sensor
The sensing characteristics of the pure and Cr-doped ZnO NFs were studied using a setup developed in our laboratory. The setup consisted of a closed stainless-steel test chamber with a volume of 20 L, which had an inlet and outlet for acetone vapor. To fabricate the gas sensors, the precursor composite fibers were spun on the quartz substrates and pyrolyzed for 2 h at 550 °C. Following pyrolysis, silver paste was coated on the edges of the sample and attached to a silver electrode to measure resistance. All the measurements were conducted within a temperature range of 25–350 °C with a controllable heater unit. The resistance values were measured using a digital multimeter.
3. Results and discussion
3.1. Morphological study
(a) depicts the SEM images of the pure and Cr-doped ZnO NFs, as well as their size distribution pyrolyzed at 600 °C. The ZnO NFs were smooth and continuous with an average fiber diameter (AFD) of 256 nm. shows the Cr-doped ZnO NFs with a rough surface and an AFD in the range of 150–1970 nm. PVA completely evaporated during pyrolysis, and the addition of Cr caused the NFs to shrink. Wang et al. (Citation2010) observed a broader distribution of NFs with an AFD of 130 nm for as-spun fibers. Hilal Elhousseini et al. (Citation2020) observed smooth and continuous as-spun NFs with an AFD of 400 nm. After pyrolysis at 500 °C, the AFD reduced to 177 nm, with a rough surface.
Figure 2. AFD of the NFs of (a) pure ZnO, (b) 1 w%, (c) 2 w%, (d) 3 w% and (e) 4 w% Cr-doped ZnO NFs.


To confirm the presence and spatial distribution of elements such as zinc (Zn), chromium (Cr) and oxygen (O), EDX analysis has been carried out, and it is depicted in . The EDX analysis confirms the presence of Cr in the fibers pyrolyzed at 550 °C with uniform distribution.
3.2. Structural
shows the XRD patterns of ZnO and Cr-doped NFs pyrolyzed at 550 °C. The NFs showed a polycrystalline nature, with peaks corresponding to the hexagonal wurtzite structure of ZnO. The observed peaks are in line with the standard ICDD data (01-074-9943) for ZnO. The fibers showed (100), (002), (101), (110), (103) and (112) peaks with maximum intensities along the (101) plane corresponding to the c-axis orientation. No additional peaks attributed to the metallic phases of Cr are observed. From , it can be observed that the XRD peaks slightly shift toward higher angles as the doping concentration increases. This is attributed to the lattice expansion when the Cr atom gets substituted in the ZnO crystal lattice. Further due to the higher ionic radius of Zn2+(0.74 Å) than that of Cr3+ (0.63 Å), a slight decrease in crystal lattice constant is observed as the Cr concentration increases. With the increase in Cr concentration in ZnO lattice, the volume of the unit cell was also decreased suggesting that the Cr ion fits into the Zn site in ZnO structure (Habib et al., Citation2019; Kaur et al., Citation2015; L. Li et al., Citation2009). The crystallinity of the fibers was determined using EquationEquation (1)(1)
(1) .
(1)
(1)
Pure ZnO had a crystallite size of 23 nm, and it decreased with the increase in Cr concentration. The 4 w% Cr-doped NFs had a size of 20 nm. This is due to the distortion of Cr3+ ions in the host ZnO lattice. The lattice parameters a and c were found to be 3.2 Å and 5.21 Å, matching the standard ZnO values. According to G. H. Zhang et al. (Citation2016), the highest peak of the ZnO crystalline phase was oriented along the (101) plane. Furthermore, Hilal Elhousseini et al. (Citation2020) reported that the ZnO fibers pyrolyzed at 600 °C exhibited sharp peaks with preferred orientation along (101) plane.
