Abstract
Non-equilibrium molecular dynamics (NEMD) simulations were employed to investigate the effect of charged pore-wall of carbon nanotubes (CNTs), which is the model of microporous membranes. The study found that the charged atoms on the CNT pore-wall significantly affect how water molecules orient themselves in the pore through the formation of hydrogen bonds with charged pore-wall atoms. Two different atomic charge arrangements on the pore-wall were investigated: a linear arrangement and a double helix arrangement. The latter resulted in a higher flux when tested at higher applied pressure. Additionally, the fluxes in charged pore-wall models were higher than that in uncharged pore-wall models. It is concluded that optimizing charge arrangements could enhance water permeation, which holds potential for improving conventional RO membranes.
1. Introduction
The reverse osmosis (RO) membranes utilized for seawater desalination or water treatment necessitate high water permeability and effective salt rejection. The efficacy of RO membranes has been attained through material optimization, originating in cellulose acetate and aromatic polyamide membranes developing in the 1960s. For further enhanced membrane efficiency, however, developing RO membranes should be based on novel membrane materials or separation mechanisms different from that in conventional RO membranes.
From this perspective, membrane materials designed with diverse nanostructures have garnered significant attention. For instance, two-dimensional layered compounds such as graphene oxide (GO) have exhibited commendable separation efficacy and robust resistance to fouling (Lin et al. Citation2015; Liu et al. Citation2017; Thebo et al. Citation2018; Wu et al. Citation2019; Zhang et al. Citation2019; Su et al. Citation2020). Thebo et al. (Citation2018) addressed durability concerns by utilizing tannic acid and theanine amino acids as both reducing and crosslinking agents, achieving a noteworthy water permeability of 10,000 L m−2 h−1 bar−1 with a rejection nearing 100%. They also suggested the possibility of synthesizing a membrane with distinct organic dye separation properties.
Molecular dynamics (MD) simulations aid in evaluating membrane performance in ideal configurations (Shaikh et al. Citation2015; Nagumo et al. Citation2016; Takaba et al. Citation2017; Yoshioka et al. Citation2018). In the assessment by Lin and Grossman (Citation2015), the water permeability and salt rejection capabilities were investigated through MD. Despite the GO sheet being a monolayer, their findings revealed that the pore structure of GO is conducive to separation due to its low epoxy/hydroxyl ratio, low oxygen ratio, and elevated reduction temperature. Their work demonstrated the synthesis of a highly selective (rGO) membrane, achieving an 99% salt removal rate. Wu et al. (Citation2019) investigated a composite membrane, where the GO membrane was enveloped with a porous polymer and sandwiched between hollow fibers to counteract separation deterioration caused by peeling due to GO membrane swelling. Their findings suggested a higher rejection of 97–98%.
Covalent organic frameworks (COFs), structured porous organic compounds, also hold promise as separation membranes (Lin et al. Citation2015; Fan et al. Citation2018; Zhang et al. Citation2018; Zhou et al. Citation2019; Itoh et al. Citation2022). Itoh et al. (Citation2022) demonstrated the creation of a porous structure through COF layering, showcasing rapid water permeation. Moreover, Zhou et al. (Citation2019) evaluated the water permeability and salt rejection using multilayer Ta-Pa-1, a COF, employing non-equilibrium molecular dynamics (NEMD), displaying higher water permeability than conventional nanofiltration membranes and a complete MgCl2 rejection, indicating its potential as a desalination membrane. Fan et al. (Citation2018) developed a COF membrane composed of two-dimensional imine bonds on an alumina support, exhibiting greater water permeability than a typical NF membrane; however, the salt rejection remained a concern at 12%.
Carbon nanotubes (CNTs), fashioned from ordinary graphite with one-dimensional nanopores, exhibit potential not only in water treatment membranes but also in filters and gas adsorbents (Dresselhaus et al. Citation2001; Meyyappan Citation2005; Sianipar et al. Citation2017; Rashed et al. Citation2021; Rathinavel et al. Citation2021). Though forming CNTs into a membrane poses challenges, their water permeability has been theoretically assessed using MD (Hummer et al. Citation2001; Kalra et al. Citation2003; Majumder et al. Citation2005; Corry Citation2008; Araki et al. Citation2015). Majumder et al. (Citation2005) reported that a typical RO membrane, a polyamide membrane infused with CNTs, presents high water permeability and effective salt rejection due to the hydrophobic nature of CNT membranes, showcasing a distinct permeation mechanism from traditional hydrophilic RO membranes, featuring characteristics akin to single-line diffusion and surface selectivity based on interactions with walls and surface hydrophobicity. Notably, CNTs with a diameter of less than 0.6 nm exhibit high water permeability and salt rejection properties (Corry Citation2008), positioning CNTs as a burgeoning separation material.
