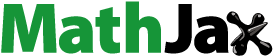
Abstract
This paper introduces a compact, circularly polarized spidron-fractal slot antenna with a rectangular island. The reflection-coefficient |S11| of the proposed antenna covers the band from 7 GHz to 10 GHz. The proposed antenna is circularly polarized with an axial-ratio (AR) bandwidth that extends from 7 GHz to 10 GHz. It offers simultaneous dual circular polarization (RHCP and LHCP). The gain of the proposed antenna varies between 4 dBi and 6.9 dBi. The simulated efficiency reaches 97%. The overall dimensions of the antenna are 19.7 x 23 x 0.508 mm3 (0.55λ0 x 0.65λ0 x 0.014λ0), making it suitable for CubeSats with limited surface area. The proposed antenna can be employed for both the X-band satellite downlink (from 7.25 GHz to 7.75 GHz) and uplink (from 7.9 GHz to 8.4 GHz) frequency bands. Additionally, the antenna can be utilized in small satellite Synthetic-Aperture-Radar (SAR) systems operating from 9 GHz to 10 GHz, military applications, and RFID tag tracking-equipment. A prototype of the proposed antenna has been fabricated and then measured using Vector-Network-Analyzer (VNA) and inside an Anechoic chamber. The measurement results of the proposed antenna are in excellent match with the simulated results.
REVIEW EDITOR:
1. Introduction
X-band technology is broadly used in numerous applications owing to the high rate of data transmitting, short-range features, and large bandwidths. However, designing X-band antennas to meet the specific requirements of these applications remains a challenge (Arnaud et al., Citation2020; El Khamlichi et al., Citation2021; Genovesi & Dicandia, Citation2022; Leszkowska et al., Citation2021; Varun Yadav et al., Citation2020). There is a growing need for compact, lightweight antennas that can be easily integrated into modern communication systems. Planar antennas, which are low-cost, low-profile, and easy to fabricate, have gained extensive utilization in advanced satellite and wireless communication devices (Adhitya et al., Citation2019; Imbraile, Citation2012). However, planar antennas have some significant drawbacks, such as small bandwidth, low power handling capacity, and low gain (Adhitya et al., Citation2019). Therefore, achieving wide bandwidth, acceptable gain, and small antenna size is a challenging goal. Satellites have become increasingly important in many applications, such as communication, oceanography, agriculture, astronomy, positioning, and surveillance (May, Citation2017; Zyl, Citation2013). Satellites are classified according to their size, with the smallest being femto-satellites, which have a mass smaller than 0.1 Kg (Xue et al., Citation2008). The design of satellite antennas differs from that of antennas used in other applications. The design constraints imposed by the satellite on size, shape, and weight are also critical factors to consider (Hwang, Citation1992). Modern satellite communication systems require very small, low-cost, low-profile, and high-gain antennas. Planar antennas are particularly desired for usage in satellite communication systems because of their low profile, lightweight, ease of fabrication, robustness, ability to be easily installed on various mounting surfaces and ability to be compatible with microwave-monolith-integrated-circuits (MMICs) besides optoelectronics-integrated-circuits (OEICs) technologies. Planar antennas are also very adaptable in terms of resonance frequency, polarizations, impedance and radiation patterns, making them an attractive option for many applications (Adhitya et al., Citation2019; Imbraile, Citation2012; Khac et al., Citation2018). Circular polarization is a highly advantageous property that finds widespread applications in various fields. One of the primary advantages of circular polarization is its ability to overcome the unwanted effects caused by multipath interference. This is due to its inherent property of rejecting signals with an opposite-handedness polarization, which makes it highly effective in mitigating the interference caused by reflected signals (Dicandia et al., Citation2017). In addition to its interference-rejection capabilities, circularly polarized (CP) signals are highly robust in communication links. Unlike linearly polarized radiators, CP signals are not affected by polarization misalignment between transmitting and receiving antennas. This makes them highly advantageous in situations where the relative orientation between the antennas is variable, which is the case in satellite communications (Lin & Wong, Citation2017).
