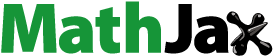
ABSTRACT
Soil moisture variation plays a key role in maintaining the engineering properties of soils. One of the main factors that impact the soil moisture content is the temperature, which has a strong impact to change the initial or design moisture and results in significant soil volume changes. This study aims to investigate the effects of temperature on the performance of enzymatically stabilised soils. The enzyme used in this study is Eko-soil and the soil used is clayey silt of low plasticity (ML). The mechanical behaviour was first assessed through the unconfined compression strength and weight measurements based on samples exposed to a series of operational temperatures. X-ray μCT tomography images were then used to assess the microstructure. Porosity, size and geometrical shape of the pores were analysed at elevated temperatures. Results revealed that the enzyme is effective in maintaining the initial pore structure under the tested temperature range.
1. Introduction
Soils are composites of different components consisting of minerals, microorganisms and water. Their formation involves a series of complex physiochemical processes with the presence of various chemicals, pressure, temperature and humidity content with respect to the time of the development. Unlike other civil engineering construction materials such as cementitious products and steel, soil is susceptible to volume variation due to the applied loadings or environmental conditions. Volume changes are more common in expansive soils and occur mainly due to the changes in moisture content. Changes in atmospheric conditions lead the expansive soils to experience swelling and shrinkage resulting in uneven settlement, cracking, rutting and other forms of defects which subsequently will damage the infrastructure placed both under and above ground. A study carried out by the National Science Foundation claimed that the damages due to the soil volume variation in pavement structures are greater than natural disasters like earthquakes and floods (Murmu, Dhole, and Patel Citation2020; Punthutaecha et al. Citation2006; Zhang et al. Citation2022). In the United Kingdom, the annual cost of infrastructure damages related to soil failure is approximately £400 million (Dang et al. Citation2015) while in the U.S.A., the same is estimated to be approximately $US15 billion (Jones and Jefferson Citation2012). Significant scale damages are also reported in China due to soil failure (Li, Cameron, and Ren Citation2014). Around 50,000 houses are cracked each year in Australia as a result of physical changes in the foundations caused by unwanted soil movement (Considine Citation1984). Therefore, there is a need to mitigate volume changes in problematic/expansive soils subjected to weather changes and take necessary engineering precautions to minimise the damages to infrastructures built upon them.
Stabilisation is a common method to control the expansiveness of soil by altering the soil composition or by preserving the clayey particles from moisture and temperature variations. Traditional methods of soil stabilisation such as mixing soil with a higher ratio of coarse aggregates and compaction have been used for many decades to improve the engineering properties of expansive soils (Afrin Citation2017; Amšiejus et al. Citation2014; Mishra, Sachdeva, and Manocha Citation2019; Tisdall and Oades Citation1979; Xie et al. Citation2020). While engineering properties of soil can be improved by mixing with aggerates, maintaining the design engineering properties (such as degree of compaction/moisture content) throughout the year is challenging due to climate variations (Saberi, Annan, and Konrad Citation2018). Alternatively, various chemical products are being used to stabilise soils thereby mitigating the swelling and shrinkage. Researchers have invested significant efforts into evaluating soil stabilisation under various environmental conditions including frosty environments. (Almajed, Khodadadi Tirkolaei, and Kavazanjian Citation2018) used cohesionless soil material (i.e. Ottawa Sand) and enzymatically stabilised the soil by using the enzyme product content 3 g/L of water, calcium chloride concentration of 0.67 M and Ezyme-Induced Carbonate Precipitation (EICP) treatment solution composed of 1 M urea. Samples were prepared by using the solely percolation method to develop the cylindrical sample 10 cm in height and 5 cm in diameter consisting of acrylic thin-walled product and placing the polypropylene-liner within the cylinders to facilitate any extraction and testing of the treated soil. The authors used multiple treatments of EICP and washed with deionised water, thus; poured from the top of the tube and drained by gravity by placing a syringe needle in the base of the tube (the samples were cured 7 days after each treatment to achieve the precipitated carbonated calcium, hence the stabilisation). The UCS test was then conducted and the authors found that the UCS value of 88.8 kPa was achieved when the relative density of sand reached 92%. (Wang et al. Citation2021) evaluated the unconfined compressive strength (UCS) of soil stabilised with cement and other admixtures at approximately frozen environmental conditions. The authors used 6% cement (i.e. dry mass of soil) with different dosages of other admixtures and found that the UCS values were decreased as the dosages of admixtures were increased. In addition, the UCS values were increasing at elevated temperatures (i.e. from −12.0 to 1.5 Celsius (Co)). (Aryal and Kolay Citation2020) used cement in different quantities in combination with polypropylene fibre to stabilise soil and tested under the mean of freeze and thaw testing (i.e. from −12 Co to 23 Co). The authors found that at 10% cement, soil specimens were incapable to resist the freeze and thawing due to the high cement content. However, it was also found that stabilisation was most achievable by adding 5% of the polypropylene fibres and 5% cement to the soil. Cement-based stabilisation with other chemical or texture products can have enhanced effectiveness in soil stabilisation mechanism; however, it should be noted that soil stabilisation using cement with other texture products can incur heavy construction costs. (George, Ponniah, and Little Citation1992) evaluated the effectiveness of the soil stabilisation by using the lime for different soils (i.e. Clayey Silt and Silty Clay) at different elevated temperatures and wet/dry conditions using the UCS test. The authors used different lime contents for each of the soil materials and determined the UCS values after 7 days and 21 days of curing at 20, 35 and 50 Celsius. Their results indicated that in both curing periods, the UCS values were increased with the increase in temperature. (Nasrizar, Muttharam, and Illamparuthi Citation2010) also used lime to stabilise black cotton soil at 3, 5, 7 and 9 Percentages of the bulk mass at elevated temperatures by the mean of the UCS test. The authors found, after 7 days and 28 days of curing period at 5 , 30 and 50 Celsius, UCS values increased with the increase of lime content after the 28-day curing period. (Salimi and Ghorbani Citation2020) used geopolymer products of Granulated Blast Furnace Slag (GBFS) and Basic Oxygen Furnace Slag (BOFS) with different contents of activators such as calcium oxide (CaO) and medium reactive magnesia (MgO). The authors evaluated the effectiveness of geopolymer for soil stabilisation at 20 Co and 45 Co after 7, 28 and 90 days of curing period, and found that the UCS values increased with the increasing activator content at 45 Co curing temperature and 90 days of the curing period. (Bin-Shafique et al. Citation2010) used fly ash for soil stabilisation under freeze and thawing environmental conditions. The authors used various fly ash contents with soft clay and expansive clay at 12-h freeze and thawing cycle (i.e. 12-h at frost conditions and 12-h at ambient conditions). They found that the UCS values increased with the increase of fly ash content after 12 days for the expansive soil. Therefore, it is evident that traditionally used chemical products can be used for soil stabilisation at different temperatures. However, one of the drawbacks of these chemicals is the significant impact they impose on the environment. Nevertheless, there is limited research conducted in evaluating the effects of soil stabilisation applications at different environmental conditions and determining its impacts, typically at various operational temperatures. Hence, there is a need for further progression in investigating the soil performance when experiencing high temperatures and ensuring that the soil stabilisation mechanism is maintained when exposed to ambient environmental conditions.