To study membrane permeation phenomena using MD, maintaining the pressure difference, the driving force, is essential. Non-equilibrium molecular dynamics methods have been used in the study of the dynamics of permeation phenomena in both liquids (Takaba et al. Citation2007; Higuchi et al. Citation2022) and gases (Yoshioka et al. Citation2001; Hirosawa, Miyagawa, et al. Citation2023; Hirosawa, Watanabe, et al. Citation2023). Evaluations of CNTs’ water permeability and salt rejection attributes in various structures, such as multiwalled CNTs, different pore sizes, and modifications in functional groups, have been conducted using NEMD (Corry Citation2011; Nicholls et al. Citation2012; Chan et al. Citation2013; Thomas and Corry Citation2016; Rizzuto et al. Citation2018). In these studies, CNTs are used as uncharged, hydrophobic materials.
Conversely, in biological membrane water channels like aquaporins, a distinct water permeation mechanism prevails. Research has highlighted the role of amino acid residues, capable of forming hydrogen bonds, distributed appropriately in pores sufficiently large for a single water molecule’s transport, enabling selective and smooth water molecule permeation under osmotic pressure as the driving force (de Groot and Grubmüller Citation2001; Bordin et al. Citation2023). Interactions, such as hydrogen bonding in the membrane, alleviate permeation resistance as water molecules enter the membrane, enabling controlled movement and potentially rapid transportation of water molecules. This distinctive permeation mechanism diverges from conventional polymer-based RO membranes. Hence, the introduction of such a mechanism into nanostructured membranes is anticipated for the development of innovative water-selective separation membranes.
Hence, in this study, a NEMD was employed to evaluate the water permeability of a CNT membrane with partially charged pore-walls. CNTs with an electric charge have distinct features from hydrophobic CNTs, which may lead to unique water permeability similar to biological membranes. By elucidating the relationship between the formation of hydrogen bonds and the permeation rate, it was demonstrated that imparting a partial charge to the wall surface facilitated greater water molecule permeation compared to conventional polymer membranes.
2. Method
2.1. NEMD
MD is a simulation technique that predicts the dynamic behavior of individual atoms by numerically integrating equations of motion. RO separation relies on pressure as the driving force, which challenges MD in managing the constant pressure difference across the membrane. One of the MD methodologies for such permeation phenomena is to introduce piston-like walls in conventional MD. This NEMD was calculated using in-house code. Numerical integration of the Newton equation utilized the velocity Verlet method with a time step of 1.0 fs. All NEMD simulations temperature of system was controlled by the velocity scaling method. The 12-6 Lennard-Jones potential was used to represent the van der Waals interactions with a cut off of 1.0 nm. The parameters in the 12-6 Lennard-Jones potential were determined based on the parameters of the consistent valence force field (CVFF) (Dauber-Osguthorpe et al. Citation1988). The Ewald summation technique (Ewald Citation1921) with an Ewald accuracy parameter of 0.4184 J/mol was used for the summation of the electrostatic interactions. illustrates the unit cell in the fluctuating-wall MD. In this approach, a feed region and a region are situated on opposite sides of a centrally positioned membrane model, encompassing liquid molecules within each area. Simultaneously, a constant pressure is applied to the liquid via each fluctuating wall. By implementing differing pressures in opposite directions on two fluctuating walls, it becomes feasible to conduct a membrane permeation under a steady gradient of pressure (Takaba et al. Citation2007).