3.3. Optical properties
The optical properties of the pure and Cr-doped NFs were determined using a UV-Vis spectrometer. shows the transmittance spectra and bandgap of undoped and Cr-doped ZnO NFs pyrolyzed at 550 °C. The transmittance of the NFs was observed to be 80% in the visible region for pure ZnO. Similarly, for doped NFs, it was found to be 90% for 1 w%, 65% for 2 w%, 85% for 3 w% and 85% for 4 w% Cr concentration. The absorption edge of the NFs was 385 nm. The sharp absorption edge corresponds to better optical and structural properties of the fibers. The better transparency of the NFs was due to the increased crystallinity and fewer defects. The lattice orientation of the NFs increases with increasing crystallinity and decreasing light scattering. The bandgaps of the NFs were calculated using EquationEquation (2)(2)
(2) .
(2)
(2)
where α is the adsorption coefficient, hν is the incident photon energy, Eg is the optical bandgap and n is the index. The energy bandgap of the NFs was measured to be 3.32 eV, which is in line with the theoretical value for ZnO. It was observed that the optical band gap value for Cr-doped NFs decreases from 3.2 to 2.65 eV with an increase in doping concentration. Rajeswari Yogamalar and Chandra Bose (Citation2011) reported a decrease in the optical band gap values from 2.99 to 2.85 eV for ZnO nanorods. Similarly, Andhare et al. (Citation2020) observed that doping with Zn caused a decrease in the bandgap upon doping, and the doping of Zn led to a decrease in the optical bandgap of the cobalt nanostructure from 2.80 to 2.25 eV. Further, Hosseini et al. (Citation2015) observed a decrease in the bandgap from 3.25 to 3.18 eV upon silver doping. A decrease in the optical bandgap energy is observed in Cr-doped ZnO NFs, which may be attributed to the formation of defects and an increase in the flow of electrons from the valence band (VB) to the conduction band (CB) of the ZnO material. This leads to an increase in the electronic conductivity of the sensing material (Chang, Yang, et al., Citation2014).
3.4. Gas sensing properties
The general sensing mechanism of an n-type semiconductor material can be understood using the space-charger layer method (An et al., Citation2014; Gao & Wang, Citation2005; Sonker et al., Citation2015), which correlates the change in sensor resistance in air and gas atmospheric media. When the NFs surface is exposed to air, some of the oxygen molecules are chemisorbed, forming chemisorbed oxygen species (
and
). This results in the extraction of electrons from the conduction band (CB) of the material under various operating temperatures, and the following reactions occur (Barsan & Weimar, Citation2001). depicts the gas sensing mechanism for acetone vapor.
Likewise, when the NFs are exposed to reducing gases such as hydrogen, carbon monoxide and methane, the surface reacts with chemisorbed oxygen molecules, decreasing the number of oxygen molecules. This decreases electron extraction from the CB, thereby reducing the sensor resistance. Similarly, when the NFs are exposed to oxidizing gases, such as oxygen, nitrogen dioxide and sulfuric acid, the chemisorbed oxygen molecules are oxidized, increasing the number of oxygen molecules. This increases the number of electrons extracted from the conduction band, thereby increasing sensor resistance.
The gas sensing response of pure and Cr-doped ZnO NFs was investigated by measuring the resistance at operating temperatures ranging from 25 °C to 350 °C. The concentration of acetone vapor was maintained constant at 50 ppm, while the temperature was varied at intervals of 50 °C. The sensitivity (S) can be defined as:
(7)
(7)
where Ra and Rg are the resistances of the sensor in the air gaseous atmosphere.
shows the gas response with respect to the working temperature. For the pure ZnO, the sensing response increased with the operating temperature up to 260 °C and decreased as the optimum temperature increased. The maximum response of 10.05 was observed for pure ZnO. summarizes the sensing response carried out by various researchers for acetone vapor.
Table 1. Comparison of sensing parameters for acetone vapor.
