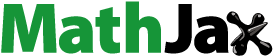
ABSTRACT
Climate change and its impacts on agriculture are relatively well documented at the global and regional levels, while adaptation measures to sustain food production are mostly location specific. Such measures integrated into a production system as a farming practice will become robust based on its performance at smallholder farms. Therefore, a long-term (2011-2018) study was undertaken to evaluate these practices in a crop-livestock integrated farming system (IFS) approach as a climate-smart agricultural (CSA) strategy for enhancing agricultural production on a humid tropical island. The results showed a significant increase in on-farm productivity from 2.8 t ha−1 in 2011–35.6 t ha−1 in 2018 and net returns from USD 359 to more than 3500 ha−1 due to the adoption of CSA practices. Most importantly the study found increase in the sustainability index (0.89) by the adoption of CSA practices such as crop and enterprise diversification and land manipulation. The climate smartness of these practices was witnessed in reduced greenhouse gas (GHG) emissions and expanded carbon sink that resulted in a mitigation benefit of 5.40 Mg CO2eq ha−1yr−1. CSA practices such as crop diversification through raised beds, enterprise diversification, crop rotation, green manuring, in-situ rainwater harvesting, and contingency cropping significantly contributed to enhancing the production of crop-livestock IFS. Crop residue mulching, agro-forestry and organic waste recycling contributed for enlarging the C sink and minimizing net GHG emissions. Based on these findings, we demonstrated that a crop-livestock IFS was the best climate-smart strategy to enhance the productivity and food security of smallholder farmers in a sustainable way against the consequences of climate change and to increase the mitigating potential through carbon stocking.
Research highlights
Crop-livestock IFS is climate-smart agriculture strategy for humid tropical region
It included enterprise diversification, land manipulation and rainwater harvesting
Climate smart IFS increased on-farm productivity from 2.8–35.6 t ha−1
Positive interlinks of components increased sustainability and nutritional security
Greenhouse gas emissions reduced with mitigation benefit of 5.40 Mg CO2eq ha−1yr−1
1. Introduction
One of the biggest challenges of the twenty-first century is to feed 9 billion people by 2050 in a way that is not detrimental to our planet under a changing climate and in the context of growing competition for land and other natural resources (The Wageningen Statement, Citation2011). In achieving global food security and poverty alleviation, smallholder farms play a significant role, as they are the main source of food, nutrition and livelihood security for 33% of humanity. Conversely, these smallholders and their dependents are undernourished because they are mostly net buyers of food, and their income level is insufficient to access balanced food that they do not produce themselves (IFAD, Citation2013). These small farms deserve greater attention, as they are the engines of growth and form a key element and driver of economic transformation within the broader context of urbanization and development of the non-farm sector.
Reflecting the importance of smallholder farms, there was a heightened focus on them at various levels during the first two decades of the twenty-first address food security and poverty alleviation. In a broader sense, all these activities brought to light the importance of the direct linkages of on-farm agricultural production in smallholder farms with household dietary patterns and the nutrition of individual members (Carletto et al., Citation2015; Swarnam et al., Citation2014). However, most of these small farms in developing countries are rain-fed and highly dependent on climatic conditions, which consequently impact agricultural production (Joshi & Tyagi, Citation2019). Furthermore, the global climate change projections for the tropical region showed an increase in the incidence of natural hazards, such as the variability of rainfall, temperature, tidal surges, floods, droughts and soil salinity (IPCC, Citation2014). This will increase the risks for agricultural production, while their coping abilities at the present level of technologies are reduced with the increase in the frequency and severity of climatic variations (Supplementary Figure 1). This compelling situation has brought together the global- and regional-level focus on addressing food security and resource degradation under emerging climate scenarios at the smallholder level.