2.2. Membrane model
In the study, the membrane model used was single-walled CNTs, both charged and uncharged. These CNTs were (5,5) tubes featuring a pore size of 3.38 Å. This sizing consideration was established based on the narrowest segment of aquaporin, estimated at 3 Å, allowing passage for a single water molecule. To introduce the development of hydrogen bonds as in an aquaporin’s water channels, atomic charges were positioned on the pore walls to enable hydrogen bond formations between the walls and permeating water molecules. The charge configuration involved attributing a positive charge (0.3) or a negative charge (–0.3) to the atom in a CNT wall, ensuring a net charge of 0. These atomic charges are comparable to those of atoms in water molecules. Two distinct models differing in their charge arrangements were considered. The straight model featured linear placement of positive and negative atomic charges along the pore channel, while the double helix model exhibited a spiral pattern of positive and negative atomic charges along the pore channel, which are illustrated in .
Figure 2. Side views of the pore of used two membrane models. (Left) The straight model. (Right) The double helix model. Charged carbon-like atoms are spheres colored blue (shade) or red (light), while the other atoms grey are uncharged (shown by bonding lines).
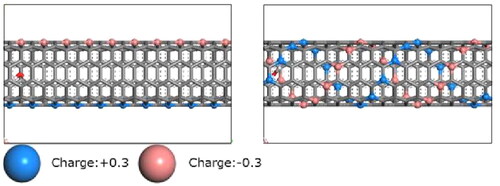
The length of the used CNT was 50 Å. Creating the membrane surface structure involved integrating graphene at both ends of the CNT, thereby constructing a membrane model permitting water molecules to permeate through the pores. Each region, both feed and permeate region, includes 200 water molecules at liquid density. All simulations were employed at room temperature (300 K). The atoms in CNT and graphene were fixed during NEMD calculations. The pressure of the fluctuating-wall on the permeate region was adjusted to 100 atm. Meanwhile, the pressure on the feed region was gradually increased from 100 atm to 1000 atm in increments of 100 atm. The NEMD simulations were conducted up to 400 ps, which was enough time to achieve the steady-state permeations.
3. Results and Discussion
The snapshots of the unit cells after 500 ps of NEMD under a feed region pressure of 500 atm are illustrated in . The illustration exhibits the permeating water molecules from the left feed region to the right, resulting in the reduction in volume on the feed region. These figures also show the sequential entry of water molecules into the pores, diffusing in a single file as expected by the pore size. Furthermore, in the straight model ((a)), water molecules consistently maintain a fixed orientation influenced by charges located on the pore walls. Specifically, one hydrogen atom of the water molecule aligns towards the wall, while the remaining hydrogen atom forms a hydrogen bond with the oxygen atom of the preceding water molecule. Conversely, in the double helix model ((b)), the rotational movement of water molecules through the pore occurs due to the spiral arrangement of charges on the pore wall. For a model where the pore wall is uncharged ((c)), water molecules tend to orient their hydrogen atoms toward the permeation side, fostering hydrogen bonds amongst neighboring water molecules. However, due to the absence of hydrogen bonds with the wall, the orientation of water molecules is marginally more disorderly than observed in the linear or double helix models. It is evident that the presence of atomic charge distribution on the pore walls leads to varied orientations of permeating water molecules.
Figure 3. Side views of the unit cell at 500 ps obtained by NEMD. The snap shots for (a) the straight model, (b) the double helix model, and (c) the uncharged model.
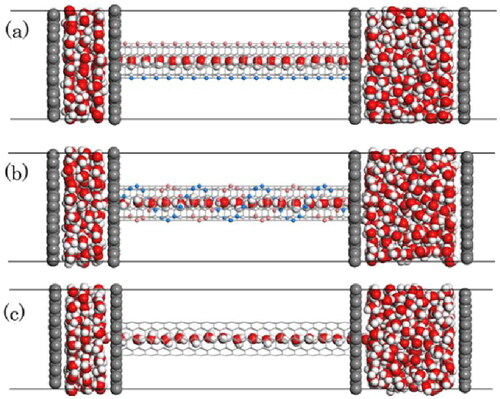
The angles between the water molecule’s dipole moment and the permeation direction were calculated to analyze the temporal evolution of molecular orientation. This analysis focused on a singular water molecule permeating through, and a typical angle plot is presented in . It is shown that molecules exhibit frequent rotations while present in the feed or permeate regions, distinct from their behavior inside the membrane pore. Conversely, in (a), during the duration when the molecules are in the pore, the angle exhibits small change compared to the feed or permeate regions, preserving nearly constant orientation during passage. In the case of (b), characterized by a spiral charge arrangement on the pore-wall, large instantaneous changes due to molecular rotation within the pore are observed. However, the orientation remains nearly constant, indicating a molecular rotation was controlled by electrostatic interactions with atoms on the pore wall. Conversely, in (c), the angle amplitude of water molecules within the pore is more expansive compared to (a) and (b). Nevertheless, the angle oscillates around 30, demonstrating vibrations of approximately ±20. Overall, when the pore diameter is close to the size of one water molecule, the water molecule diffuses in a single-file manner, while engaging in hydrogen bond formations. In addition, when atomic charges are present on the membrane pore walls, the precedence is on hydrogen bond formation with the pore wall, exerting stronger control on permeation compared to molecular orientation.