Similarly, the sensing responses for Cr-doped NFs increased with the temperature until 260 °C and then decreased with further increase in temperature. The 4 w% Cr doping showed the highest response of 88.65. The addition of Cr enhanced the sensing response at lower temperatures by increasing the surface activity because of its lower ionization energy than that of Zn. This leads to a reduction in the activation energy of the chemisorbed surface, resulting in an increase in gas adsorption (N. Al-Hardan, Abdullah, Abdul Aziz, & Ahmad, Citation2010). At lower temperatures, the reaction rate between the acetone vapor and the absorbed oxygen species was low because of the high activation energy. As the operating temperature increased, the response improved owing to the availability of sufficient thermal energy to overcome the barrier height. However, at higher temperatures, the adsorbed oxygen may be desorbed from the surface, leading to a decrease in the response because it is not sufficient to react with the vapors. This might be the probable cause of the decrease in response at higher temperatures (Barreca et al., Citation2010; Gong et al., Citation2006). The response and recovery times of the 4 w% Cr-doped ZnO sample were approximately 80 and 55 s for 50-ppm acetone. shows the transient responses of the 4 w% Cr-doped ZnO NFs in acetone vapor at various concentrations. It can be observed that the response increased with increasing gas concentration with respect to time.
Figure 8. Transient responses of the 4 w% Cr-doped NFs for various concentrations of acetone ethanol vapor.

Cross-sensitivity is another important characteristic of a gas sensor. The 4 w% Cr-doped ZnO NF sensor was exposed to 50 ppm of different gases (CH3COCH3, C2H5OH, NH3, C6H6 and CH3OH). depicts the selectivity of the sensors for several gases at 260 °C with a vapor concentration of 50 ppm. All the ZnO sensors showed a higher response to acetone vapor than to the other vapors. The responses to C2H5OH, CH3COOH, C3H8, H2S and NH3 are 24, 13, 5, 27 and 8.3, respectively.
4. Conclusions
The undoped and Cr-doped ZnO NFs were prepared using the ES technique for gas sensing applications. The morphology of 4 w% Cr-doped NFs was found having lower AFD of 150 nm pyrolyzed at 550 °C The XRD results revealed the high crystallinity of the ZnO NFs with preferred orientation along (101) plane. The bandgap energy decreases with increasing dopant concentration. The maximum response increased with operating temperature and a maximum response of 88.65 was found for 4 w% Cr-doped NFs at an operating temperature of 260 °C. Furthermore, no change in the response was observed when compared with other vapors.
CRediT author statement
Vishwas Rajesh: Literature survey and original draft preparation. Niranjan N. Prabhu: Literature survey, editing and reviewing. Basavanna Shivamurthy: Conceptualization, supervision and administration.
Acknowledgment
The authors gratefully acknowledge support provided by the Manipal Academy of Higher Education.
Disclosure statement
No potential conflict of interest was reported by the authors.
Data availability statement
The authors confirm that the data supporting the findings of this study are available within the article.
Additional information
Funding
Notes on contributors
Vishwas Rajesh
Mr. Vishwas Rajesh is a student of Manipal Institute of Technology, Manipal perusing his B.Tech degree in the field of Mechanical & Industrial Engineering. He has done a project based on electrospinning and his main interest is focused on Automotive Engineering field.
Niranjan N. Prabhu
Mr. Niranjan N Prabhu is a research scholar in the department of Mechanical & Industrial Engineering at Manipal Institute of Technology, Manipal Academy of Higher Education, Manipal. He received his B.E degree in Mechanical Engineering in the year 2013, and MTech in Design Engineering in the year 2016. He has worked as an Automotive Design Engineer from 2017 to 2020 in Pune. His research interests include electrospinning, and gas sensors.
Basavanna Shivamurthy
Dr. B Shivamurthy, received the B.E. degree in mechanical engineering from Mysore University, India, in 1991, M. Sc. Engineering by research degree in mechanical engineering from Visvesvaraya Technological University, Belagavi, India in 2008. He obtained Ph. D in Metallurgical & Materials Engineering from National Institute of Technology Karnataka in 2014. He is currently working as Professor at Manipal Institute of Technology, Manipal Academy of Higher Education, Manipal, India. From 1991 to 2001, he was worked with the polymer engineering Industries in production, R&D in various sectors such as polyester films, thermoset & thermoplastic composites for various applications. He also executed selection and installation of plant and machinery for the same. Further 2001 till date he is working as an Engineering faculty and researcher in India. He also worked as program coordinator at Manipal School of Engineering and IT at Dubai. His research interest includes polymer composites, Nano hybrid composites for electronics, biomedical, and engineering sectors. Dr. B. Shivamurthy published research articles in the field of electronic materials, wear-resistant polymers, structure properties of various Nano hybrid polymer composites, fire retardant materials, EMI Shielding, and impact-resistant materials.