Recently, researchers have identified alternative soil stabilisers that have the potential to limit the use of chemical stabilisers. For example, enzyme-based products that have the ability to bio-chemically attain more densified and stabilised soil structure have gained the attention of researchers. The mechanism of soil stabilisation using the enzyme-based products involves the electronegativity charge neutralisation of the clay minerals; thus, negatively charged fine-grained materials encapsulate with the addition of the enzyme materials and decrease the water affinity, therefore leading the soil molecules to more stabilised, hence more densified soil material (Rauch, Katz, and Liljestrand Citation1993). Various researchers have utilised enzymes for soil stabilisation in laboratory experiments as well as in field applications. Results of these studies have demonstrated significant benefits in performance under stabilised conditions (Li, Li, and Dan Citation2011; Marasteanu et al. Citation2005; Milburn and Parsons Citation2004; Thomas, Tripathi, and Yadu Citation2018; Zamri et al. Citation1999). (Renjith et al. Citation2020) used Eko-soil enzyme-based product to evaluate the effectiveness of different content of Eko-soil enzyme on the fine-grained field soil and showed that enzymatic stabilisation can be effective in improving confined soil strength. The findings revealed that the optimum enzyme content in a diluted mass ratio (DMR) for the typical soil conditions was 1:500 when adding an application mass ratio (AMR) of 1%. Furthermore, the authors evaluated the effect of temperature on enzymatic stabilisation by heating the raw soil for 48 h to different elevated temperatures at 20, 40 and 60 Celsius before adding the enzyme. Soil stabilisation was then assessed by the mean of the UCS tst where the authors found that the most stabilised soil material is obtained at the pre-enzyme cured temperature of 40 Co. (Sidiq et al. Citation2023) evaluated the effectiveness Eko-soil product with the addition of the cement product at the laboratory scale by using the identical contents as have been used in the field in stabilising approximately 34, 238 m2 land area for the large industrial infrastructure to be built upon it. The authors found that the addition of the Eko-soil product (i.e. without cement product) improved the densification (i.e. MDD) of soil by 4.2% and reduction in moisture content (i.e. OMC) by approximately 10% in comparison to the raw soil. In addition, the authors observed a significant improvement in mechanical properties such as the CBR value (representing the confined soil conditions in the field) was increased by a factor of 4 in comparison to the raw soil (i.e. without the addition of cement product). The utilisation of the enzyme product for enzymatically stabilised soil has been used for areas up to 65,000 m2 (O’Donnell et al. Citation2022). However, research exploring the effects of temperature and other environmental conditions on post-enzymatic stabilisation is limited, and there is a need to further evaluate the mechanical behaviour considering advanced microstructural analyses to better understand the mechanism of stabilization.
This study investigates the effects of elevated temperature on the mechanical response of enzymatic stabilised soils using unconfined compressive strength and state-of-the-art microscopic tests for a soil located in Melbourne, Australia. The study uses enzyme-based product, Eko-soil to evaluate its effectiveness of maintaining stabilisation at different elevated temperatures at consecutive curing periods, which can be expected in the field conditions due to the seasonal periods. The mechanical behaviour was first assessed through UCS and moisture content variation tests. The efficiency of the soil stabilisation at different elevated temperatures was then assessed and compared to the raw soil through the porosity, pores size and micro-architectural variation obtained from the reconstructed three-dimensional images.
2. Research significance
Soil stabilisation is commonly used at laboratory scale by means of mechanical/hydraulic testing without evaluating its effectiveness under temperature variations as typically applicable in field contexts. As a result, there is limited literature on the performance of stabilised soil and its mechanism changes at temperatures other than tested conditions in labs/fields. This study aims to investigate the soil stabilisation mechanism under various operational temperatures through two stages of testing; 1) Evaluation of mechanical efficacy for enzymatic stabilised soil at different elevated temperatures and consecutive curing periods and 2) Demonstration of micro-architectural aspects of soil matrix at elevated temperatures by the mean of the most advanced contactless and non-destructive test through X-ray micro-tomography. Findings from this study will assist in understanding how enzymatic stabilisation behaves at elevated/reduced temperatures from ambient temperature conditions, thus enabling optimisation of its use for mitigating serviceability issues from moisture-sensitive expansive soils.
3. Methodology
3.1. Material
The materials used in the experiments include soil obtained from a field located in Melbourne- Australia and commercially available Eko enzyme-based products. The soil used in this study was obtained from the field by land excavation at 1 m depth and twelve points throughout the entire field. The soil at the site generally consisted of 50% gravel and 50% clay. According to Unified Soil Classification Systems (USCS), the dominant soil type can be classified as Clayey Silt of Low Plasticity (ML). The soil characteristics in accordance with the AS1289.3.1.1–2009 (Citation1289.3.1.1, A. 2009), AS1289.3.2.1–2009 (Citation2009, A.), AS1289.3.3.1–2009 (Citation1289.3.3.1, A. 2009), AS1289.3.4.1–2016 (Citation1289.3.4.1, A. 2008) including the Particle Distribution Size in accordance with AS 1289.3.6.1–2009 (Citation1289.3.6.1, A. 2009) are shown in and . The Enzyme, Eko-soil, was used for the soil stabilisation process and was obtained from the Centre for Pavement Excellence Asia Pacific Ltd. The enzyme, which is brown in colour with a sweet ferment odour, is produced from the water and purified protein obtained from the plants’ sources and chemically; is a non-toxic, non-hazardous, and environmentally friendly product (Soil Citation2015). According to the manufacturer (Soil Citation2015), the enzyme contains 20% water, 20% non-ionic surfactant and 60% of ferment base ingredients. shows the physical and chemical characteristics of the enzyme used as an additive in this study.
Table 1. Typical characteristics of the selected soil.
Table 2. Physical and chemical characteristics of the enzyme.
3.2. Sample preparation and curing
Soil was heated to 110 Co for 24 h to remove any moisture and was sieved through 19 mm according to AS 1289.5.1.1–2017 (Standard, A. Citation2017). The samples were then prepared in accordance with the Australian Standards for compaction and the UCS testing while Eko-Soil was added at a diluted mass ratio and application mass ratio of 1:312 and 1%, respectively. The quantity of the enzyme content (i.e. in liquid state) was subtracted from the optimum moisture content to eliminate the increase in the liquid content within the soil. For the compaction and dry density evaluation, samples were prepared in accordance with AS 1289.5.1.1–2017 (Stabdard, A. Citation2017) by using the standard compaction method. Thus, mixing the dried soil was progressed with an increment of 2% water content from dry to wet of the OMC and pre-cured for three days at ambient conditions (additional attention was taken to maintain the consistency of the moisture content). Then, the samples were prepared and cured a further four days at ambient conditions.
3.2.1. Mechanical tests
For the UCS tests, the samples were prepared in accordance with AS 5101.4_2008 (Standard, A. Citation2008), then they were cured (i.e. after four days of post-curing) at controlled relative humidity at 65% and elevated temperatures from 0, 15, 30, 45 and 60 Celsius at 24 h intervals. It should be noted that the samples tested at 60 Celsius had experienced a temperature of 15, 30 and 45 Celsius (i.e. four day-night cycles). The UCS tests were conducted in accordance with AS 5101.4_2008 (Standard, A. Citation2008). The soil specimen was placed in the lower bearing plate of the MTS 1000kN machine while the upper bearing plate was in contact with the top surface of the sample. Caution was taken during this process to minimise any damage to the specimen. Testing was proceeded by applying 60 kN/min till the sample reached the failure condition ().
3.2.2. Microstructural tests
For the microstructural tests, cylindrical soil samples with a size ratio of 1:0.32 to the UCS samples were prepared in accordance with the AS 5101.4_2008 (Standard, A. Citation2008) for investigating the micro-structural aspect of the pores by using the X-ray micro CT tomography. Identical to the UCS samples, the soil samples tested for the X-ray tomography were also placed at different elevated temperatures starting from 0 Co and after the completion of the test, the same samples were placed and cured at controlled relative humidity (i.e. 65%) and experienced the prospective elevated temperature of 15, 30, 45 and 60 Celsius for the subsequential 24 hours’ time. Microstructural tests were conducted by Bruker 1275 SkyScan to obtain the micro-architectural pores within the soil samples. The instrument was set to 100 μA tube and 100 kV tube and by rotating the soil sample at a speed of 0.2 degrees per second around its axis, a total of 1535 of 2D images were obtained to reconstruct 3D profiles and obtain internal porosity data ().
4. Results and discussion
In the following sections, results from the mechanical and non-destructive tests are presented and discussed. Results from the compaction, bulk/dry weight ratio (i.e. moisture variation indicator) and UCS are presented first followed by a demonstration of the micro-structural analyses in relation to the overall porosity, single pore volume and the geometrical aspects such as the sphericity, Structure Model Index and orientation of the pores within the soil matrix obtained from the X-ray micro-CT tomography.