Figure 4. Time evolution of typical one water molecule’s angle plot that permeated through the membrane when the applied pressure of feed region was 500 atm. (a) The straight model, (b) the double helix model, and (c) the uncharged model.
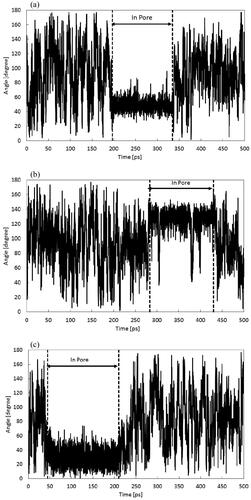
To reveal the effect of molecular orientation behavior on the water flux, shows the time evolution of the number of water molecules in the permeate region. The increment in the count of permeated water molecules demonstrates a linear increase, enabling determination of the water flux from the slope. In , the increments in the number of permeating molecules show consistently in a linear increment, signifying the steady-state permeation took place. Additionally, the slopes were changed depending on the feed region pressures. Conversely, within model (c) where the pore wall is uncharged, the number of molecules appears to exhibit a reverse flow when the feed pressure is below 200 atm, failing to depict a linear increase. This phenomenon arises due to the greater permeation resistance exceeding the applied pressure difference. However, in the models (a) and (b), a certain permeation manifests even below 200 atm, suggesting that the addition of a charge to the pore walls promotes the water permeation. Furthermore, upon comparison between (a) and (b) for pressures at or below 200 atm, the elevation in the number of permeating molecules is more pronounced in (a), indicating lower permeation resistance, which is discussed in details in the next sentence.
Figure 5. Change of number of permeated water molecules for various different applied pressure conditions calculated by NEMD. (a) The straight model, (b) the double helix model, and (c) the uncharged model.
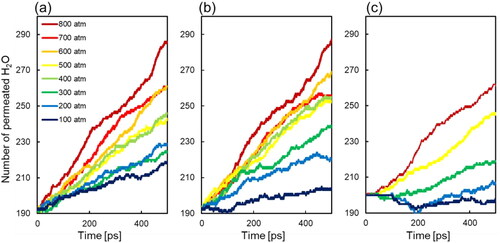
Calculated flux form is summarized in as a function of the applied feed pressure. The straight model indicates the highest permeation flux under 200 atm range, while at higher pressures, the double helix model exhibits the highest permeation flux. Considering the charge density on the pore walls in the linear and double helix models, the latter showcases a larger charge density on the pore-wall. At lower pressures, where the flux is relatively small, hydrogen bond formations readily occur with the pore-walls due to the slower mobility of a water molecule. Consequently, the double helix model has a higher charge density, which makes the permeation resistance increase, resulting in less flow. Conversely, under elevated pressures, the higher mobility of permeating water molecules makes it difficult for them to form hydrogen bonds with the pore-wall. Therefore, the charge density of the pore-wall plays an important role in determining the water flux, leading to a higher water flow in the double helix model. Furthermore, the uncharged model shows the smallest permeation flux. This is due to the hydrophobic effect of pore-wall, reducing the frequency of water molecules entering the pores from the liquid phase.
Figure 6. Dependency of calculated flux on the applied feed pressure for three different models. Keys: ● the double helix model, ▲ the straight model, □ the uncharged model. Surface pore ratio: 0.0837.