References
- Agarwal, S., Rai, P., Gatell, E. N., Llobet, E., Güell, F., Kumar, M., & Awasthi, K. (2019). Gas sensing properties of ZnO nanostructures (flowers/rods) synthesized by hydrothermal method. Sensors and Actuators B: Chemical, 292, 24–31. https://doi.org/10.1016/j.snb.2019.04.083
- Al-Hardan, N., Abdullah, M. J., Abdul Aziz, A., & Ahmad, H. (2010). Low operating temperature of oxygen gas sensor based on undoped and Cr-doped ZnO films. Applied Surface Science, 256(11), 3468–3471. https://doi.org/10.1016/j.apsusc.2009.12.055
- Al-Hardan, N. H., Abdullah, M. J., Abdul Aziz, A., Ahmad, H., & Low, L. Y. (2010). ZnO thin films for VOC sensing applications. Vacuum, 85(1), 101–106. https://doi.org/10.1016/j.vacuum.2010.04.009
- Al-Hardan, N. H., Abdullah, M. J., & Aziz, A. A. (2013). Performance of Cr-doped ZnO for acetone sensing. Applied Surface Science, 270, 480–485. https://doi.org/10.1016/j.apsusc.2013.01.064
- An, D., Li, Y., Lian, X., Zou, Y., & Deng, G. (2014). Synthesis of porous ZnO structure for gas sensor and photocatalytic applications. Colloids and Surfaces A: Physicochemical and Engineering Aspects, 447, 81–87. https://doi.org/10.1016/j.colsurfa.2014.01.060
- Andhare, D. D., Patade, S. R., Kounsalye, J. S., & Jadhav, K. M. (2020). Effect of Zn doping on structural, magnetic and optical properties of cobalt ferrite nanoparticles synthesized via. Co-precipitation method. Physica B: Condensed Matter, 583, 412051. https://doi.org/10.1016/j.physb.2020.412051
- Barreca, D., Bekermann, D., Comini, E., Devi, A., Fischer, R. A., Gasparotto, A., MacCato, C., Sberveglieri, G., & Tondello, E. (2010). 1D ZnO nano-assemblies by plasma-CVD as chemical sensors for flammable and toxic gases. Sensors and Actuators B: Chemical, 149(1), 1–7. https://doi.org/10.1016/j.snb.2010.06.048
- Barsan, N., & Weimar, U. (2001). Conduction model of metal oxide gas sensors. Journal of Electroceramics, 7(3), 143–167. https://doi.org/10.1023/A:1014405811371
- Bernard, A., Villacorte, E., Maussen, K., Caudron, C., Robic, J., Maximo, R., Rebadulla, R., Bornas, M. A. A. V., & Solidum, R. U. (2020). Carbon dioxide in Taal Volcanic Lake: A simple gasometer for volcano monitoring. Geophysical Research Letters, 47(24), e2020GL090884. https://doi.org/10.1029/2020GL090884
- Bhati, V. S., Ranwa, S., Fanetti, M., Valant, M., & Kumar, M. (2018). Efficient hydrogen sensor based on Ni-doped ZnO nanostructures by RF sputtering. Sensors and Actuators B: Chemical, 255, 588–597. https://doi.org/10.1016/j.snb.2017.08.106
- Bhati, V. S., Ranwa, S., Rajamani, S., Kumari, K., Raliya, R., Biswas, P., & Kumar, M. (2018). Improved sensitivity with low limit of detection of a hydrogen gas sensor based on rGO-loaded Ni-doped ZnO nanostructures. ACS Applied Materials & Interfaces, 10(13), 11116–11124. https://doi.org/10.1021/acsami.7b17877
- Boeglin, M. L., Wessels, D., & Henshel, D. (2006). An investigation of the relationship between air emissions of volatile organic compounds and the incidence of cancer in Indiana counties. Environmental Research, 100(2), 242–254. https://doi.org/10.1016/j.envres.2005.04.004