Various antenna designs for X-band satellite applications were investigated. The first antenna, introduced in (Arnaud et al., Citation2020), is a telemetry antenna that is compact and has dual circular polarization. It covers a bandwidth that extends from 8.025 GHz to 8.4 GHz, with a 3-dB axial-ratio (AR) bandwidth of 370 MHz and has a maximum gain of 5 dBi. The antenna is considered relatively large, having a diameter equals to 238 mm (6.52λ0) and a height equals to 185 mm (5.13λ0), compared to its realized gain. The second antenna, introduced in (Leszkowska et al., Citation2021), is a superstrate antenna suitable for CubeSat applications. It has a bandwidth that extends from 8 GHz to 9.7 GHz, a 3-dB axial-ratio bandwidth of 580 MHz which doesn’t achieve circular polarization the whole bandwidth of antenna and a maximum gain of 14 dBi. The antenna size is 62 x 62 x 22 mm3 (1.77λ0 x 1.77λ0 x 0.63λ0), which is considered a large volume especially due to the large antenna thickness. The third antenna, introduced in (Fouany et al., Citation2017), is a telemetry circularly polarized antenna designed for CubeSats. It has a bandwidth that extends from 8 GHz to 8.4 GHz, a 3-dB AR-bandwidth of 400 MHz, and a maximum gain of 5 dBi. The antenna size is 100 x 100 x 15 mm3 (2.74λ0 x 2.74λ0 x 0.41λ0), which is considered a relatively large size compared to the achieved gain. The fourth antenna introduced in (Genovesi & Dicandia, Citation2022), is a metasurface (MS) based on 4 x 4 loop elements for X-band satellite communications. It has a bandwidth that extends from 7 GHz to 8.9 GHz, a 3-dB AR-bandwidth of 1.15 GHz, and a maximum gain of 8.6 dBi. The antenna has a diameter equals to 50 mm (1.3λ0) and a height equals to 5.25 mm (0.137λ0). The fifth antenna, introduced in (El Khamlichi et al., Citation2021), is a broadband substrate integrated waveguide antenna for X-band SAR applications. It has a bandwidth that extends from 9.4 GHz to 10.5 GHz, does not achieve circular polarization, and achieves a maximum gain of 9 dBi. The antenna size is 59 x 14 x 0.76 mm3 (1.96λ0 x 0.46λ0 x 0.025λ0). The sixth antenna, introduced in (Anim et al., Citation2021), is a broadband planar antenna for X-band SAR onboard small satellites. It has a bandwidth of 9.01 GHz to 10.2 GHz, does not achieve circular polarization, and achieves a maximum gain of 8 dBi. The antenna size is 14 x 14 x 3.986 mm3 (0.45λ0 x 0.45λ0 x 0.126λ0). The research gap lies in the fact that none of the antennas investigated in this literature survey have been able to achieve a sufficiently wide bandwidth and wide axial-ratio bandwidth to cater to the X-band satellite uplink, downlink, and SAR applications. Moreover, none of the antennas specifically designed for SAR applications have been successful in achieving circular polarization. Furthermore, a significant drawback of the existing designs is their large physical size, which limits their practicality, particularly in scenarios where surface area is limited such as small satellite applications. Consequently, the development of compact antenna designs capable of operating in multiple applications has become an urgent and crucial requirement.
This paper aims to focus on designing a wideband antenna that operates in the X-band. The proposed antenna design is required to cover the X-band satellite uplink from 7.25 GHz to 7.75 GHz and downlink from 7.9 GHz to 8.4 GHz for satellite communication application. The proposed antenna design is also required to cover the band extending from 9 GHz to 10 GHz for X-Band Synthetic-Aperture-Radar (SAR) application, making the antenna able to serve multiple applications. The proposed antenna is designed to achieve circular polarization all over its operating band with a wide axial-ratio (AR) bandwidth compared to the previously mentioned designs. The proposed antenna is designed to cover the band extending from 7.25 GHz to 10 GHz while achieving circular polarization in this band. It is also required to achieve gain greater than or equal 4 dBi all over the desired band, with a smaller size compared to the previously mentioned designs.
To mitigate the limitations of planar antennas, meet the specific needs of satellite communication systems, and align with the proposed requirements, it is essential for the proposed planar antenna to be compact, provide wide bandwidth, and support circular polarization. Recently, there has been growing interest in the field of fractal antenna theory. Fractal antennas are known for their compact size, lightweight construction, conformal shapes, broad bandwidths, and high gains. These antennas are based on intricate shapes that exhibit self-similarity or self-affinity in their geometric structure, such as the Spidron-fractal (Karmakar, Citation2020; Kwon et al., Citation2017; Nguyen Thi et al., Citation2013). The embedded islands inside slot antennas serve to enhance the antenna’s bandwidth and circular polarization by widening the axial-ratio (AR) bandwidth. Additionally, the embedded islands can enhance the antenna’s gain and directivity by providing additional resonant structures. Overall, an embedded island contribute to the improved performance and functionality of slot antennas (Abed et al., Citation2020; Anantrasirichai et al., Citation2005; Kumar et al., Citation2019; Trinh-Van et al., Citation2019).