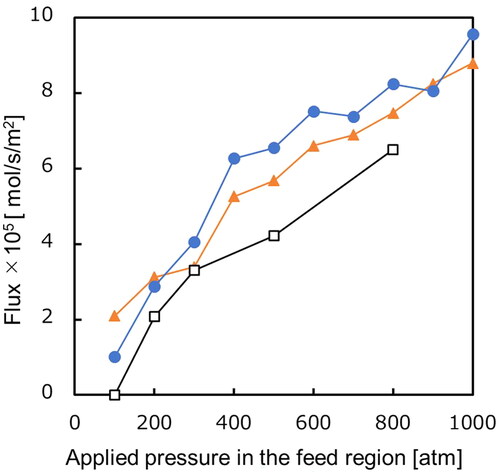
All calculated flux for the models surpassed the reported water molecule permeation rate of 108 mol/s in aquaporins (de Groot and Grubmüller Citation2001), although the model employed in this study applies a pressure greater than osmotic pressure. Hinds et al. (Citation2004) synthesized a membrane in which aligned CNTs were incorporated into a polymer film and reported on its water permeability. They reported that the water permeability of CNT membranes is 4.68 × 10−5 mmol/m2/s/bar. The water permeability coefficient in the double helix model of our study was 4.35 × 10−6 mmol/m2/s/bar. There is a difference of about 10 times when comparing the two. This is probably due to imperfections in the membranes used in the experiment. Since our membrane model has no defects, it is reasonable that our value is smaller than the experimental value. Our NEMD simulation results can be considered valid by comparing them with other NEMD results and experimental results, as mentioned above.
4. Conclusions
NEMD was used to investigate water permeation through charged CNT membranes with different charge arrangements on the pore wall.
The water flux in the charged pore-wall models showed higher water compared to that in the uncharged pore-wall model.
The charged atoms on CNT pore-wall significantly alter the orientation of permeating water molecules in the pore.
A double helix arrangement showed a higher flux at the higher applied pressure than the linear arrangement due to the frequent formation of hydrogen bonds with the pore walls.
A linear arrangement and a double helix arrangement, the latter exhibiting higher flux at the higher applied pressure due to the frequent formation of hydrogen bonds with pore walls.
Optimization of charge arrangements offers the potential to improve water permeation through CNT-like microporous membranes, especially at lower applied pressure conditions. As the flux in the double helix model showed higher flux, the higher the charge density in the pore wall, the higher the flux. It is noted that the atomic charge in the pore wall is similar to the atomic charge of water. These findings provide insights for the development of strategies to improve water permeation in RO membranes.
References
- Araki T, Cruz-Silva R, Tejima S, Takeuchi K, Hayashi T, Inukai S, Noguchi T, Tanioka A, Kawaguchi T, Terrones M, et al. 2015. Molecular dynamics study of carbon nanotubes/polyamide reverse osmosis membranes: polymerization, structure, and hydration. ACS Appl Mater Interfaces. 7:24566–24575. doi: 10.1021/acsami.5b06248.
- Bordin JR, Ilha AV, Côrtes PRB, da Silva Oliveira W, Pinheiro LA, de Moraes EE, Grison TG, Köhler MH. 2023. Molecular modeling of aquaporins and artificial transmembrane channels: a mini-review and perspective for plants. Theor Exp Plant Physiol. 2023:1–16. doi: 10.1007/s40626-023-00284-2.
- Chan W-F, Chen HY, Surapathi A, Taylor MG, Shao X, Marand E, Johnson JK. 2013. Zwitterion functionalized carbon nanotube/polyamide nanocomposite membranes for water desalination. ACS Nano. 7:5308–5319. doi: 10.1021/nn4011494.
- Corry B. 2008. Designing carbon nanotube membranes for efficient water desalination. J Phys Chem B. 112:1427–1434. doi: 10.1021/jp709845u.
- Corry B. 2011. Water and ion transport through functionalised carbon nanotubes: implications for desalination technology. Energy Environ Sci. 4:751–759. doi: 10.1039/c0ee00481b.
- Dauber-Osguthorpe P, Roberts VA, Osguthorpe DJ, Wolff J, Genest M, Hagler AT. 1988. Structure and energetics of ligand binding to proteins: E. coli dihydrofolate reductase-trimethoprim, a drug-receptor system. Proteins. 4:31–47. doi: 10.1002/prot.340040106.
- de Groot BL, Grubmüller H. 2001. Water permeation across biological membranes: mechanism and dynamics of aquaporin-1 and GlpF. Science. 294:2353–2357. doi: 10.1126/science.1062459.