- Chang, C. J., Chen, J. K., & Yang, T. L. (2014). Cr-doped ZnO based NO2 sensors with high sensitivity at low operating temperature. Journal of the Taiwan Institute of Chemical Engineers, 45(4), 1876–1882. https://doi.org/10.1016/j.jtice.2013.10.010
- Chang, C. J., Yang, T. L., & Weng, Y. C. (2014). Synthesis and characterization of Cr-doped ZnO nanorod-array photocatalysts with improved activity. Journal of Solid State Chemistry, 214, 101–107. https://doi.org/10.1016/j.jssc.2013.09.039
- Chen, L., Yu, Q., Pan, C., Song, Y., Dong, H., Xie, X., Li, Y., Liu, J., Wang, D., & Chen, X. (2022). Chemiresistive gas sensors based on electrospun semiconductor metal oxides: A review. Talanta, 246, 123527. https://doi.org/10.1016/j.talanta.2022.123527
- Chow, L., Lupan, O., Chai, G., Khallaf, H., Ono, L. K., Roldan Cuenya, B., Tiginyanu, I. M., Ursaki, V. V., Sontea, V., & Schulte, A. (2013). Synthesis and characterization of Cu-doped ZnO one-dimensional structures for miniaturized sensor applications with faster response. Sensors and Actuators A: Physical, 189, 399–408. https://doi.org/10.1016/j.sna.2012.09.006
- Francioso, L., Forleo, A., Taurino, A. M., Siciliano, P., Lorenzelli, L., Guarnieri, V., Adami, A., & Agnusdei, G. (2008). Linear temperature microhotplate gas sensor array for automotive cabin air quality monitoring. Sensors and Actuators B: Chemical, 134(2), 660–665. https://doi.org/10.1016/j.snb.2008.06.008
- Gao, T., & Wang, T. H. (2005). Synthesis and properties of multipod-shaped ZnO nanorods for gas-sensor applications. Applied Physics A, 80(7), 1451–1454. https://doi.org/10.1007/s00339-004-3075-2
- Ghafari, E., Feng, Y., Liu, Y., Ferguson, I., & Lu, N. (2017). Investigating process-structure relations of ZnO nanofiber via electrospinning method. Composites Part B: Engineering, 116, 40–45. https://doi.org/10.1016/j.compositesb.2017.02.026
- Ghorbani, H. R., Mehr, F. P., Pazoki, H., & Rahmani, B. M. (2015). Synthesis of ZnO nanoparticles by precipitation method. Oriental Journal of Chemistry, 31(2), 1219–1221. https://doi.org/10.13005/ojc/310281
- Gong, H., Hu, J. Q., Wang, J. H., Ong, C. H., & Zhu, F. R. (2006). Nano-crystalline Cu-doped ZnO thin film gas sensor for CO. Sensors and Actuators B: Chemical, 115(1), 247–251. https://doi.org/10.1016/j.snb.2005.09.008
- Habib, I. Y., Tajuddin, A. A., Noor, H. A., Lim, C. M., Mahadi, A. H., & Kumara, N. T. R. N. (2019). Enhanced carbon monoxide-sensing properties of chromium-doped ZnO nanostructures. Scientific Reports, 9(1), 9207. https://doi.org/10.1038/s41598-019-45313-w
- Halvorsen, H. P., Grytten, O. A., Svendsen, M. V., & Mylvaganam, S. (2018). Environmental monitoring with focus on emissions using IoT platform for mobile alert [Paper presentation]. 2018 28th EAEEIE Annual Conference, EAEEIE 2018, 1–7. https://doi.org/10.1109/EAEEIE.2018.8534197
- Hilal Elhousseini, M., Isık, T., Kap, Ö., Verpoort, F., & Horzum, N. (2020). Dual remediation of waste waters from methylene blue and chromium (VI) using thermally induced ZnO nanofibers. Applied Surface Science, 514, 145939. https://doi.org/10.1016/j.apsusc.2020.145939