4.1. Compaction characteristics
Compaction characteristics at the ambient environmental conditions for the soil mixture with and without the enzyme products are shown in . It can be seen that the compaction measure for the raw soil mixture (i.e. soil without enzyme product) is 11.4% (OMC) and 2010 kg/m3 (MDD). Effectively, the addition of the enzyme to the soil impacts the compaction behaviour of the soil, i.e. the moisture content is shifted towards the left direction to 9.25% which is a reduction of approximately 18.8% in comparison to the raw soil, while the dry density was increased by 4.2% compared to the raw soil mixture. The reduction in OMC is due to the confinement mechanism of the soil molecules (it can be noticed that the soil is classified as clayey silt, therefore, this soil has a low portion of the clay mixed with silt) from the enzyme leading to balance the electronegativity change of the soil molecules (i.e. low portion of clay mixed with silt), hence reduction on the water absorbency mechanism. According to (Rauch, Katz, and Liljestrand Citation1993), enzyme-soil stabilisation applications involve encapsulating the clay mineral molecules with enzyme-based products. By using the identical enzyme product for soil stabilisation application, similar observations were also made by (Renjith et al. Citation2017) and (Pooni et al. Citation2019a, Citation2021).
4.2. Moisture variation
Results of bulk weight/dry weight ratio for the soil mixtures with and without enzyme product are presented in this section as an indicator of moisture variation at different elevated temperatures. These measures were obtained by monitoring bulk weights during incrementing temperature of 15 Co over 24-h intervals (i.e. from 15 to 60 Celsius) and compared to the initial maximum dry weight of the soil sample at the room temperature 22.5 C0 (i.e. raw and enzymatic soil mixtures); hence, the bulk weight/dry weight implies sample moisture variation at a given exposed temperature. After 4 days of sample curing at ambient environmental conditions, samples to be frozen were further cured for 24 h at 0 Co while the remaining samples were placed at a controlled relative humidity of 65% and 15 Co. After each test, the temperature was further increased by 15 Co, thus the samples tested at 60 Co have been cured for 4 days after the initial curing of 4 days (i.e. at atmospheric conditions).
shows the bulk weight/dry weight ratio variation of the two soil mixtures at different elevated temperatures at 24-h intervals. The rate of reduction of moisture at different elevated temperatures varies for the enzymatic soil compared to the raw soil, as shown in , i.e. the larger amount of water was lost from control samples compared to enzymatic soils (i.e. higher gradient for raw soil compared to enzymatic soils). This reveals that the enzymatic soils are capable of retaining moisture thereby the initial porosity compared to non-enzymatic soils.
Figure 4. Bulk/Dry weight ratio for the soil mixtures with and without the enzyme product at elevated temperatures.
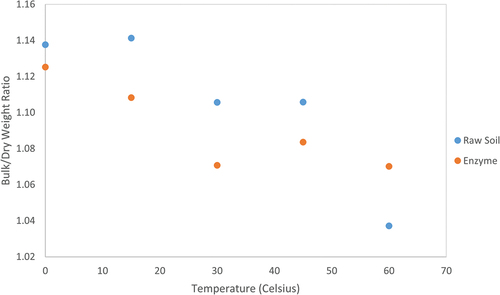
It can be seen that, at 0 Co, the bulk/dry weight ratio for raw soil is higher by approximately 1.09% than the soil containing the enzyme product. This increase is due to the higher optimum moisture content in raw soils compared to enzymatic samples. After 24 h and an increase of 15 Co, this ratio for the enzymatic soil was dropped to 1.11 (i.e. reduction of 2.88%) while the raw soil ratio remains largely unchanged. The subsequent increase in temperature has resulted in a decreasing trend of bulk weight for both soil mixtures at 30 Co at a higher reduction rate for control samples than enzymatic soils. This clearly indicates that the enzyme is responsible for controlling the moisture content. It acts as a barrier for the clay particles and is responding to control the moisture absorbency within the soil matrix whereas at the same temperature and humidity conditions, the moisture absorbency was higher in raw soil samples which effectively leads to decrease the moisture content rapidly. However, as the temperature reached 45 Co, the moisture content for the enzymatic soil increased by a further 1.27% compared to the raw, indicating the enzyme is responsive to retaining the moisture in the sample from the surrounding environmental impact. After 4 days of post-curing (i.e. at the temperature of 60 Co), the ratio for raw soil has a significant drop, which is a reduction of 6.63% less from the previous temperature (i.e. 45 Co), and this can be due to the large moisture lost within the raw soil matrix. However, for the enzymatic soil, the ratio was decreased by1.27% less from the previous temperature (i.e. 45 Co), due to the responsive maintenance of the moisture content from the encircled humidity content. Notably, the moisture loss for the raw soil is rapid at high temperatures compared to the enzymatic soil (i.e. range from 30 to 60 Celsius).
4.3. Unconfined Compression Strength (UCS)
illustrates the UCS results for the soil mixtures with and without the enzyme at different elevated temperatures. At frozen conditions, the UCS values for both soil mixtures are drastically higher in comparison to the other environmental conditions of elevated temperatures. At frozen conditions, The UCS values for the raw soil mixture and enzymatic soil are 4254 kPa and 3069 kPa, respectively. Relatively, the UCS value for the raw mixture is higher by 38.6% in comparison to the enzymatic soil under frozen conditions. High UCS values under frost conditions can be due to the higher interlocking of the ice component (i.e. available water content within the soil matrix) that can be added to the initial UCS values of the soil. Similar observations were obtained by Louis and James (Wolfe and Thieme Citation1964) who found that for the clay material, the UCS values were parabolically increased as the temperature was drastically reduced from 0 to −200 Celsius and the maximum UCS values were obtained as ~ 45 MPa and 65 MPa for clay and silt material at −130 Co and −125 Co, respectively in comparison to the artificial ice having the UCS values of 19.3 MPa at −200 Co. Moreover, after curing the soil samples for 24 h at 15 Co (i.e. after 4 days of post-curing), the UCS values were drastically decreased. Thus, for the raw soil and enzymatic soil, UCS was dropped to 309.2 kPa and 275.9 kPa, respectively. An explanation of such a drop in the UCS values can be due to a subsequential mechanism; 1) the loss of the ice content (which acted as a barrier to resist loading), and 2) a change in the microstructural properties of the soil matrix (i.e. the change in volume of the water state from the solid to liquid). Enzymatic soil has proven to be more effective in encapsulating the clayey particles and control of the moisture content while reducing the porosity. However, such reduction in porosity is not reflected in UCS which was 10.7% lower in enzymatic soils compared to the control condition. Similar lower UCS response was observed by (Renjith et al. Citation2020) who subsequently showed that the reduction in porosity is more pronounced under confined conditions (such as CBR) for enzymatic soil which showed high CBR compared to control soils. Effectively, for the enzymatic soil, the effect of the curing period and incremental in temperature have impacted the performance of the mechanical properties at 30 Co while a decrease in the UCS value (21.9%) was obtained in comparison to the raw soil at 45 Co. However, at 60 Co, the UCS values for both soil mixtures are almost identical (the difference is only 4.9%) indicating that the enzymatic soil was able to achieve higher UCS at increased temperatures. The increase of the UCS values at much higher temperatures can be due to subsequential mechanism, i.e. 1) the microstructural re-arrangement of the clayey particles and the affinity of the moisture content; as the soil (i.e. both the raw and enzymatic soils) experience higher temperatures, the clayey particles initiate the development of the larger pores. On the other hand, by maintaining the relative humidity of 65%, these pores are enclosed resulting in increased adhesions between the clayey particles; 2) the incremental curing time which potentially leads to further progression of the microstructural re-arrangement of the minerals. Similar results were obtained by (Wang, Zentar, and Abriak Citation2017) who noted an increase in the soil strengths at various curing times while changing the temperatures 40 and 60 Celsius when using lime and cement at 3% and 6% as the additives for stabilising the marine soils for road constructions. Ultimately, the UCS value difference for the enzymatic and raw soil is decreasing as the temperature increases, i.e. UCS of both raw soil and enzymatic soil increases with increasing temperature while the rate of increase in UCS is higher for enzymatic soils compared to raw soils. This can be due to the rate of the substrate reaction of the enzyme product that increases on the surface of the clayey particles at higher temperatures (Rao, Scelza, and Gianfreda Citation2014; Tabatabai Citation1994), leading to a greater encapsulation mechanism on the clayey particles, hence controlling the microstructure between the clayey particles. Similar results were noted by (Stone et al. Citation2012). The microstructural analyses of the soil matrix are further detailed in later sections of this study.