- Dresselhaus MS, Gene D, Phaedon A. 2001. Carbon nanotubes: synthesis, structure, properties, and applications. Berlin: Springer.
- Ewald PP. 1921. Die Berechnung optischer und elektrostatischer Gitterpotentiale. Ann Phys. 369:253–287. doi: 10.1002/andp.19213690304.
- Fan H, Gu J, Meng H, Knebel A, Caro J. 2018. High-flux membranes based on the covalent organic framework COF-LZU1 for selective dye separation by nanofiltration. Angew Chem Int Ed Engl. 57:4083–4087. doi: 10.1002/anie.201712816.
- Higuchi H, Miyagawa M, Takaba H. 2022. Solvent–solute interaction effect on permeation flux through forward osmosis membranes investigated by non-equilibrium molecular dynamics. Membranes. 12:1249. doi: 10.3390/membranes12121249.
- Hinds BJ, Chopra N, Rantell T, Andrews R, Gavalas V, Bachas LG. 2004. Aligned multiwalled carbon nanotube membranes. Science. 303:62–65. doi: 10.1126/science.1092048.
- Hirosawa F, Miyagawa M, Takaba H. 2023. High efficient CO2 separation at high pressure by grain-boundary-controlled CHA zeolite membrane investigated by non-equilibrium molecular dynamics. Membranes. 13:278. doi: 10.3390/membranes13030278.
- Hirosawa F, Watanabe K, Miyagawa M, Takaba H. 2023. Direct evaluation of void effect on gas permeation in mixed matrix membrane by non-equilibrium molecular dynamics. J Membr Sci. 677:121594. doi: 10.1016/j.memsci.2023.121594.
- Hummer G, Rasaiah JC, Noworyta JP. 2001. Water conduction through the hydrophobic channel of a carbon nanotube. Nature. 414:188–190. doi: 10.1038/35102535.
- Itoh Y, Chen S, Hirahara R, Konda T, Aoki T, Ueda T, Shimada I, Cannon JJ, Shao C, Shiomi J, et al. 2022. Ultrafast water permeation through nanochannels with a densely fluorous interior surface. Science. 376:738–743. doi: 10.1126/science.abd0966.
- Kalra A, Garde S, Hummer G. 2003. Osmotic water transport through carbon nanotube membranes. Proc Natl Acad Sci U S A. 100:10175–10180. doi: 10.1073/pnas.1633354100.
- Lin L-C, Choi J, Grossman JC. 2015. Two-dimensional covalent triazine framework as an ultrathin-film nanoporous membrane for desalination. Chem Commun. 51:14921–14924. doi: 10.1039/c5cc05969k.
- Lin L-C, Grossman JC. 2015. Atomistic understandings of reduced graphene oxide as an ultrathin-film nanoporous membrane for separations. Nat Commun. 6:8335. doi: 10.1038/ncomms9335.
- Liu J, Wang N, Yu LJ, Karton A, Li W, Zhang WX, Guo FY, Hou LL, Cheng QF, Jiang L, et al. 2017. Bioinspired graphene membrane with temperature tunable channels for water gating and molecular separation. Nat Commun. 8:2011. doi: 10.1038/s41467-017-02198-5.
- Majumder M, Chopra N, Andrews R, Hinds BJ. 2005. Nanoscale hydrodynamics: enhanced flow in carbon nanotubes. Nature. 438:44–44. doi: 10.1038/438044a.
- Meyyappan M. 2005. Carbon nanotubes: science and applications. Boca Raton, FL: CRC Press.
- Nagumo R, Muraki Y, Iwata S, Mori H, Takaba H, Yamada H. 2016. Molecular dynamics simulation study on CO2 physical absorption mechanisms for ethylene-glycol-based solvents using free energy calculations. Ind Eng Chem Res. 55:8200–8206. doi: 10.1021/acs.iecr.6b01074.
- Nicholls WD, Borg MK, Lockerby DA, Reese JM. 2012. Water transport through carbon nanotubes with defects. Mol Simul. 38:781–785. doi: 10.1080/08927022.2011.654205.
- Rashed AO, Merenda A, Kondo T, Lima M, Razal J, Kong L, Huynh C, Dumée LF. 2021. Carbon nanotube membranes – strategies and challenges towards scalable manufacturing and practical separation applications. Sep Purif Technol. 257:117929. doi: 10.1016/j.seppur.2020.117929.