Although studies have proven the negative impact of climate change on agricultural production, 19–29% of global greenhouse gas (GHG) emissions are produced by farming (Richards et al., Citation2015; Tubiello, Citation2013). The major portion of this emission comes directly from agricultural production activities and indirectly from land use changes driven by agriculture. With increasing demand for food and other agricultural products, agricultural GHG emissions are likely to increase, largely due to continuing expansion in livestock production, fertilizer use and land cover change (Campbell et al., Citation2014). It is therefore imperative to develop technologies for smallholder farms to reduce GHG emissions, which will not only mitigate climate change but also increase agricultural production by reducing external input use through improved nutrient use efficiency and residue recycling. In this context, agricultural diversification strategies along with the integration of different farm enterprises having synergistic effects (Kurgat et al., Citation2018) play a crucial role in ensuring food security under a changing climate. These practices have the potential to address the basic elements of climate-smart agriculture (CSA) by contributing to sustainable productivity, food security, adaptation to climate change and reducing C emissions (The World Bank, Citation2019). At the same time, a suitable combination of practices and/or enterprises from available options should be made for specific farming situations. Towards this, an integrated farming system (IFS) is an appropriate strategy aimed at efficient, sustainable resource management by judiciously combining different components for increased productivity in such a way that one complements the other in smallholder farms (Gajender et al., Citation2021). The principles of IFS provide enough scope for the inclusion of CSA practices to enable agricultural production systems to be more resilient or less dependent and, thus, less vulnerable to unpredictable hazards (Darnhofer et al., Citation2010). Furthermore, sustainable intensification through IFS has the potential to reduce the demand for land and water, therefore reducing the pressure on natural resources. This is more pertinent to tropical islands where agriculture is more dependent on the performance of monsoons and limited land resources at the same time it has to function within fragile island ecosystems (Velmurugan, Citation2018). Under such situations, the inclusion and evaluation of CSA practices within the farming system approach on a long-term basis will go a long way to address the challenge of achieving food security now and into the future, adapting to climate change and reducing the size of agriculture’s global carbon footprint.
In view of this, the present study was undertaken to evaluate the effect of diversification and integration of farm enterprises by following suitable CSA practices in the tropical coastal lowlands of Andaman Islands, India. Based on the critical review of earlier works and the existing farming systems in island ecosystems, we hypothesize that the integration of location-specific CSA practices into smallholder farms based on the IFS approach will make their production systems climate resilient, contribute to food security by enhancing diversity and help in the reduction of GHG emissions. This pilot study provides a long-term account of the transformation of subsistence rice monocropping to diversified crop-livestock IFS that are climate-smart and offer greater scope for up scaling to similar situations prevailing in most of the tropical islands and coastal region around the globe.
2. Materials and methods
2.1. Study area
The Andaman Group of Islands is located 1200 km south-east of the mainland India in the Bay of Bengal. Similar to most of the tropical islands, Andaman Islands have tropical to humid tropical climate with distinct dry and wet season. The islands receive copious amounts of annual rainfall averaging 2900–3100 mm with the mean maximum and mean minimum temperature of 32°C and 22°C, respectively. The relative humidity varies from 68 to 86%. Further, during the monsoon season the islands also experience deep depressions and tropical cyclones causing severe damage to island agriculture and inundating large tracts of coastal lowlands. The rainfall covers the potential evapo-transpiration (ET) demands during most part of the year, except for seasonal water deficit of 30–40 cm from December to April. The major land forms are longitudinal hills or parallel ridges, valley areas between the parallel ridges and coastal plains. The arable crops such as rice, pulses, and vegetables are grown in valley and coastal plains while plantations of coconut and arecanut are found in slopes of the longitudinal hills. Typical tropical rain forests are found in the higher slopes and hilltops, while mangroves are densely scattered along the coastal region.
2.2. Site description and experimental plan
To address the objectives of the study, a long-term crop-livestock integrated farming system experiment was initiated in 2011 in an area of 0.75 ha. This represents small and marginal holdings in the tropical areas. Crop and enterprise diversification was carried out over a 3-year period from 2011 to 2014 by the introduction of suitable strategies. In 2011, rice was grown during the wet season (June-November) followed by green gram in the entire area of 0.75 ha. During the dry months of 2012, land manipulation was carried out to make permanent raised beds alternating with furrows, called the broad bed and furrow system (BBF), in an area of 0.30 ha. This was done to achieve crop diversification by promoting drainage during the wet season and in-situ rainwater harvesting in the furrows to provide irrigation for dry season crops grown on the raised beds.