4.4. X-ray analysis
In this section, 2D images from X-ray micro-CT tomography were reconstructed into 3D images, and the effectiveness of the enzyme products was evaluated by using the size and micro-architectural of the pores within the soil matrix.
4.4.1. Visual analysis
Qualitative analysis was used to evaluate the effectiveness of the enzyme product in the soil matrix through the 3D images from the X-ray micro-CT tomography. shows the 3D images of the raw and enzymatic soil mixtures at different elevated temperatures; the red-coloured objects represent the pores, and the green represents the soil. The pores’ structure and volume seem to be slightly lower for the raw soil with more of the pores shaping in rode-like structures at 0 Co in comparison to the enzymatic soil. An explanation can be due to the formation of the ice fragments with the sharp ends (i.e. from the water content) that can potentially impact the pores’ shape within the soil matrix, whilst for the enzymatic soil, the pores’ shape remained unchanged, indicating the ice fragments and its sharpness had a minimum effect due to the addition of the enzyme product and the encapsulation mechanism of the soil particles, hence resisting any internal changes. After curing the soil samples at 15 Co for 24 h (i.e. post-curing for 4 days), the internal pores for both soil mixtures were increased as a result of the increased temperatures. It should be noted that for the enzymatic soil, most of the pores seem to be interconnected rather than developing new-formed pores due to temperature change, in comparison to the raw soil which indicated the formation of new pores. In addition, for the enzymatic soil, the new formation of the pores remained at a minimum when the temperature was increased to 30 Co during the following 24 h. For the raw soil, drastic changes occurred when the temperature rises to 45 Co during a further 24 h as the pores’ formation started to significantly increase in comparison to the previous temperatures whilst for the enzymatic soil, such formation of pores was very low. This indicates the enzyme is holding its original pore structure at higher temperatures and restrains the soil from undergoing adverse changes at high temperatures. In fact, as the temperature was further increased to higher degrees, the quantity of the pores within the enzymatic soil was reduced, indicating its efficiency in maintaining the soil interconnection and densifications in comparison to the raw soil where the quantity of the pores was significantly increased due to the lower capability in maintaining the soil structure (i.e. loss of moisture content and absorbency of the moisture due to the relative humidity 65%). The temperature increase has led to loose higher moisture in the raw soil (thus larger porosity resulted), whilst for the enzymatic soil, the enzyme restraints the soil matrix from the pores’ formation, hence a more densified material was obtained.
4.4.2. Porosity
Using 3D images reconstructed from X-ray micro-CT tomography, a concise segmentation between the solid and void within the soil matrix was obtained. The porosity within the specified object is defined as the void fraction within the sample, hence leading to the densification characteristics of the material (Li et al. Citation2021; Sidiq et al. Citation2019). The porosity variation for the raw and enzymatic soil mixtures due to the elevated temperatures is shown in . It can be seen that the porosity for the raw and enzymatic soil is 0.82% and 1.74%, respectively, at 0 Co, which indicates the addition of the enzyme is less effective in minimising the porosity within the soil matrix in comparison to the raw soil at low temperature. Similar observations were obtained earlier (i.e. qualitative analysis) where the geometrical aspects of the pores vary for the raw and enzymatic soil mixtures. When the soil matrix was exposed to 15 Co for 24 h, the porosity for raw and enzymatic soil was increased to 0.95% and 2.7%, respectively, which can be due to the release of the moisture content within the soil matrix. However, as the temperature was increased to 30 Co, there is a change in the trend for both soil mixtures, i.e. the enzymatic soil showed a reduction in the porosity to 2.51% while the raw soil gained an increase in porosity to 1.51%. This is due to the rate of the moisture content loss within the raw soil matrix is relatively high at higher temperatures, leading to the rapid formation of the pores within the soil matrix in contrast to enzymatic soils which can hold the pores at elevated temperatures. Further, the curing time of 3 days (i.e. after 4 days post-curing) at different elevated temperatures assists the enzyme to minimise the formation of the newly pores as a 3-day curing time is ideal for optimum enzymatic stabilisation. Similar results were found by (Pooni et al. Citation2019b; Renjith et al. Citation2020). The increase in temperature beyond 45 Co has resulted in a decrease in the porosity for both raw and enzymatic soils. This can be due to the micro-stability of the soil in the presence of relative humidity and the water absorbency of the clayey particles. As the soil experiences high temperatures, the soil becomes more porous due to the loss of moisture content; on the other hand, the presence of relative humidity prevents the formation of such pores, leading to less porous materials. These results were also observed in the mechanical section (section 4.3) as the strength was increasing with the increment of the temperature, or the strength could potentially be reduced without the presence of the humidity. In addition, for the enzymatic soil, the porosity is lower for high temperatures in comparison to the raw soil, which is due to the rate of the substrate reaction of the enzyme being greater at higher temperatures (Stone et al. Citation2012; Tabatabai Citation1994). One could argue that for the enzymatic soil, the mechanical performance was reduced at higher temperatures when compared to raw soil, while at identical temperatures, the porosity for the enzymatic is reduced when compared to the raw soil (results in a denser material, therefore could have been resulted in higher strength). It can be attributed that at higher temperatures, the substrate reaction of the enzyme is relatively higher, which leads to further achievement in encapsulating the clayey particles, therefore the water content (i.e. from the relative humidity of 65%) that is available within the soil matrix is less absorbed by the clayey particles, leading to lower the mechanical performance under unconfined conditions. Hence, at higher temperatures, the higher substrate reaction of the enzyme is preventing the clayey particles from losing water content within the clayey particles and limits the formation of the pores at high temperature. Similar results are also demonstrated as the temperature reaches 60 Co.
4.4.3. Volume variation of pores
The volumetric size of the pores was obtained from the reconstructed 3D images from X-ray micro-CT tomography by applying the marching cube algorithm. Since each voxel in the marching cube algorithm has eight vertexes, there are 256 possibilities of every voxel interconnecting to the next one (Lorensen and Cline Citation1987; Sidiq et al. Citation2021). shows the volumetric size of the pores and their occurrences for the raw and enzymatic soil mixtures. For the raw soil, the quantity of the pores with identical volumetric sizes increases at elevated temperatures. For instance, pores with a volumetric size of approximately 0.001 mm3 (i.e. peak value) are increased by a factor of 1.43, 1.87 and 1.88 for 15, 30 and 45 Celsius respectively in comparison to the soil at low temperature (i.e. 0 Co) with a slight drop of the peak at 60 Co. Concurrently, at different temperatures, the peaks are positively skewed, indicating larger pores are formed as the soil experienced higher temperatures. On the other hand, the addition of the enzyme product has drastically changed the volumetric size of the pores when compared to the raw soil, although both mixtures experienced identical curing time and environmental conditions. Considering the same pore volume size of 0.001 mm3, the quantity of the pores at 15 Co and 30 Co is increased by a factor of 1.58 and 1.47, respectively for the enzymatic soil, in comparison to the values obtained at 0 Co which is very similar to the raw soil at the same temperatures. It can be noted that the enzymatic stabilisation is more productive in limiting the formation of the newly pores from the elevated temperatures, while for the same conditions, the pore sizes in the raw soil were consecutively increased. Moreover, for the enzymatic soil, the formation of the pores is decreasing as the sample experienced a temperature beyond 30 Co whilst the skewness of the curve is slightly lower in comparison to the raw soil at the higher temperatures (>30 Co). It can be noted that the addition of the enzyme product has the capability to minimise the changes occurred within the soil matrix when the soil is subjected to different temperature conditions. This is due to the encapsulation mechanism that adhered the soil particles thus minimising the soil particles to undergo changes in the moisture content.