This paper proposes a single element fractal slot antenna with a rectangular island fed by a conventional rectangular feedline from the bottom layer of the antenna. The proposed antenna provides a wide bandwidth covering the X-band uplink and downlink for satellite communication applications, along with Synthetic-Aperture-Radar (SAR) application. This research work offers several advantages due to the implementation of the spidron-fractal slot and the rectangular island. These include a wide bandwidth, the ability to achieve circular polarization with a significant axial-ratio (AR) bandwidth, and a compact antenna size. However, it is important to note a couple of limitations associated with this research work. Firstly, the complexity of the spidron-fractal structure can pose a challenge in design and manufacturing. Secondly, while the initial iterations of the spidron-fractal antenna design may yield substantial improvements in performance, such as increased bandwidth and compactness, the subsequent iterations may result in diminishing incremental enhancements that may not justify the added complexity (Anantrasirichai et al., Citation2005; M & Boopathi Rani, Citation2020). The proposed antenna provides a wide axial-ratio (AR) bandwidth covering the whole bandwidth of the antenna. The design procedure is presented in section 1. Results and analysis are introduced in section 2. The proposed antenna design and experimental results are given in section 3. Final assessment and comparison with recent X-band antenna designs are introduced in section 4. Conclusion is presented in Section 5.
2. Design procedure
The proposed antenna is composed of three layers. The top layer where a spidron-fractal slot and the rectangular island take place. The feedline exists in the bottom layer. The substrate layer exists in between as shown in . The substrate used is Rogers-RT-Duroid-5880 with dielectric constant, of 2.2, loss tangent of 0.0009 and thickness of 0.508 mm. The overall length, L and width W of the proposed antenna are selected to be slightly greater than the height of the spidron-fractal slot as shown in . The design procedure is presented in the following three steps.
2.1. Step 1: spidron-fractal slot design
A spidron-fractal is composed of a number of right-angle triangles starting with a large triangle with height Hs and then reduced in size by a common factor so that the height of the next triangle is
and so on until reaching the nth triangle whose height would be
as shown in (Kwon et al., Citation2017). Each triangle is then rotated backward towards the larger triangle by a fixed angle, θ to form the shape of a spidron as shown in . The first design step of the antenna is cutting a spidron-fractal slot of height Hs centered in the top layer of the antenna at distances Sx and Sy from the left and bottom edges of the top layer respectively as shown in . The height of the spidron slot can be derived starting from the equation of the slot antenna width (Balanis, Citation2016; Moitra et al., Citation2013; Xu-Bao et al., Citation2012). The derivation process is outlined as follows:
(1)
(1)
(2)
(2)
(3)
(3)
2.2. Step 2: feedline configuration
The second step is the microstrip feedline configuration. The slot antenna is fed using a microstrip feedline at the bottom layer as shown in . The length and width of the feedline are Lf and Wf respectively. The feedline is initially positioned in the middle of the antenna at a distance of FL from the left side of the antenna as shown in . In the next section parametric analysis is performed on the feed location, FL to optimize its location. optimum feed location results are introduced in the next section.
2.3. Step 3: rectangular island integration
The third step is placing a rectangular island centered in the middle of the slot. The function of the embedded island is achieving a large Bandwidth along with wideband circular polarization (large 3-dB AR-bandwidth). The rectangular island is placed at a distance Rp from the left side of the spidron-fractal slot and Rg from the bottom side of the spidron-fractal slot as shown in . The embedded rectangular island has a height of LR and a width of WR. For the rectangular island to fit within the spidron-fractal slot, it is important to choose the length LR and width WR to be less than by a specific percentage. The initial values of LR and WR are approximately equal to
Parametric analyses are then performed on the dimensions of the rectangular island, LR and WR along with its horizontal position Rp. The parametric analysis is performed to achieve the widest required reflection-coefficient |S11| bandwidth along with the widest required axial-ratio (AR) bandwidth. Parametric analyses are performed, and optimum results are introduced in the next section.
3. Results and analysis
The proposed design is then simulated and analyzed using CST. The dimensions of the spidron-fractal slot opening are calculated using EquationEquation (3)(3)
(3) at angle, θ equals 35 degrees. This section consists of two parts. Part ‘a’ studies the spidron-fractal slot antenna with no island (SFSA-NI) along with deciding the optimum feed location. Part ‘b’ studies the spidron-fractal slot antenna with embedded rectangular island (SFSA-RI). In part ‘b’ parametric analyses are then performed on the embedded square island shown in , to achieve the widest reflection-coefficient |S11| bandwidth along with the widest axial-ratio (AR) bandwidth. The parametric analyses are performed in three steps. The first step is performed on the height of the embedded rectangular island, LR. The second step is performed on the width of the embedded rectangular island, WR. The third step is performed on the position of the embedded rectangular island, Rp.