Under this method, the original rice field (lowland) was converted into alternating raised beds (4 m width and 60 m length with 1m height) and furrows (6 m width, 60 m length with 1.5 m depth). The bed area was raised by excavating the soil from either side of the bed, and the excavated area was formed into a furrow 6m wide. At the lower end of the furrows, a fish shelter (2 x2 m) was made by further excavating soil up to a depth of 2.5 m (Velmurugan et al., Citation2015). There were 5 raised beds alternating with 5 furrows (a). The raised beds effectively prevented the entry of floodwater into the system, promoted drainage and enabled the cultivation of vegetables throughout the year, while the furrows enabled in-situ rainwater harvesting. After land manipulation in 2012, the soil was kept fallow during the subsequent wet period for removal of any toxic substances. The actual cultivation of vegetable crops has been taken up on raised beds since January 2013, and the green fodder crop Bajra Napier (BN) hybrid was grown on side sloes to stabilize the raised beds. The normal cropping system that was followed in the raised beds and furrows is given in . In the furrows, freshwater catfish such as singhi (Heteropneustes fossilis) and magur (Clarias batrachus) were grown with rice as a rice-fish system without any additional feed. Fishlings of at least 5 cm in size were introduced into the furrows during August every year and were normally harvested in April—May before the onset of monsoon rains. During the dry season or a break in the monsoon season, fish moved into the fish shelter provided at the lower end of every furrow.
Figure 1. Experimental layout and design of BBF. (a) Layout of raised bed and sunken furrow system; (b) Field layout and components of a crop-livestock integrated farming system.

Table 1. General crop/enterprise rotations followed in the crop-livestock IFS system.
In the remaining 0.35 ha of land area, different rice-based cropping systems/rotations have been followed since 2012. To increase nutrient availability and reduce fertilizer use, a green manure crop (Sesbania rostrata) was sown in April—May and incorporated into the soil in June before rice transplantation. Different dry season crops, viz., green gram, maize, sorghum and seasonal vegetables, were grown from December to March after the harvest of rice to meet the household dietary requirements and fodder requirements of the livestock unit. The standard management practices suitable for the islands were followed for all crops and dairy animals (Gangwar & Bandyopadhyay, Citation1996). After the rice harvest, dry season crops were grown utilizing residual moisture, and care was taken to complete the sowing of these crops by mid-January to prevent the chances of crop failure due to terminal drought. To conserve moisture, mulching was performed using rice straw, especially for vegetables. One or two supplementary irrigations from harvested rainwater were also given based on the crop need.
The livestock (dairy) component was introduced in June 2014 by the inclusion of 2 nos of heifers of Holstein Friesian cross-bred cows. The manure/solid waste generated from the animal shed was collected and used for the preparation of compost by mixing with other crop residues or other organic farm wastes generated within the farm. Along the farm boundary,Gliricidia sepium L was grown as a biofuel, which was also used as green fodder for dairy animals, feedstock in the composting unit and for mulching dry season crops for moisture conservation and weed suppression. In addition, arecanut (Areca catechu L), banana (Musa spp.) and coconut (Cocos nucifera) trees were also maintained along the bunds or field boundaries without any additional nutrient management.
By 2014, the crop-livestock IFS was fully established and integrated by the inclusion of all the components and ensuring resource flow between the components. The integrated system was maintained, and observations were taken until 2018. The details of different farm enterprises followed within this system are described in , and the field layout of the experiment after the integration of all the components is depicted in b.
2.3. Climate-smart practices
Within the umbrella of integrated farming systems, different CSA practices included in this study to address the identified challenges are summarized in . These practices are included to address a specific challenge directly or indirectly linked to climate change in the study area.
Table 2. Climate smart practices followed in the study.
2.4 Evaluation of crop-livestock IFS for climate smartness
The crop-livestock integrated farming system was evaluated for climate smartness by assessing productivity, sustainability, food security and reduction in greenhouse gas emissions. The details are described in the following subsections.
2.4.1. Productivity
2.4.1.1. Physical productivity
The productivity of different components was determined based on marketable produce in terms of grains, green fodder, milk, fish, vegetables and fruits. The crop residues and waste generated from the dairy component were also accounted for calculating the production from different farm enterprises. Then, the productivity of different components was converted into equivalent yields for comparison. The productivity of the system was calculated on the basis of rice equivalent yield (REY) by converting the yield of non-rice crops and economic products of other enterprises into equivalent rice yields on a price basis using the formula:
Where Yx is the yield of non-rice crops (kg), Px is the price of non-rice crops (Rs. kg−1), and Pr is the price of rice.
2.4.1.2. Economic returns
The cost analysis including gross returns, cost of production and net returns were calculated as per the standard procedures (Sanjeev et al., Citation2012) and reported in USD by taking the average value of Indian rupee during that particular year.