4.4.4. Sphericity variation
The confined air or water within the soil matrix is formed in various shapes and varies from the perfect special, partially spherical, or non-spherical as a result of the balanced pressures from the different mediums (Or and Tuller Citation2002). Sphericity is calculated as the ratio of the surface area in volume equivalent and the surface area of a particle and its measure ranges from zero to one (i.e. non-spherical to a perfect sphere shape) (Wadell Citation1935). In this study, the sphericity scale is used to investigate the shape of the pores based on the sphericity scale that occurs during the elevated temperatures and 24-h incremented curing time. shows the sphericity shape of each of the pores in relation to the volume for the raw and enzymatic soil mixtures 0 Co. The range of the sphericity scale for both soil mixtures is almost the same, whilst; the sphericity scale is shifted towards the left direction of the x-axis for the raw soil (i.e. greater data density towards zero), indicating more of the pores with larger volume having the shape of complete non-spherical. In regard to the enzymatic soil, the sphericity scale of pores is close to the values of one demonstrating pores are more in spherical shape. Due to the extensive number of pores within the soil matrix and the performance of the analysis for individual pores, statistical analyses were conducted to evaluate the effectiveness of the pores’ shape (i.e. sphericity scale) at elevated temperatures.
Figure 9. Sphericity variation for different soil mixtures at different zero Celsius (a) raw soil and (b) enzymatic soil.
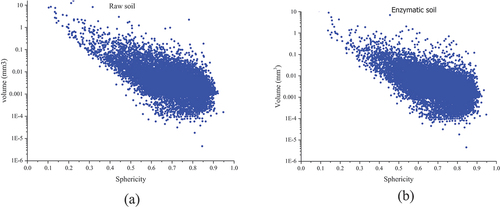
The Probability Density Function (PDF) technique was used to evaluate the statistical analysis of the pores within the soil matrix (EquationEquation 1(1)
(1) ) for determining the density of the probability rather than the actual probability. shows the probability density function for raw and enzymatic soil and the changes in the sphericity occurrences at different elevated temperatures. The average sphericity scale for the raw soil at 0 Co and 15 Co is 0.735 ± 0.113 while the curve is negatively skewed, demonstrating majority of the pores are towards the non-spherical shape. When the temperature increases to 30 , 45 and 60 Celsius, the mean value decreases to 0.727, 0.722 and 0.717, respectively, with lower PDF values. This indicates the shape of the pores is becoming more non-spherical with the increase in temperatures. While for the enzymatic soil, the sphericity scale of the pores at frozen status is 0.735 ± 0.110 which is identical to the raw soil. This can be due to the impact of a large quantity of the pores (i.e. obtaining data set for each pore within the soil samples) within the two soil mixtures on sphericity values. Similar results were obtained in the earlier section as the porosity of the enzymatic soil was higher at a very low temperature. The condition remains similar at 15 Co as for the raw soil; however, the sphericity scale slightly decreases to 0.729, 0.726 and 0.725 at a temperature of 30 , 45 and 60 Celsius with higher PDF values in comparison to the raw soil. In addition, the skewness (i.e. towards the left lower sphericity values) of the data for enzymatic soils is more compacted when compared to the raw soil. Such a result demonstrates the addition of the enzyme product limits the change of the geometry of the pores in the soil matrix, hence indicating the pore volume/shape remained unchanged from the shrinkage and development of the cracks during the elevated temperatures as the result of the encapsulation mechanism of the enzyme within the soil matrix. Concurrently, at higher temperatures, the enzyme product is responsive in re-attracting the clayey particles and reforming a more densified soil.
Figure 10. Probability density function curves for different soil mixtures at elevated temperatures (a) raw soil and (b) enzymatic soil.
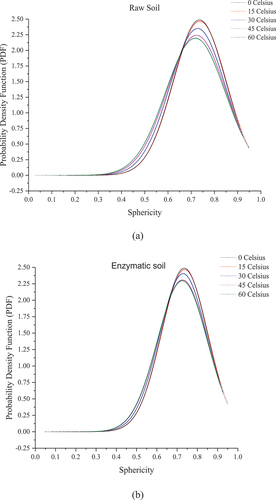
since
and stands for sphericity standard deviation, mean and data variable for the sphericity scale (Okeniyi et al. Citation2014). The sphericity scale is a unitless measure.
4.4.5. Structure model index
Further analyses on the geometrical aspects of the voids within the soil matrix were conducted to evaluate the shape of each of the pores by the mean of the Structural Model Index (SMI). This type of analysis was first investigated by Hilderbrand and Ruegsegger in 1997 (Hildebrand and Rüegsegger Citation1997) which was then widely used in biomedical image investigations to evaluate the micro-architectural structure of bone tissues. Such innovative technique is adopted to the soil to characterise the shape of the crack as ‘plate-like, ‘rod-like, or ‘sphere’ according to SMI of 0, 3, and 4, respectively, which is calculated based on the surface area and the volume of the cracks; the surface area is obtained by applying the triangulation methods from (Lorensen and Cline Citation1987) and the volume is obtained from the polyhedron inside the voids (EquationEquation 2(2)
(2) ).
Since
S’, V and S respectively represent the first derivative of the total surface area (µm2), total volume (µm3) and total surface area (µm2), respectively.
The Distribution of the Structural Model Index of the pores for the raw and enzymatic soil mixtures is shown in . It can be seen the distribution of the shapes for the raw soil at 0 Co are commonly within the rod-like shape; thus, the SMI value is approximately 2.97 (i.e. averaged peak value). An explanation for such pores’ shape can be due to the formation of the ice lattice within the voids that implement the changes in the shape of the pores. These results were also visualised in the earlier section (visual analysis, section 4.4.1). Effectively, the frequency of pores was increased by a factor of 1.38 as the temperature has risen to 15 Co (i.e. at SMI value of 2.97). These changes are due to the interconnection of the initial pores (i.e. at frozen statues) and the formation of the newly pores due to the rise of the temperature resulting in the loss in moisture content. Furthermore, as the temperature increases to 30 Co and 45 Co, the frequency of the pores drastically increases, thus; resulting in SMI of 2.37 and 4.66, respectively. It should be noted that during the consecutive curing period (i.e. After 4 days post-curing), the soil samples experienced consistent relative humidity of 65% during the increase in temperature. As the raw soil was incapable of maintaining the moisture content consistency, the quantity of the pores in rod-like shape were significantly increased as the result of the loss of moisture content. In addition, at 45 Co, the shape of the pores was further shifted towards the left direction of the x-axis, indicating the pores were becoming plate-like shapes (i.e. the pores have the 3D space) (). Moreover, at 60 Co, the quantity of the pores is similar as the sample experienced 45 Co while the SMI value was further shifted towards the left direction of the x-axis. This reveals that the pores within the soil matrix are forming the 3D space, indicating the formed pores are becoming closer to each other and leading to form a large plate-like shape. However, the pore structure was changed when enzyme product was added to the soil (i.e. 0 Co); thus, the SMI peak is slightly shifted towards the right direction of the x-axis and SMI value of approximately 3.2 was obtained. Such behaviour of SMI indicates pores are more in spherical shape, demonstrating the effectiveness of enzymes in controlling the shape of the pores when exposed to very low temperatures by limiting the ice lattice to change the shape of the pores. The quantity of the pores was increased by a factor of 1.54 as the temperature was increased to 15 Co in comparison to the frozen status. It can be noted that the quantity of the pores is increased by 11.5% in comparison to the raw soil whilst the averaged SMI value is different for the raw soil and enzymatic soil (i.e. SMI values of raw soil and enzymatic soil are 2.97 3.2 respectively). Effectively, the quantity of the pores for the same SMI value of 3.2 is increased by a factor of 2.13 in comparison to the frozen status as the soil experienced a temperature of 30 Co (i.e. decrease of 10.1% in comparison to the raw soil). The changes in the shapes of the pores were further progressed as the temperature was increased to 45 Co and the quantity of the pores was increased by 1.61 in comparison to the frozen status (i.e. an increase of 8.2%). It can be noted that there are significant changes in the pores shape formation at 45 Co for the raw soil compared to enzymatic soil. i.e. for the raw soil, the quantity of pores was increased by a factor of 4.66 compared to the increase in enzymatic soil (1.61) which is more towards the spherical shape. Such spherical shapes can be considered as more stabilised shapes when compared to rod and plate-like shapes as the result of the circumferential pressure resulting in equilibrium forces between the different Mediums (Hover Citation1993; Or and Tuller Citation2002). Moreover, at 60 Co, the frequency for the pores for the same SMI value (i.e. 3.2) is decreased by 27.7% and 47.5% in comparison to the frozen status and 15 Co, respectively. In addition, the SMI value is further shifted towards the right direction of the x-axis indicating the pores are more in the spherical ranges. These results demonstrate the addition of the enzyme potentially limits the porosity changes, hence limiting the changes in the pores’ shape occurrences.