- Hosseini, S. M., Sarsari, I. A., Kameli, P., & Salamati, H. (2015). Effect of Ag doping on structural, optical, and photocatalytic properties of ZnO nanoparticles. Journal of Alloys and Compounds, 640, 408–415. https://doi.org/10.1016/j.jallcom.2015.03.136
- Hsu, K. C., Fang, T. H., Hsiao, Y. J., & Li, Z. J. (2021). Rapid detection of low concentrations of H2S using CuO-doped ZnO nanofibers. Journal of Alloys and Compounds, 852, 157014. https://doi.org/10.1016/j.jallcom.2020.157014
- Hussien, N. M., Mohialden, Y. M., Ahmed, N. T., Mohammed, M. A., & Sutikno, T. (2020). A smart gas leakage monitoring system for use in hospitals. Indonesian Journal of Electrical Engineering and Computer Science, 19(2), 1048. https://doi.org/10.11591/ijeecs.v19.i2.pp1048-1054
- Iqbal, J., Jan, T., & Ronghai, Y. (2013). Effect of Co doping on morphology, optical and magnetic properties of ZnO 1-D nanostructures. Journal of Materials Science: Materials in Electronics, 24(11), 4393–4398. https://doi.org/10.1007/s10854-013-1415-8
- Jian, S., Tian, Z., Hu, J., Zhang, K., Zhang, L., Duan, G., Yang, W., & Jiang, S. (2021). Enhanced visible light photocatalytic efficiency of La-doped ZnO nanofibers via electrospinning-calcination technology. Advanced Powder Materials, 1(2), 100004. https://doi.org/10.1016/j.apmate.2021.09.004
- Kaur, P., Kumar, S., Negi, N. S., & Rao, S. M. (2015). Enhanced magnetism in Cr-doped ZnO nanoparticles with nitrogen co-doping synthesized using sol–gel technique. Applied Nanoscience, 5(3), 367–372. https://doi.org/10.1007/s13204-014-0326-1
- Lee, J. H., Kim, J. Y., Kim, J. H., & Kim, S. S. (2019). Enhanced hydrogen detection in ppb-level by electrospun SnO2-loaded ZnO nanofibers. Sensors (Switzerland), 19(3), 726. https://doi.org/10.3390/s19030726
- Li, L., Wang, W., Liu, H., Liu, X., Song, Q., & Ren, S. (2009). First principles calculations of electronic band structure and optical properties of Cr-doped ZnO. The Journal of Physical Chemistry C, 113(19), 8460–8464. https://doi.org/10.1021/jp811507r
- Li, Y., Liu, M., Lv, T., & Lian, X. X. (2016). Gas sensing and photoluminescence of flower-like ZnO hollow microspheres synthesised using facile solvothermal method. Materials Technology, 31(1), 1–6. https://doi.org/10.1179/1753555715Y.0000000014
- Li, Z., Askim, J. R., & Suslick, K. S. (2019). The optoelectronic nose: Colorimetric and fluorometric sensor arrays. Chemical Reviews, 119(1), 231–292. https://doi.org/10.1021/acs.chemrev.8b00226
- Liu, Y. J., Zhang, H. D., Yan, X., Zhao, A. J., Zhang, Z. G., Si, W. Y., Gong, M. G., Zhang, J. C., & Long, Y. Z. (2016). Effect of Ce doping on the optoelectronic and sensing properties of electrospun ZnO nanofibers. RSC Advances, 6(89), 85727–85734. https://doi.org/10.1039/C6RA16491A
- Maciel, A. P., Lisboa-Filho, P. N., Leite, E. R., Paiva-Santos, C. O., Schreiner, W. H., Maniette, Y., & Longo, E. (2003). Microstructural and morphological analysis of pure and Ce-doped tin dioxide nanoparticles. Journal of the European Ceramic Society, 23(5), 707–713. https://doi.org/10.1016/S0955-2219(02)00190-5
- Mahmood, K., Khalid, A., Ahmad, S. W., & Mehran, M. T. (2018). Indium-doped ZnO mesoporous nanofibers as efficient electron transporting materials for perovskite solar cells. Surface and Coatings Technology, 352, 231–237. https://doi.org/10.1016/j.surfcoat.2018.08.039