2.4.2. Sustainability
The sustainability of crop-livestock IFS and different farm enterprises was evaluated using the sustainable yield index (SYI) (Sanjeev et al., Citation2012), which was estimated as follows:
where, AY – Average rice grain equivalent yield of the respective enterprise or system; Sd – Standard deviation; YMax – Maximum yield obtained from the respective enterprise or farming system.
2.4.3. Food security
Although food security is a multidimensional concept, some food security indicators, such as availability (physical supply), accessibility and diversity of food for the household, were measured by on-farm food production per capita (household) and the farm-level diversity index. On-farm food production was compared with dietary requirements prescribed by the Indian Council of Medical Research (ICMR, Citation2011) based on the guidelines of the World Health Organization (WHO).
One of the basic aims of this study was to diversify food production from rice to other essential food items, such as maize, pulses, oil seeds, vegetables, fruits, milk and fish products, within the farm by suitable diversification strategies enabled by specific land management techniques. This is essential to meet the dietary requirements of farm families through on-farm production. Hence, farm-level diversity assessment was performed using Simpson’s diversity index (Kumar et al., Citation1994; Simpson, Citation1949) which is defined as,
Farm diversity index 2.
where S is the number of species or activities that are present, ni (for i = 1 to S) is the area devoted to the ith species or activity or income of the ith activity, and N ( = Sum of ni) is the gross total area across all the activities or total farm income. For a farm with only one species or activity with no diversity, the farm diversity index (FDI) is zero. As farm diversity increases, FDI approaches unity.
2.4.4. Estimation of greenhouse gas (GHG) emission
GHG emissions from different components of the farming system were calculated using the IFS-GHG Estimation Tool developed by the ICAR-Indian Institute of Farming Systems Research (Subash, et al., Citation2018). This tool allows the farm-scale-level estimation of GHG emissions from different components from production to harvest. The tool consists of a generic set of empirical models that are used to estimate farm-level product emissions based on life cycle assessment (LCA) using the Tier 2 approach of the IPCC,(1997), where the emission sources are broken down into different categories for convenient quantification of major emissions of CO2, CH4 and N2O. The tool uses national-level emission factors for different crops, land uses and livestock in emission calculations (AbbaChhabra et al., Citation2013; IPCC, Citation2007; MOEF, Citation2004; Swamy & Bhattacharya, Citation2006). The GHG emissions from farm-level management were also estimated, including farm machinery and the application of agrochemicals such as fertilizers and pesticides. In this tool, the GHG emissions are reported in CO2 equivalent per unit of crops and per head for livestock using the 100-year global warming potentials used in national GHG accounting (IPCC, Citation2006). In the present study, emissions were estimated from thirteen input sections that dealt with each enterprise of the farming system (calculation and data are given as S File GHG calculator). This tool also allows the user to account for the carbon credit potential of the system as a whole and compare it with other farming practices.
3. Results and discussion
3.1. Enhanced Productivity
In a broader sense, the foremost objective of climate-smart agriculture is to increase farm production and income even in the presence of adverse situations through technological interventions and by making suitable adjustments in the system. This was evidenced in the present study, as the total farm productivity increased approximately 11 times (2.8–35.6 t ha−1) from 2011 to 2018 by various interventions, such as crop and enterprise diversification, cropping system intensification enabled by suitable land management (). Cultivation of a long-duration photosensitive rice variety (C 14–8) during the monsoon season recorded only 2.8 t ha−1 in 2011, which was the existing practice. In subsequent year (2012), this variety was replaced with long-duration high-yielding rice varieties (CARI 5, CARI 7 and CSR 36) as a part of climate-smart interventions, which resulted in increased rice productivity up to 4.3 t ha−1,representing a 53% increase over traditional rice cultivation. In addition the total farm productivity was enhanced to 8.0 t ha−1 (REY) by the inclusion of dry season crops,viz., maize, green gram, ground nut and okra, in the cropping system after the harvesting of rice and the conversion of crop residues and farm wastes into compost. The production system was further intensified in 2013 by growing seasonal vegetables throughout the year on the raised beds of the BBF, which recorded a 100% increase in productivity (16.1 t ha−1) compared to the previous year. The construction of raised beds and furrows in part of the rice monocropped area enabled the growth of vegetables on the raised beds by facilitating the removal of excess water in otherwise waterlogged ecosystems. Moreover, life-saving irrigation was provided to the vegetable crops grown in the raised beds during dry months so that the land was effectively utilized and the productivity of the same unit of land was improved (Velmurugan et al., Citation2015).