4.4.6. Void orientation
The internal orientation of the single void in the soil mixtures at evaluated temperatures is assessed in this section. Based on the X-ray images followed by reconstructing the three-dimensional images, Mean Tensor Length (MTL) technique was used and the orientation of the single cracks in the matrix was obtained. The detailed process of evaluating the MTL can be found in (Dawson et al. Citation2012). shows the distribution of the voids and their structural shape such as plate-like, rod-like and spherical shapes at elevated temperatures. It can be seen that the quantity of the pores for the raw soil and enzymatic soil is distributed more or less equally for the two-soil mixture at frozen status with a lesser quantity (i.e 52.8%) of the pores within the raw soil in comparison to the enzymatic soil. In addition, for the raw soil, the quantity of voids are close to the SMI values lower than 3.0 in comparison to the enzymatic soil. The quantity of the pores slightly increases as the temperature rises to 15 Co and 30 Co while the orientation is approximately maintained consistently. However, changes to the quantities of the pores occur when the temperature was increased to 45 Co and 60 Co; thus, the raw soil becomes more porous while the enzymic soil shows less porousness compared to low-temperature status. This is due to the ability of controlling the initial pore structure in the enzymatic soil matrix as explained previously. However, the orientation of the pores has yet remained unchanged for the two-soil mixture. This is due to the consistency in the equilibrium pressures within the soil matrix leading to minimise the formed pores changing the direction at different angles. One of the advantages of such analysis is that it can evaluate the pore distribution within the soil in correlation with the strength performance. Typically, having a uniform distribution of voids within the sample implies high compaction efficiency which will eventually lead to superior performance. Enzymatic soils reveal uniform void distribution compared to raw soil, enabling to minimise the pre-mature soil failures due to concentrated voids. As soil experiences applied loadings, internal shear stresses would be developed within shear planes. If the orientations of the voids are concentrated towards any angles, then the soil is more likely to result in pre-mature failures prior to design loads. Concurrently, by obtaining the spherical shapes in the soil matrix, it can lead to a lower voids ratio in the soil matrix (Rodriguez Citation2013). Evenly orientated distributed voids in enzymatic soils under high temperatures would limit the weak shear stresses with the uniform distribution of applied forces throughout the soil matrix.
5. Conclusion
Performance of the soil in combination with the enzyme product at elevated temperatures and consecutive curing periods was investigated in this study through the means of mechanical and non-destructive testing techniques. UCS and weight tests were conducted to assess the mechanical performance. X-ray micro-CT tomography and three-dimensional images were used to assess the effectiveness of stabilisation within the soil matrix at elevated temperatures in relation to the porosity and geometrical and orientation of the pores within the soil matrix.
Based on the results, the following conclusions can be drawn;
The addition of the enzyme product into the soil in the ambient environment has resulted in decreasing moisture content by up to 18.8% and increasing MDD by approximately 4.2% in comparison to the raw soil due to the encapsulation process of the enzyme product with the soil particles.
Results from the UCS showed that the soil with and without the enzyme potentially maintained high strength at extremely low temperatures; however, the unconfined strength significantly dropped at the initial stages of the temperature rising. Relatively, the strength for raw soil and enzymatic soil was increased as the temperature was increased to 60 Co due to a subsequent increase in suction at reduced moisture content.
Based on the qualitative analysis obtained from the X-ray micro-CT tomography 3D images, the porosity within the raw soil was increased as the temperature rises while for the enzymatic soil, the porosity within the soil matrix is slightly higher and was maintained approximately consistent till 45 Co prior to decreasing further as the temperatures reached 60 Co.
Based on the quantitative analysis, the porosity for the enzymatic soil was higher at lower temperatures in comparison to the raw soil; however, once the soil samples were exposed to 30 to 60 Celsius, the porosity parabolically decreased compared to the raw soil where the porosity was relatively higher.
Based on the volumetric variation of the pores, the frequency of newly formed pores in the raw soil was significantly increased with the temperature while for the enzymatic soil, the frequency of the newly formed pores was limited irrespective of the increase in temperature.
Results from the sphericity analysis indicated that the addition of the enzyme product into the soil maintained a consistent pore shape at different elevated temperatures in comparison to the raw soil where the sphericity is slightly shifted towards the non-spherical ranges due to the newly formed pores at elevated temperature.
Based on the SMI results, the pores within the raw soil are more in the rod to plate-like shape and the frequency of occurrences of pores increased as the temperature increased, whilst for the enzymatic soil, the averaged pore shapes are rod to spherical shape, and it adheres to the approximately consistent frequency of occurrences at various temperatures.
The orientation of the pores for both soil mixtures remained unchanged during the elevated temperatures indicating that the shear stresses under unconfined conditions remained almost consistent when subjected to applied loadings. For the enzymatic soil, pores remained almost consistent during the elevated temperatures showing more of the rod and spherical shapes compared to the raw soil which showed plate-like shape formed at various orientations.
Results from the experimental program revealed that mixing enzyme products into the soil can increase maximum dry density with reduced optimum moisture content. Also, the addition of the enzyme product into the soil limited the formation of the newly formed pores with the soil matrix and it facilitates holding the initial porosity approximately consistent at various temperatures. Furthermore, the addition of the enzyme in the soil potentially lowers the quantity of pores within the soil matrix as the temperatures reached elevated temperatures (such as 60 Co) due to the adhesion mechanism from enzyme chemical reactions at high temperatures (Razavi, Blagodatskaya, and Kuzyakov Citation2016; Stone et al. Citation2012).
Acknowledgments
This research was conducted by the Australian Research Council Industrial Transformation Research Hub for nanoscience-based construction material manufacturing (IH150100006) and Transformation of Reclaimed Waste Resources to Engineered Materials and Solutions for a Circular Economy (IH200100010). The X-ray facility and Microscopy & Microanalysis facility provided by RMIT University is acknowledged.
Disclosure statement
No potential conflict of interest was reported by the author(s).
Additional information
Funding
Notes on contributors
Amir Sidiq
Dr Amir Sidiq is a Research Fellow at RMIT University, Australia. He obtained his first degree in 2012 followed by his Master’s degree in 2016 and Ph.D. degree in 2021 at RMIT University. He has 2 years of industrial experience in geotechnical engineering in Australia and has conducted multidisciplinary research works. His research areas are construction materials investigation, structural design, asset management and structural digital twin development.
Dilan J. Robert
Dilan Robert is a Chartered Civil Engineer and an Associate Professor in Engineering at RMIT University. He obtained his first degree (2005) from University of Moratuwa, Sri Lanka, and the PhD degree (2011) from University of Cambridge, UK. His research areas include road pavements, soil stabilisation, soil–structure interaction analysis, offshore geotechnics, unsaturated soils and waste recycling in construction. He is a Fellow of Engineers Australia and has over 20 years experience in geotechnical and underground infrastructure projects.
Brian O’Donnell
Mr. O’Donnell is the Chief Executive Officer of Center for Pavement Excellence Asia Pacific (CPEAP), established to provide standards and guidance on innovative stabilisation techniques for road pavements. His career spans more than 50 years in local government, culminating as a City Engineer/City Planner at municipalities in Melbourne Australia, and his interest in design of pavements commenced in 1970 when he had to manage highly reactive clays for constructing roads. He has been recently awarded a Shobhit University Honorary Doctorate for Research.
Susanga Costa
Dr Susanga Costa is a senior lecturer in geotechnical engineering at Deakin University, Australia. He completed his PhD in geomechanics from Monash University Australia. He has expertise in expansive soil behaviour, fracture in clay soils and soil stabilization. Dr Costa’s research interests include use the reclaimed and recycled waste for soil stabilization, mitigation of desiccation cracks, application of microbial calcite precipitation to fine grained soils and impact of climate change on shallow ground.
Sujeeva Setunge
Sujeeva Setunge is the Associate Deputy Vice Chancellor Research and Innovation for Science, Engineering and Health at RMIT University in Melbourne, Australia. She is the director of the ARC research hub on transformation of reclaimed resources to engineered materials and solutions for a circular economy (TREMS). She leads other large multidisciplinary research projects in the areas of climate resilience, digital twins of infrastructure, smart cities and zero emissions. Professor Setunge is a Fellow of Engineers Australia and a chartered professional engineer.