- Maswanganye, M. W., Rammutla, K. E., Mosuang, T. E., & Mwakikunga, B. W. (2017). The effect of Co and In combinational or individual doping on the structural, optical and selective sensing properties of ZnO nanoparticles. Sensors and Actuators B: Chemical, 247, 228–237. https://doi.org/10.1016/j.snb.2017.02.039
- Nakarungsee, P., Srirattanapibul, S., Issro, C., Tang, I. M., & Thongmee, S. (2020). High performance Cr doped ZnO by UV for NH3 gas sensor. Sensors and Actuators A: Physical, 314, 112230. https://doi.org/10.1016/j.sna.2020.112230
- Norouzi, M. A., Montazer, M., Harifi, T., & Karimi, P. (2021). Flower buds like PVA/ZnO composite nanofibers assembly: Antibacterial, in vivo wound healing, cytotoxicity and histological studies. Polymer Testing, 93, 106914. https://doi.org/10.1016/j.polymertesting.2020.106914
- Pan, L. (2020). Preventing forest fires using a wireless sensor network. Journal of Forest Science, 66(3), 97–104. https://doi.org/10.17221/151/2019-JFS
- Pant, B., Park, M., Ojha, G. P., Park, J., Kuk, Y. S., Lee, E. J., Kim, H. Y., & Park, S. J. (2018). Carbon nanofibers wrapped with zinc oxide nano-flakes as promising electrode material for supercapacitors. Journal of Colloid and Interface Science, 522, 40–47. https://doi.org/10.1016/j.jcis.2018.03.055
- Pavase, T. R., Lin, H., Shaikh, Q. u. a., Hussain, S., Li, Z., Ahmed, I., Lv, L., Sun, L., Shah, S. B. H., & Kalhoro, M. T. (2018). Recent advances of conjugated polymer (CP) nanocomposite-based chemical sensors and their applications in food spoilage detection: A comprehensive review. Sensors and Actuators, B: Chemical, 273, 1113–1138. https://doi.org/10.1016/j.snb.2018.06.118
- Poland, M. P., Lopez, T., Wright, R., & Pavolonis, M. J. (2020). Forecasting, detecting, and tracking volcanic eruptions from space. Remote Sensing in Earth Systems Sciences, 3(1–2), 55–94. https://doi.org/10.1007/s41976-020-00034-x
- Prabhu, N. N., Jagadeesh Chandra, R. B., Rajendra, B. V., George, G., Mourad, A. H. I., & Shivamurthy, B. (2022). Electrospun zinc oxide nanofiber based resistive gas/vapor sensors - A review. Engineered Science, 19, 59–82. https://doi.org/10.30919/es8d612
- Prabhu, N. N., Shivamurthy, B., Anandhan, S., Rajendra, B. V., Basanna, J. C. R., & Srivathsa, M. (2023). An investigation on the acetone and ethanol vapor-sensing behavior of sol–gel electrospun ZnO nanofibers using an indigenous setup. ACS Omega, 8(51), 49057–49066. https://doi.org/10.1021/acsomega.3c06744
- Qamar, M. A., Shahid, S., Javed, M., Iqbal, S., Sher, M., Bahadur, A., Al-Anazy, M. M., Laref, A., & Li, D. (2021). Designing of highly active g-C3N4/Ni-ZnO photocatalyst nanocomposite for the disinfection and degradation of the organic dye under sunlight radiations. Colloids and Surfaces A: Physicochemical and Engineering Aspects, 614, 126176. https://doi.org/10.1016/j.colsurfa.2021.126176
- Rajeswari Yogamalar, N., & Chandra Bose, A. (2011). Tuning the aspect ratio of hydrothermally grown ZnO by choice of precursor. Journal of Solid State Chemistry, 184(1), 12–20. https://doi.org/10.1016/j.jssc.2010.10.024