3.1. Spidron-fractal slot antenna with no island (SFSA-NI)
First the spidron-fractal slot antenna with no island (SFSA-NI), shown in , is simulated at different values of feed location, FL as shown to study its effect. The value of FL that gives the wider bandwidth and the wider 3-dB AR-bandwidth is then selected. The SFSA-NI antenna is simulated at FL= 8.35 mm, 10.9 mm (centered) and 13.4 mm. shows the reflection-coefficient |S11| at FL= 8.35 mm, 10.9 mm and 13.4 mm where the bandwidth is equal to 900 MHz extending from 7.1 GHz to 8 GHz, 1 GHz extending from 7.4 GHz to 8.4 GHz and 1.4 GHz extending from 10.1 GHz to 11.5 GHz respectively. shows the axial-ratio (AR) at FL= 8.35 mm, 10.9 mm and 13.4 mm where the SFSA-NI antenna achieves circular polarization only at FL=13.4 mm with 3-dB AR-bandwidth of 600 MHz extending from 8 GHz to 8.6 GHz. The selected value of the feed location FL is 13.4 mm where it achieves circular polarization along with the widest bandwidth. The optimized dimensions of the SFSA-NI antenna are given in . The SFSA-NI antenna is still not covering the whole required band, the 3-dB AR-bandwidth is not wide enough to achieve circular polarization for the whole required band. In addition, there is no common – 10-dB reflection-coefficient |S11| and 3-dB AR-bandwidth. The – 10-dB reflection-coefficient |S11| bandwidth and the 3-dB AR-bandwidth can be aligned and increased using the embedded rectangular island. In the upcoming subsection, an embedded rectangular island will be placed within the spidron-fractal slot to control the reflection-coefficient |S11| and increase the bandwidth by creating additional resonant modes. Also, to control and improve circular polarization by increasing the axial-ratio (AR) bandwidth. This can be performed by adjusting the dimensions and location of the rectangular embedded island.
Table 1. optimized dimensions of SFSA-NI antenna.
3.2. Spidron-fractal slot antenna with rectangular island (SFSA-RI)
The embedded rectangular island with width, WR and length LR is then placed centered in the middle of the spidron-fractal slot at a distance Rp from the left edge of the spidron-fractal slot as shown in . The optimized dimensions of the SFSA-RI antenna are given in . Three steps of parametric analysis are performed to study the effect of LR, WR, and RP on the reflection-coefficient |S11| bandwidth and the axial-ratio (AR) bandwidth. The length, LR and the width, WR should be chosen to be smaller than by a percentage that ensures the rectangular island fits within the spidron slot. To begin the parametric analysis, the starting values of LR and WR are initially selected to be approximately equal to
Table 2. Optimized dimensions of SFSA-RI antenna.
3.3. Step 1: studying the effect of length LR
In this step, a parametric study is performed on LR while all the other parameters are kept constant. The SFSA-RI antenna shown in is simulated at different values of LR. Any variation in the length of the embedded rectangular island, LR will change the gap length, Rg as shown in . The reflection-coefficient |S11| and the axial-ratio (AR) curves for different values of LR are shown in and respectively. It is shown in that the reflection-coefficient |S11| bandwidth at LR = 3.25 mm, 4.25 mm, 5.25 mm, and 6.25 is 1.3 GHz (9.5 GHz - 10.8 GHz), 1.52 GHz (9.33 GHz - 10.85 GHz), 3.4 GHz (7.2 GHz - 10.6 GHz) and 2.7 GHz (6.7 GHz - 9.4 GHz) respectively. shows that the 3-dB AR-bandwidth at LR = 3.25 mm, 4.25 mm, 5.25 mm, and 6.25 is 600 MHz (7.6 GHz – 8.2 GHz), 800 MHz (7.4 GHz - 8.2 GHz), 1 GHz (7.3 GHz - 8.3 GHz) and 2.3 GHz (7.52 GHz- 9.82 GHz) respectively. LR = 6.25 mm achieves the largest – 10-dB reflection-coefficient |S11| bandwidth which is common with the largest 3-dB AR-bandwidth, reaching 1.88 GHz. Therefore, the selected length of the rectangular island, LR, is 6.25 mm. In the upcoming step parametric study is performed on WR for further improvement in the reflection-coefficient |S11| bandwidth and the 3-dB AR-bandwidth.