Usha Iyer-Raniga
Professor Usha Iyer-Raniga is at the School of Property and Construction Management, RMIT University. Usha was co-lead of the UN’s One Planet Network’s Sustainable Buildings and Construction Programme (SBC), where the work directly impacted SDG 11 on Sustainable Cities and Communities and SDG 12 on Responsible Consumption and Production. She is on the editorial board for refereed journals and has led Special Issues. Usha serves on the Boards of various not for profit organisations and has also served as panel judge for state, national and international awards.
References
- 1289.3.1.1, A., Methods of Testing Soils for Engineering Purposes, Method 3.1.1: Soil Classification Tests - Determination of the Liquid Limit of a Soil - Four Point Casagrande Method. 2009.
- 1289.3.3.1, A., Methods of Testing Soils for Engineering Purposes Soil Classification Tests - Calculation of the Plasticity Index of a Soil. 2009.
- 1289.3.4.1, A., Methods of Testing Soils for Engineering Purposes Soil Classification Tests - Determination of the Linear Shrinkage of a Soil - Standard Method. 2008.
- 1289.3.6.1, A., Methods of Testing Soils for Engineering Purposes - Soil Classification Tests - Determination of the Particle Size Distribution of a Soil - Standard Method of Analysis by Sieving. 2009.
- 2009, A., Methods of Testing Soils for Engineering Purposes Method 3.2.1: Soil Classification Tests-Determination of the Plastic Limit of a Soil Standard Method
- Afrin, H. 2017. “A review on different types soil stabilization techniques.” International Journal of Transportation Engineering Technology 3 (2): 19–24. https://doi.org/10.11648/j.ijtet.20170302.12.
- Almajed, A., H. Khodadadi Tirkolaei, and E. Kavazanjian Jr. 2018. “Baseline investigation on enzyme-induced calcium carbonate precipitation.” Journal of Geotechnical and Geoenvironmental Engineering 144 (11): 04018081.
- Amšiejus, J., N. Dirgėlienė, A. Norkus, and Š. Skuodis. 2014. “Comparison of Sandy Soil Shear Strength Parameters Obtained by Various Construction Direct Shear Apparatuses.” Archives of Civil and Mechanical Engineering 14 (2): 327–334. https://doi.org/10.1016/j.acme.2013.11.004.
- Aryal, S., and P. Kolay. 2020. “Long-Term Durability of Ordinary Portland Cement and Polypropylene Fibre Stabilized Kaolin Soil Using Wetting–Drying and Freezing–Thawing Test.” International Journal of Geosynthetics & Ground Engineering 6 (1): 1–15. https://doi.org/10.1007/s40891-020-0191-9.
- Bin-Shafique, S., K. Rahman, M. Yaykiran, and I. Azfar. 2010. “The Long-Term Performance of Two Fly Ash Stabilized Fine-Grained Soil Subbases.” Resources, Conservation & Recycling 54 (10): 666–672. https://doi.org/10.1016/j.resconrec.2009.11.007.
- Considine, M. 1984. “Soils Shrink, Trees Drink and Houses Crack.” Journal ECOS Magazine 41:13–15.
- Dang, L. 2015. “Influence of Bagasse Ash and Hydrated Lime on Strength and Mechanical Behaviour of Stabilised Expansive Soil.” Journal GEOQuébec. https://opus.lib.uts.edu.au/handle/10453/37558.
- Dawson, A. R., P. K. Dehdezi, M. R. Hall, J. Wang, and R. Isola. 2012. “Enhancing Thermal Properties of Asphalt Materials for Heat Storage and Transfer Applications.” Road Materials and Pavement Design 13 (4): 784–803. https://doi.org/10.1080/14680629.2012.735791.
- George, S., D. Ponniah, and J. Little. 1992. “Effect of temperature on lime-soil stabilization.” Construction and Building Materials 6 (4): 247–252. https://doi.org/10.1016/0950-0618(92)90050-9.
- Hildebrand, T., and P. Rüegsegger. 1997. “Quantification of Bone Microarchitecture with the Structure Model Index.” Computer Methods in Biomechanics and Biomedical Engineering 1 (1): 15–23. https://doi.org/10.1080/01495739708936692.
- Hover, K. 1993. “Why is There Air in Concrete?” Journal Concrete Construction 38 (1): 11–15.
- Jones, L., and I. Jefferson. 2012. “ChapterC5 expansive soils.” ICE manual of geotechnical engineering 1:413–441.
- Li, J., D. A. Cameron, and G. Ren. 2014. “Case Study and Back Analysis of a Residential Building Damaged by Expansive Soils.” Journal Computers Geotechnics 56:89–99. https://doi.org/10.1016/j.compgeo.2013.11.005.
- Li, Y. J., L. Li, and H. C. Dan. 2011. “Study on Application of TerraZyme in Road Base Course of Road.” Applied Mechanics and Materials 97–98:1098–1108. https://doi.org/10.4028/www.scientific.net/AMM.97-98.1098.
- Li, L., X.-A. Li, Li Wang, Bo Hong, J. Shi, and J. Sun. 2021. “Correction To: The Effects of Soil Shrinkage During Centrifuge Tests on SWCC and Soil Microstructure Measurements.” Bulletin of Engineering Geology and the Environment 80 (1): 731–733. https://doi.org/10.1007/s10064-020-02024-1.
- Lorensen, W. E., and H. E. Cline. 1987. “Marching Cubes: A High Resolution 3D Surface Construction Algorithm.” In ACM Siggraph Computer Graphics, 163–169. ACM SIGGRAPH Computer Graphics. https://doi.org/10.1145/37401.37422.
- Lorensen, W. E., and H.E.J.A.s.c.g. Cline. 1987. “Marching Cubes: A High Resolution 3D Surface Construction Algorithm.” ACM SIGGRAPH Computer Graphics 21 (4): 163–169. https://doi.org/10.1145/37402.37422.
- Marasteanu, M. O. 2005. “Preliminary Laboratory Investigation of Enzyme Solutions as a Soil Stabilizer.”
- Milburn, J. P., and R. L. Parsons. 2004. Performance of Soil Stabilization Agents. Kansas. Dept. of Transportation.
- Mishra, S., S. Sachdeva, and R. Manocha. 2019. “Subgrade soil stabilization using stone dust and coarse aggregate: A cost effective approach.” Journal International Journal of Geosynthetics Ground Engineering 5 (3): 1–11. https://doi.org/10.1007/s40891-019-0171-0.
- Murmu, A. L., N. Dhole, and A. Patel. 2020. “Stabilisation of Black Cotton Soil for Subgrade Application Using Fly Ash Geopolymer.” Journal Road Materials Pavement Design 21 (3): 867–885. https://doi.org/10.1080/14680629.2018.1530131.
- Nasrizar, A., M. Muttharam, and K. Illamparuthi. “Role of Lime Content on Soil-Lime Reaction Under Thermal Curing.” In Proceedings of Indian Geotechnical Conference–2010, GEOtrendz, IIT Bombay, Mumbai, India, December 16. 2010.
- O’Donnell, B., A. S. Amir Sidiq, D. Robert, S. Setunge. 2022. Guidelines for Enzymatic Soil Stabilization. International conference on Variability of the Sun and sun-like stars: from asteroseismology to space weatherInternational conference on Variability of the Sun and sun-like stars: from asteroseismology to space weather, Monash University, Melbourne, Australia, p. 373–398.
- Okeniyi, J. O., I. J. AMBROSE, S. O. OKPALA, O. M. OMONIYI, I. O. OLADELE, C. A. LOTO, and P. A. IDOWU POPOOLA. 2014. “Probability Density Fittings of Corrosion Test-Data: Implications on C6H15NO3 Effectiveness on Concrete Steel-Rebar Corrosion.” Sadhana 39 (3): 731–764. https://doi.org/10.1007/s12046-014-0226-9.
- Or, D., and M. Tuller. 2002. “Cavitation during desaturation of porous media under tension.” Journal Water Resources Research 38 (5): 19-1-19–14. https://doi.org/10.1029/2001WR000282.