- Rathinavel S, Priyadharshini K, Panda D. 2021. A review on carbon nanotube: an overview of synthesis, properties, functionalization, characterization, and the application. Mater Sci Eng B. 268:115095. doi: 10.1016/j.mseb.2021.115095.
- Rizzuto C, Pugliese G, Bahattab MA, Aljlil SA, Drioli E, Tocci E. 2018. Multiwalled carbon nanotube membranes for water purification. Sep Purif Technol. 193:378–385. doi: 10.1016/j.seppur.2017.10.025.
- Shaikh AR, Kamio E, Takaba H, Matsuyama H. 2015. Effects of water concentration on the free volume of amino acid ionic liquids investigated by molecular dynamics simulations. J Phys Chem B. 119:263–273. doi: 10.1021/jp5095239.
- Sianipar M, Kim SH, Khoiruddin K, Iskandar F, Wenten IG. 2017. Functionalized carbon nanotube (CNT) membrane: progress and challenges. RSC Adv. 7:51175–51198. doi: 10.1039/C7RA08570B.
- Su P, Wang F, Li Z, Tang CY, Li W. 2020. Graphene oxide membranes: controlling their transport pathways. J Mater Chem A. 8:15319–15340. doi: 10.1039/D0TA02249G.
- Takaba H, Hisabe T, Shimizu T, Alam MK. 2017. Molecular modeling of OH– transport in poly(arylene ether sulfone ketone)s containing quaternized ammonio-substituted fluorenyl groups as anion exchange membranes. J Membr Sci. 522:237–244. doi: 10.1016/j.memsci.2016.09.019.
- Takaba H, Onumata Y, Nakao SI. 2007. Molecular simulation of pressure-driven fluid flow in nanoporous membranes. J Chem Phys. 127:54703–54709. doi: 10.1063/1.2749236.
- Thebo KH, Qian X, Zhang Q, Chen L, Cheng H, Ren W. 2018. Highly stable graphene-oxide-based membranes with superior permeability. Nat Commun. 9:1486. doi: 10.1038/s41467-018-03919-0.
- Thomas M, Corry B. 2016. A computational assessment of the permeability and salt rejection of carbon nanotube membranes and their application to water desalination. Philos Trans A Math Phys Eng Sci. 374:20150020. doi: 10.1098/rsta.2015.0020.
- Wu W, Su J, Jia M, Zhong W, Li Z, Li W. 2019. Ultrastable sandwich graphene oxide hollow fiber membranes with confined interlayer spacing. J Mater Chem A. 7:13007–13011. doi: 10.1039/C9TA03236C.
- Yoshioka T, Kotaka K, Nakagawa K, Shintani T, Wu H-C, Matsuyama H, Fujimura Y, Kawakatsu T. 2018. Molecular dynamics simulation study of polyamide membrane structures and RO/FO water permeation properties. Membranes. 8:127. doi: 10.3390/membranes8040127.
- Yoshioka T, Tsuru T, Asaeda M. 2001. Molecular dynamics studies on gas permeation properties through microporous silica membranes. Sep Purif Technol. 25:441–449. doi: 10.1016/S1383-5866(01)00073-9.
- Zhang C, Wu BH, Ma MQ, Wang Z, Xu ZK. 2019. Ultrathin metal/covalent-organic framework membranes towards ultimate separation. Chem Soc Rev. 48:3811–3841. doi: 10.1039/C9CS00322C.
- Zhang M, Guan K, Ji Y, Liu G, Jin W, Xu N. 2019. Controllable ion transport by surface-charged graphene oxide membrane. Nat Commun. 10:1253. doi: 10.1038/s41467-019-09286-8.
- Zhang W, Zhang L, Zhao H, Li B, Ma H. 2018. A two-dimensional cationic covalent organic framework membrane for selective molecular sieving. J Mater Chem A. 6:13331–13339. doi: 10.1039/C8TA04178D.
- Zhou W, Wei M, Zhang X, Xu F, Wang Y. 2019. Fast desalination by multilayered covalent organic framework (COF) nanosheets. ACS Appl Mater Interfaces. 11:16847–16854. doi: 10.1021/acsami.9b01883.