3.4. Step 2: studying the effect of length WR
After updating the value of LR, a parametric study is performed on WR while all the other parameters are kept constant. The SFSA-RI antenna shown in is simulated at different values of WR. The reflection-coefficient |S11| and the axial-ratio (AR) curves for different values of LR are shown in and respectively. It is shown in that the reflection-coefficient |S11| bandwidth at WR = 6.87 mm, 7.87 mm, 8.87 mm, and 9.87 is 2.5 GHz (6.8 GHz-9.3 GHz), 3.1 GHz (6.6 GHz-9.7 GHz), 3.2 GHz (6.7 GHz-9.9 GHz) and 3.7 GHz (6.2 GHz-9.9 GHz) respectively. shows that the 3-dB AR-bandwidth at WR = 6.87 mm, 7.87 mm, 8.87 mm, and 9.87 is 2.2 GHz (7.6 GHz-9.8 GHz), 2.8 GHz (7 GHz-9.8 GHz), 1 GHz (6.8 GHz-7.8 GHz) and 1 GHz (6 GHz-7 GHz) respectively. LR = 7.87 mm achieves the largest – 10-dB reflection-coefficient |S11| bandwidth that is common with the largest 3-dB (AR) bandwidth, reaching 2.7 GHz with an improvement of 43.6% compared to step 1. Therefore, the selected width of the rectangular island, WR, is 6.25 mm. In the upcoming step parametric study is performed on Rp for further improvement in the reflection-coefficient |S11| bandwidth and the 3-dB AR-bandwidth.
3.5. Step 3: studying the effect of length Rp
After attuning the length, LR, and the width, WR of the rectangular island to achieve the largest common – 10-dB reflection-coefficient |S11| and 3-dB AR-bandwidth, a parametric study is performed on the rectangular island position Rp, while all the other parameters are kept constant. The SFSA-RI antenna shown in is simulated at different values of Rp. The reflection-coefficient |S11| and the axial-ratio (AR) curves for different values of Rp are shown in and respectively. It is shown in that the reflection-coefficient |S11| bandwidth at Rp = 2.94 mm, 3.94 mm, and 4.94 mm is 3.2 GHz (6.5 GHz-9.7 GHz), 2.8 GHz (6.7 GHz-9.5 GHz) and 3.2 GHz (6.9 GHz-10.1 GHz) respectively. shows that the 3-dB AR-bandwidth at Rp = 2.94 mm, 3.94 mm, and 4.94 mm is 2.2 GHz (7.6 GHz-9.8 GHz), 2.8 GHz (7 GHz-9.8 GHz) and 3.7 GHz (7 GHz-10.7 GHz) respectively. Rp = 4.94 mm achieves the largest – 10-dB reflection-coefficient |S11| bandwidth that is common with the largest 3-dB AR-bandwidth, reaching 3 GHz with an improvement of 11.11% compared to step 2. Therefore, the selected position of the rectangular island, Rp is 4.94 mm. The optimized dimensions of the SFSA-NI antenna are given in .
4. Proposed design and experimental results
This section provides a detailed description of the final design of the proposed spidron-fractal slot antenna with an embedded rectangular island (SFSA-RI). The proposed antenna was fabricated using RT/Duroid 5880 substrate with dielectric constant, εr = 2.2, low loss tangent tan(δ) = 0.0009, and height h = 0.508 mm as shown in . The antenna’s simulated radiation patterns and antenna parameters are presented, as well as the measurements taken from a fabricated prototype of the antenna. The final dimensions of the antenna are given in . The reflection-coefficient |S11| of the antenna was measured using ROHDE & SCHARZ 20 Vector Network Analyzer (VNA) with measurement range up to 20 GHz. The radiation pattern and other antenna parameters were then measured in SATEMO Anechoic chamber as shown in . Simulated and measured radiation patterns and antenna parameters of the SFSA-RI antenna were analyzed and compared. The simulated surface currents at 7.5 GHz, 8.15 GHz and 9 GHz are shown in , and respectively. The surface current distribution of an antenna is an important parameter that determines its radiation characteristics. , and , are provided to describe the surface current distribution at phase angles of 0, 90, 180, and 270 degrees, respectively. The maximum surface currents at 7.5 GHz, 8.15 GHz and 9 GHz are 114 A/m, 103 A/m, and 90 A/m respectively. At 7.5 GHz, 8.15 GHz, and 9 GHz, it is observed that the surface current distributions at 0 and 90 degrees are equal in magnitude and opposite in phase to that at 180 and 270 degrees. The proposed antenna can excite LHCP and RHCP waves. The proposed antenna is a bidirectional simultaneous dual circularly polarized antenna, as it is able to generate a left-hand circular polarization (LHCP) in