- Pooni, J., F. Giustozzi, D. Robert, S. Setunge, and B. O’Donnell. 2019a. “Durability of Enzyme Stabilized Expansive Soil in Road Pavements Subjected to Moisture Degradation.” Journal Transportation Geotechnics 21:100255. https://doi.org/10.1016/j.trgeo.2019.100255.
- Pooni, J., F. Giustozzi, D. Robert, S. Setunge, and B. O’Donnell. 2019b. “Durability of enzyme stabilized expansive soil in road pavements subjected to moisture degradation.” Transportation Geotechnics 21:100255. https://doi.org/10.1016/j.trgeo.2019.100255.
- Pooni, J., D. Robert, C. Gunasekara, F. Giustozzi, and S. Setunge. 2021. “Mechanism of Enzyme Stabilization for Expansive Soils Using Mechanical and Microstructural Investigation.” Journal International Journal of Geomechanics 21 (10): 04021191. https://doi.org/10.1061/(ASCE)GM.1943-5622.0002164.
- Punthutaecha, K., A. J. Puppala, S. K. Vanapalli, and H. Inyang. 2006. “Volume Change Behaviors of Expansive Soils Stabilized with Recycled Ashes and Fibers.” Journal of Materials in Civil Engineering 18 (2): 295–306. https://doi.org/10.1061/(ASCE)0899-1561(2006)18:2(295).
- Rao, M., R. Scelza, and L. Gianfreda. 2014. “Soil enzymes.” Journal Enzymes in Agricultural Sciences 1–18. http://uploads.worldlibrary.net/uploads/pdf/20150423221243enzymes_june14.pdf.
- Rauch, A. F., L. E. Katz, and H. M. J. W. Liljestrand. 1993. “An Analysis of the Mechanisms and Efficacy of Three Liquid Chemical Soil Stabilizers.” 1.
- Razavi, B. S., E. Blagodatskaya, and Y. Kuzyakov. 2016. “Temperature Selects for Static Soil Enzyme Systems to Maintain High Catalytic Efficiency.” Soil Biology & Biochemistry 97:15–22. https://doi.org/10.1016/j.soilbio.2016.02.018.
- Renjith, R.2017 Enzyme Based Soil Stabilization for Unpaved Road Construction. In MATEC Web of Conferences, Seoul, South Korea. EDP Sciences. https://doi.org/10.1051/matecconf/201713801002.
- Renjith, R., D. J. Robert, C. Gunasekara, S. Setunge, and B. O’Donnell. 2020. “Optimization of Enzyme-Based Soil Stabilization.” Journal of Materials in Civil Engineering 32 (5): 04020091. https://doi.org/10.1061/(ASCE)MT.1943-5533.0003124.
- Rodriguez, J. 2013. “Importance of the Particle Shape on Mechanical Properties of Soil Materials.” Luleå tekniska universitet.
- Saberi, M., C.-D. Annan, and J.-M. Konrad. 2018. “On the Mechanics and Modeling of Interfaces Between Granular Soils and Structural Materials.” Archives of Civil and Mechanical Engineering 18 (4): 1562–1579. https://doi.org/10.1016/j.acme.2018.06.003.
- Salimi, M., and A. Ghorbani. 2020. “Mechanical and Compressibility Characteristics of a Soft Clay Stabilized by Slag-Based Mixtures and Geopolymers.” Journal Applied Clay Science 184:105390. https://doi.org/10.1016/j.clay.2019.105390.
- Sidiq, A., R. J. Gravina, S. Setunge, and F. Giustozzi. 2019. “Microstructural Analysis of Healing Efficiency in Highly Durable Concrete.” Construction and Building Materials 215:969–983. https://doi.org/10.1016/j.conbuildmat.2019.04.233.
- Sidiq, A., D. Robert, R. Gravina, and F. Giustozzi. 2021. “Coupled FEM-Microstructural X-Ray Examination of a Controlled Internal Damage Approach for Concrete Samples.” Archives of Civil and Mechanical Engineering 21 (2): 1–20. https://doi.org/10.1007/s43452-021-00219-1.
- Sidiq, A., D. J. Robert, B. O’Donnell, S. Setunge, A. Swarup, and F. Tostvrsnik. 2023. “Investigation of Enzyme–Based Soil Stabilization in Field Application.” Journal of Materials in Civil Engineering 35 (5): 04023086. https://doi.org/10.1061/(ASCE)MT.1943-5533.0004742.
- Soil, E., Sko Soil Manual. 2015. Melbourne_Australia.
- Stabdard, A., AS 1289.5.1.1_Method of Testing Soils for Engineering Purposes_method 5.1.1: Soil Compacting and Density Tests-Determination of the Dry Density/Moisture Content Relation of a Soil Using Standard Compactive Effort. 2017.
- Standard, A. 2008. “As 5101.4_Methods for Preperation and Testing of Stablized Materials_method 4: Unconfined Compression Strength of Compacted Materials.”
- Standard, A., Method of Testing Soils for Engineering Purposes_method 5.1.1: Soil Compacting and Density Tests-Determination of the Dry Density/Moisture Content Relation of a Soil Using Standard Compactive Effort. 2017.
- Stone, M. M., M. S. Weiss, C. L. Goodale, M. B. Adams, I. J. Fernandez, D. P. German, S. D. Allison, et al. 2012. “Temperature Sensitivity of Soil Enzyme Kinetics Under N -Fertilization in Two Temperate Forests.” Journal Global Change Biology 18 (3): 1173–1184. https://doi.org/10.1111/j.1365-2486.2011.02545.x.
- Tabatabai, M. 1994. “Soil enzymes.” Journal Methods of Soil Analysis: Part 2 Microbiological Biochemical Properties 5:775–833. https://doi.org/10.2136/sssabookser5.2.c37.
- Thomas, A., R. Tripathi, and L. Yadu. 2018. “A Laboratory Investigation of Soil Stabilization Using Enzyme and Alkali-Activated Ground Granulated Blast-Furnace Slag.” Arabian Journal for Science and Engineering 43 (10): 5193–5202. https://doi.org/10.1007/s13369-017-3033-x.
- Tisdall, J., and J. Oades. 1979. “Stabilization of Soil Aggregates by the Root Systems of Ryegrass.” Journal Soil Research 17 (3): 429–441. https://doi.org/10.1071/SR9790429.
- Wadell, H. 1935. “Volume, Shape, and Roundness of Quartz Particles.” Journal the Journal of Geology 43 (3): 250–280. https://doi.org/10.1086/624298.
- Wang, J., A. C. Fayish, B. Hoffheins, and M. L. McCahan. 2021. “The Evaluation of Chemical Admixtures on the Performance of Cement Stabilized Materials in Cold Weather.” Journal Transportation Geotechnics 30:100618. https://doi.org/10.1016/j.trgeo.2021.100618.
- Wang, D., R. Zentar, and N. E. Abriak. 2017. “Temperature-Accelerated Strength Development in Stabilized Marine Soils as Road Construction Materials.” Journal of Materials in Civil Engineering 29 (5): 04016281. https://doi.org/10.1061/(ASCE)MT.1943-5533.0001778.
- Wolfe, L. H., and J. O. Thieme. 1964. “Physical and Thermal Properties of Frozen Soil and Ice.” Journal Society of Petroleum Engineers Journal 4 (1): 67–72. https://doi.org/10.2118/675-PA.
- Xie, Y., S. Costa, L. Zhou, and H. Kandra. 2020. “Mitigation of Desiccation Cracks in Clay Using Fibre and Enzyme.” Bulletin of Engineering Geology and the Environment 79 (8): 4429–4440. https://doi.org/10.1007/s10064-020-01836-5.
- Zamri, A. 1999. National Seminar on Mechanization in Oil Palm Plantation. Towards Improving Productivity Through Mechanization, Bangi, Selangor, Malaysia. PORIM.
- Zhang, J., X. Wang, L. Shi, and Y. Yin. 2022. “Enzyme-Induced Carbonate Precipitation (EICP) Combined with Lignin to Solidify Silt in the Yellow River Flood Area.” Construction and Building Materials 339:127792. https://doi.org/10.1016/j.conbuildmat.2022.127792.