Table 3. Production and net return from different components of crop-livestock IFS over the years.
Another stimulus to the total farm production was the introduction of dairy livestock (2 milch cows) in 2014 supported by the inclusion of fodder in the cropping system, which resulted in a 120% increase in total farm productivity (35.7 t ha−1) compared with the previous year. After completion of all the interventions and integration of different farm enterprises by resource recycling, the realized mean productivity of the crop – livestock IFS during 2014–2018 was recorded as 37.6 t ha−1 under island conditions. This was due to enterprise diversification and the integration of different farm enterprises that provided an opportunity to increase total farm productivity by efficient utilization of resources and waste reduction through resource recycling in addition to imparting stability (Sanjeev et al., Citation2012). With the integration of different components and optimization of resources the production system progressed towards climate smart IFS from subsistence level (). This process also resulted in the efficient use of time, space and harvested rainwater in addition to ensuring on-farm food availability (physical supply). Similar results of enhanced productivity under crop-livestock IFS were also reported by several researchers (Gajender, et al., Citation2021; Ravisankar et al., Citation2007) on small and marginal landholdings in different agro-eco regions of India.
Figure 2. Relationship between system yield (REY) and diversity index which increased with the progression of integration of different IFS components.

Understandably increased farm production led to 10 fold increase in annual net return barring market fluctuations from USD 359 in (2011) to USD more than 3500 after 2014 () with average net return of USD 3631.2 during 2014-2018. The integration of dairy component in 2014 witnessed steep increase in total farm production and efficient recycling of resources that reflected in enhanced net return followed by its stabilization. Though, the crop component witnessed loss of returns due to flood, dry spells and pest incidences during the experimental period total net return from the IFS system significantly enhanced and sustained, primarily due to climate smart agricultural practices followed. The loss from crop component was very well compensated by livestock component with mean contribution of 58.4% to total net returns over the period. Comparable increase in farm production and net return as a result of crop diversification was also reported by Paramesh et al. (Citation2019) in coastal lowlands of Goa, India.
3.2. Enhanced resilience and adaptive capacity.
Imparting resilience and enhancing the adaptive capacity of the production system are the most desirable impacts expected from smart-climate practices. These goals can be reached by developing a robust system that is productive, profitable, and sustainable across a wide range of perturbations (Urruty, et al., Citation2016). In the present study, the system was made robust by diversifying agriculture and introducing smart combinations of technologies and approaches. As the stability of the production system reflects resilience, the sustainable yield index (SYI) was calculated for different farm enterprises and for the entire system.
The SYI for Crop-Livestock IFS was 0.84 () compared to 0.28 for the fisheries component because of wide fluctuations in productivity, as the coastal lowlands are highly vulnerable to flooding. Furthermore, fisheries as rice-fish systems in furrows of BBF have received less attention, as the main focus has been on in-situ rainwater harvesting, which will support the food and nutritional security of farm families without any additional cost. The dry season crops grown after harvesting rice in rice-based cropping systems and vegetable production in the BBF system recorded SYI values of 0.64 and 0.67, respectively, while rice performed better, at 0.68. Though the productivity of rice was low, but it was stable under waterlogged conditions. At the same time it could not provide higher return and support food requirements due to lack of diversification. The success of dry season crops depends on the sowing time and availability of water during the growing period. Off-season rainfall sometimes affected crop yield, as happened in March 2015. Likewise, delays in sowing after mid-January, as occurred in January 2015, resulted in poor yield due to terminal drought.