the positive z direction, whereas a right-hand circular polarization (RHCP) is generated in the negative z direction at the same time (Huang et al., Citation2019; Mohammadi et al., Citation2013). The simulated and measured LHCP and RHCP radiation patterns at 7.5 GHz, 8.15 GHz and 9 GHz are shown in , and respectively. The simulated and measured radiation patterns and antenna parameters of the SFSA-RI antenna are analyzed and compared. The radiation patterns E-plane (phi = 0) and H-plane (phi = 90) at frequencies of 7.5 GHz, 8.15 GHz and 9 GHz for the simulated and fabricated antennas are shown in , respectively. The simulated and measured reflection-coefficients |S11| are shown in with -10 dB reflection-coefficient |S11| bandwidths of 3.2 GHz extending 6.9 GHz to 10.1 GHz and 4.2 GHz extending from 6.8 GHz to 11 GHz, respectively. The simulated and measured axial-ratios (AR) are shown in indicating that the antenna is circularly polarized in the required band. The simulated and measured AR 3-dB bandwidths are 3.7 GHz extending 7 GHz to 10.7 GHz and 3 GHz extending from 7 GHz to 10 GHz, respectively. The simulated and measured gains are shown in with maximum gains of 5.5 dBi and 6.8 dBi, respectively. The simulated and measured total efficiencies are shown in with maximum efficiencies of 97.8% and 98%, respectively. This indicates that the proposed antenna design is well optimized and capable of achieving excellent performance in terms of efficient use of the available power. The results demonstrate that the measured parameters and radiation patterns of the fabricated antenna match the simulation results, achieving the desired design requirements.
Figure 15. Simulated surface current distribution at 7.5 GHz of the SFSA-RI antenna with embedded island at phase: (a) 0°, (b) 90°, (c) 180°, (d) 270°.

Figure 16. Simulated surface current distribution at 8.15 GHz of the SFSA-RI antenna with embedded island at phase: (a) 0°, (b) 90°, (c) 180°, (d) 270°.

Figure 17. Simulated surface current distribution at 9 GHz of the SFSA-RI antenna with embedded island at phase: (a) 0°, (b) 90°, (c) 180°, (d) 270°.

Figure 18. Simulated and measured LHCP and RHCP, radiation patterns at 7.5 GHz: (a) E-plane (phi = 0°), (b) H-plane (phi = 90°).

Figure 19. Simulated and measured LHCP and RHCP, radiation patterns at 8.15 GHz: (a) E-plane (phi = 0°), (b) H-plane (phi = 90°).

Figure 20. Simulated and measured LHCP and RHCP, radiation patterns at 9 GHz: (a) E-plane (phi = 0°), (b) H-plane (phi = 90°).

5. Final assessment and comparison with recent X-band antenna designs
This section presents a comprehensive comparison of the proposed, spidron-fractal slot antenna with rectangular island (SFSA-RI), with several recent X-band antenna designs as given in . The proposed design shows remarkable performance in terms of bandwidth, small size, and circular polarization in the required band. The table provides a detailed comparison of different antenna designs in terms of bandwidth, AR 3-dB bandwidth, maximum gain, and antenna size. It is evident that the proposed design outperforms other designs in terms of bandwidth, with a bandwidth of 4.2 GHz extending from 6.8 GHz to 11 GHz, which is exceptionally wide compared to the other designs. Moreover, the proposed design achieves a wideband circular polarization with a 3-dB AR of 3 GHz extending from 7 GHz to 10 GHz. The proposed design also demonstrates a small size with dimensions of 19.7 mm x 23 mm x 0.508 mm (0.55λ0 x 0.65λ0 x 0.014λ0), which is significantly smaller than most of the other designs while still achieving a maximum gain of 6.8 dBi. The proposed antenna can be used for multiple applications unlike other designs. The antenna design being proposed is suitable for both the downlink (7.25 GHz - 7.75 GHz) and uplink (7.9 GHz - 8.4 GHz) frequency bands in the X-band satellite communication. Furthermore, it can also be used in small satellite Synthetic-Aperture-Radar (SAR) systems that operate within the frequency range of 9 GHz to 10 GHz. The results of our study demonstrate that our proposed X-band antenna design offers superior performance compared to existing designs and has the potential to advance the field of X-band applications significantly.