- Rella, R., Spadavecchia, J., Manera, M. G., Capone, S., Taurino, A., Martino, M., Caricato, A. P., & Tunno, T. (2007). Acetone and ethanol solid-state gas sensors based on TiO2 nanoparticles thin film deposited by matrix assisted pulsed laser evaporation. Sensors and Actuators B: Chemical, 127(2), 426–431. https://doi.org/10.1016/j.snb.2007.04.048
- Saasa, V., Malwela, T., Beukes, M., Mokgotho, M., Liu, C. P., & Mwakikunga, B. (2018). Sensing technologies for detection of acetone in human breath for diabetes diagnosis and monitoring. Diagnostics, 8(1), 12. https://doi.org/10.3390/diagnostics8020012
- Sonker, R. K., Sabhajeet, S. R., Singh, S., & Yadav, B. C. (2015). Synthesis of ZnO nanopetals and its application as NO2 gas sensor. Materials Letters, 152(2), 189–191. https://doi.org/10.1016/j.matlet.2015.03.112
- Sridhar, R., Lakshminarayanan, R., Madhaiyan, K., Amutha Barathi, V., Lim, K. H. C., & Ramakrishna, S. (2015). Electrosprayed nanoparticles and electrospun nanofibers based on natural materials: Applications in tissue regeneration, drug delivery and pharmaceuticals. Chemical Society Reviews, 44(3), 790–814. https://doi.org/10.1039/c4cs00226a
- Umeh, C., Gupta, R. C., Gupta, R., Kaur, H., Kazourra, S., Maguwudze, S., Torbela, A., & Saigal, S. (2021). Acetone ingestion resulting in cardiac arrest and death. Cureus, 13(10), e18466. https://doi.org/10.7759/cureus.18466
- Wang, W., Li, Z., Zheng, W., Huang, H., Wang, C., & Sun, J. (2010). Cr2O3-sensitized ZnO electrospun nanofibers based ethanol detectors. Sensors and Actuators B: Chemical, 143(2), 754–758. https://doi.org/10.1016/j.snb.2009.10.016
- Yan, X., Li, H., & Su, X. (2018). Review of optical sensors for pesticides. TrAC - Trends in Analytical Chemistry, 103, 1–20. https://doi.org/10.1016/j.trac.2018.03.004
- Zhang, G. H., Deng, X. Y., Wang, P. Y., Wang, X. L., Chen, Y., Ma, H. L., & Gengzang, D. J. (2016). Morphology controlled syntheses of Cr doped ZnO single-crystal nanorods for acetone gas sensor. Materials Letters, 165, 83–86. https://doi.org/10.1016/j.matlet.2015.11.112
- Zhang, L., Dong, B., Xu, L., Zhang, X., Chen, J., Sun, X., Xu, H., Zhang, T., Bai, X., Zhang, S., & Song, H. (2017). Three-dimensional ordered ZnO–Fe3O4 inverse opal gas sensor toward trace concentration acetone detection. Sensors and Actuators B: Chemical, 252, 367–374. https://doi.org/10.1016/j.snb.2017.05.167
- Zhang, X., Dong, Z., Liu, S., Shi, Y., Dong, Y., & Feng, W. (2017). Maize straw-templated hierarchical porous ZnO:Ni with enhanced acetone gas sensing properties. Sensors and Actuators B: Chemical, 243, 1224–1230. https://doi.org/10.1016/j.snb.2016.12.076
- Zhao, C., Tan, A., Pastorin, G., & Ho, H. K. (2013). Nanomaterial scaffolds for stem cell proliferation and differentiation in tissue engineering. Biotechnology Advances, 31(5), 654–668. https://doi.org/10.1016/j.biotechadv.2012.08.001
- Zhu, L., Li, Y., & Zeng, W. (2017). Enhanced ethanol sensing and mechanism of Cr-doped ZnO nanorods: Experimental and computational study. Ceramics International, 43(17), 14873–14879. https://doi.org/10.1016/j.ceramint.2017.08.003