Table 3. Comparison with most recent designs of X-band antennas.
6. Conclusion
This paper presents the design, simulation, fabrication, and testing of a spidron-fractal slot antenna with embedded rectangular island (SFSA-RI). The paper introduces and demonstrates a method for enhancing the bandwidth, and axial-ratio (AR) of a fractal slot antenna by using an embedded rectangular island. The designed antenna achieved a wide bandwidth of 4.2 GHz, covering the X-band range from 6.8 GHz to 11 GHz. It also exhibited circular polarization with simultaneous dual circular polarization (RHCP and LHCP). The AR 3-dB bandwidth was measured to be 3 GHz, ranging from 7 GHz to 10 GHz. The antenna design is particularly suitable for both the X-band satellite downlink (from 7.25 GHz to 7.75 GHz) and uplink (from 7.9 GHz to 8.4 GHz) frequency bands as well as Synthetic-Aperture-Radar (SAR) on small satellites in the band from 9 GHz to 10 GHz. The proposed antenna realized a maximum gain of 6.8 dBi, with overall size of 19.7 x 23 x 0.508 mm³. The compactness and efficiency of the design are noteworthy, with a total antenna efficiency of 98%. These results show that the proposed antenna design is well-optimized and capable of delivering outstanding performance. The reliability of the design approach was verified through measurements on a fabricated prototype, further validating its effectiveness.
Data availability statement
The data that support the findings of this study are available from the corresponding author, Mostafa Mahmoud Rabie, upon reasonable request.
Disclosure statement
No potential conflict of interest was reported by the author(s).
References
- Abed, A., Singh, M., & Jawad, A. (2020). Investigation of circular polarization technique in Q-slot antenna. International Journal of Microwave and Wireless Technologies, 12(2), 176–182. https://doi.org/10.1017/S1759078719001107
- Adhitya, G., Abishek, V., Sundaram, R., & Jothilakshmi, P. (2019). Design of microstrip antenna for X band satellite communications. International Journal of Research and Analytical Reviews (IJRAR), 6(2), 342–344.
- Anantrasirichai, N., Chanoodhorm, S., Nakasuwan, J., Rakluea, P., & Wakabayashi, T. (2005). Designing rectangular slot loop antenna for WLAN application. In TENCON-2005 IEEE Region 10 Conference.
- Anim, K., Danuor, P., Park, S.-O., & Jung, Y.-B. (2021). High-efficiency broadband planar array antenna with suspended microstrip slab for X-Band SAR onboard small satellites. Sensors, 22(1), 252. https://doi.org/10.3390/s22010252
- Arnaud, E., Dugenet, J., Elis, K., Girardot, A., Guihard, D., Menudier, C., Monediere, T., Roziere, F., & Thevenot, M. (2020). Compact isoflux X-band payload telemetry antenna with simultaneous dual circular polarization for LEO satellite applications. IEEE Antennas and Wireless Propagation Letters, 19(10), 1679–1683. https://doi.org/10.1109/LAWP.2020.3013989
- Balanis, C. A. (2016). Antenna theory: Analysis and design (4th ed.). Hoboken: Wiley.
- Dicandia, F. A., Genovesi, S., & Monorchio, A. (2017). Analysis of the performance enhancement of MIMO systems employing circular polarization. IEEE Transactions on Antennas and Propagation, 65(9), 4824–4835. https://doi.org/10.1109/TAP.2017.2723083
- El Khamlichi, D., Amar Touhami, N., Elhamadi, T., & Ali Ennasar, M. (2021). High-gain and broadband SIW cavity-backed slots antenna for X-band applications. International Journal of Microwave and Wireless Technologies, 13(10), 1078–1085. https://doi.org/10.1017/S1759078721000015
- Fouany, J., Thevenot, M., Arnaud, E., Torres, F., Menudier, C., Monediere, T., & Elis, K. (2017). New concept of telemetry X-band circularly polarized antenna payload for CubeSat. IEEE Antennas and Wireless Propagation Letters, 16, 2987–2991. https://doi.org/10.1109/LAWP.2017.2756565
- Genovesi, S., & Dicandia, F. A. (2022). Characteristic modes analysis of a near-field polarization-conversion metasurface for the design of a wideband circularly polarized X-band antenna. IEEE Access. 10, 88932–88940. https://doi.org/10.1109/ACCESS.2022.3200303
- Huang, T., Liu, G.-B., Zhang, H.-F., & Zeng, L. (2019). A new adjustable frequency waveguide circularly polarized antenna based on the solid-state plasma. Applied Physics A, 125(9), 659–660. https://doi.org/10.1007/s00339-019-2965-2
- Hwang, Y. (1992). Satellite antennas. Proceedings of the IEEE, 80(1), 183–193. https://doi.org/10.1109/5.119577
- Imbraile, W. (2012). S.Gao and L.Boccia, Space Antenna Handbook. Wiley.
- Karmakar, A. (2020). Fractal antennas and arrays: a review and recent developments. International Journal of Microwave and Wireless Technologies, 13(2), 173–197. https://doi.org/10.1017/S1759078720000963
- Khac, K. N., Duy Phong, N., Manh1Tuan Anh Le Trong, L. H., Huu, H. L., Hien, B. T. T., & Chien, D. N. (2018). A design of circularly polarized array antenna forX-Band cubesat satellite communication [Paper presentation]. In international conference on advanced technologies for communications, Ho Chi Minh City, Vietnam.
- Kumar, B., Shukla, B. K., Somkuwar, A., & Meena, O. P. (2019). Analysis of hexagonal wide slot antenna with parasitic element for wireless application. Progress in Electromagnetics Research C, 94, 145–159. https://doi.org/10.2528/PIERC19032710
- Kwon, O., Park, W., Lee, S., Lee, J., Park, Y., & Hwang, K. (2017). 3D-printed super-wideband spidron fractal cube antenna with laminated copper. Applied Sciences MDPI, 7(10), 979. https://doi.org/10.3390/app7100979
- Leszkowska, L., Rzymowski, M., Nyka, K., & Kulas, L. (2021). High-gain compact circularly polarized X-band superstrate antenna for CubeSat applications. IEEE Antennas and Wireless Propagation Letters, 20(11), 2090–2094. https://doi.org/10.1109/LAWP.2021.3076673
- Lin, W., & Wong, H. (2017). Wideband circular-polarization recon_gurable antenna with L-shaped feeding probes. IEEE Antennas and Wireless Propagation Letters, 16, 2114–2117. https://doi.org/10.1109/LAWP.2017.2699289
- M, R. C., & Boopathi Rani, R. (2020). A compendious review on fractal antenna geometries in wireless communication [Paper presentation]. In 2020 International Conference on Inventive Computation Technologies (ICICT), Coimbatore, India.
- May, S. (2017). Nasa, 7 August [Online]. https://www.nasa.gov/audience/forstudents/5-8/features/nasa-knows/what-is-a-satellite-58.html.
- Mohammadi, S., Nourinia, J., Ghobadi, C., Pourahmadazar, J., & Shokri, M. (2013). Compact broadband circularly polarized slot antenna using two linked elliptical slots for C-band applications. IEEE Antennas and Wireless Propagation Letters, 12, 1094–1097. https://doi.org/10.1109/LAWP.2013.2280457
- Moitra, S., Kumar Mukhopadhyay, A., & Kumar Bhattacharjee, A. (2013). Ku-band substrate integrated waveguide (SIW) slot array antenna for next generation networks. Global Journal of Computer Science and Technology Network, Web & Security, 13(5), 11–16.
- Nguyen Thi, T., Hwang, K. C., & Kim, H. B. (2013). Dual-band circularly-polarised Spidron fractal microstrip patch antenna for Ku-band satellite communication applications. Electronics Letters, 49(7), 444–445. https://doi.org/10.1049/el.2012.2973
- Trinh-Van, S., Yang, Y., Lee, K.-Y., Kim, Y., & Hwang, K. (2019). Bandwidth-enhanced circularly polarized crescent-shaped slot antenna via circular-patch loading. Applied Sciences, 9(6), 1117–1126. https://doi.org/10.3390/app9061117
- Varun Yadav, M., Buadha, S., & Sri, C. S. (2020). Multiple Slot Plannar Antenna for X-Band Satellite Mobile Communication. in IEEE 7th Uttar Pradesh Section International Conference on Electrical, Electronics and Computer Engineering (UPCON).
- Xu-Bao, S., Cao, M-y., Hao, J-j., & Guo, Y-j (2012). A rectangular slot antenna with improved bandwidth. International Journal of Electronics and Communications, 29(15), 456–466.
- Xue, Y., Li, Y., Guang, J., Zhang, X., & Guo, J. (2008). Small satellite remote sensing and applications – history, current and future. International Journal of RemoteSensing, 29(15), 4339–4372. https://doi.org/10.1080/01431160801914945
- Zyl, J. V. (2013). Application of satellite remote sensing data to the monitoring of global resources. In 2012 IEEE technology time machine symposium (TTM). IEEE.