Although vegetable cultivation on raised beds is intensive and highly productive, it is more vulnerable to climatic parameters and water availability during dry periods. In normal years, 3 successive crops were grown by proper planning and optimum utilization of resources. However, any delay in the onset of monsoons and/or the sudden onset of monsoons after a prolonged dry spell leads to crop failure because sowing or transplanting could not be undertaken during April–May due to a lack of optimum soil moisture for field operations. Sometimes, flooding and waterlogging also affect the crop yield during the monsoon season. In contrast, the productivity of rice was stable (0.68) during the period, as it is best adapted for coastal lowlands except for biotic stresses, as seen during 2017 and 2018. Infestation by ear head bugs (Leptocorisa acuta) resulted in yield losses of 40% to 50%. Although crop components showed fluctuations in productivity from 2014 to 2018, the overall farm productivity increased because of growth in dairy production, thereby compensating for the loss. The results indicated that livestock imparted greater stability (0.80) to the overall system, as it was less affected by climatic factors such as floods and water scarcity. The strong and positive interlinks between components with different CSA practices supported the sustainability of the overall system, as reflected in the higher SYI (0.89). Crop diversification and the integration of animal components play a major role in providing sustainability to smallholder farms (Loboguerrero et al., Citation2019; Sanjeev et al., Citation2012).
The integration of different component enterprises within the farm was achieved when there is source flow between the components. The analysis of resource flow () between the components of crop-livestock IFS showed effective recycling of farm wastes, efficient resource use and increased marketable surplus. Over the years this resulted in increased productivity and sustainability of the production system. Further, effective recycling of farm wastes contributed to significant reduction in greenhouse gas emission by way of replacing synthetic fertilizer use and increased carbon sequestration in the form of soil organic carbon.
The inclusion of climate-smart practices in the crop-livestock IFS as evidenced from these results, greatly improved system resilience and adaptive capacity against the observed climate variations and adverse edaphic factors prevailing under humid tropical island conditions. For example, livestock acted as a buffer to reduce the risk associated with crop production, such as crop failures due to unforeseen events (cyclones, flooding, drought, etc.). They also represent liquid assets that can be realized at any time, adding further stability to the production system (Sansoucy, Citation1995). Furthermore, the adaptive capacity of the farm was greatly improved by land manipulation (raised beds and furrow system), which improved drainage during the wet season, facilitating vegetable cultivation on the beds. Similar increases in yield and sustainability through different land manipulation methods were reported by several authors in coastal salt-affected soils of India (Mandal et al., Citation2018; Velmurugan et al., Citation2015).
3.3. Food and nutritional security
The on-farm production of food items was diversified from rice monocropping as a part of the crop and enterprise diversification as a measure of the CSA strategy. The results showed that on-farm production of food items significantly increased and was diversified from 1.2 t of rice grain in 2011–1.4 t of cereals (rice, maize), 0.03 t of pulses, 0.12 t of oil seeds, 3.2 t of vegetables, 0.2 t of fruits, 2.9 t of milk and 0.02 t of fish in a year from a 0.75 ha land unit, which meet the food and nutritional expectations of a farm household (). The on-farm production of various food items was compared with the dietary requirements of a 5-member family to assess the potential of the climate-smart integrated farming system to meet the household food demand prescribed by ICMR (Citation2011). The results showed that crop-livestock IFS enabled the on-farm production of major food items required for a normal diet able to meet family food requirements and diet diversity per the recommended level (). Except for pulses and oil seeds, all other food requirements of the farm family were satisfied from the production system itself.
Figure 5. Glimpses of different components of climate smart IFS that provides food security evaluated for tropical island conditions. a. Vegetables in the raised beds, rice + fish in the furrows, fodder + agroforestry along boundries with dairy component; b. Land preparation and simultaneous sowing on the raisded bed even during monsoon season; c. Cultivation of improved rice varieties instead of photosensitive cultivar during wet season; d. Maize + pulse after rice harvest during dry season increased the cropping intensity.

Table 4. On-farm production of various food items from the crop-livestock IFS over the years and household food security.
The system produced a significantly higher marketable surplus of some food items, such as vegetables and milk, which was able to provide good returns and cash on hand. Furthermore, the diversity and quantity of required food items measured by the farm-level diversity index increased from 0.0 (rice monocrop) in 2011–0.86 in 2018. Several studies reported enhanced food security through improvements in the quantity and variety of food stocks and growth in income through the sale of farm produce, which was used to further improve consumption patterns among smallholders (Swarnam et al., Citation2014) by crop or enterprise diversification strategies (S. Figure 2). Similarly,a consistent positive association of farm diversification (represented by the Simpson diversity index) with household dietary diversity was reported by several authors (Adjimoti & Kwadzo, Citation2018; Mango et al., Citation2018), indicating that more diverse production systems can also lead to more diverse household diets. In summary, a crop-livestock IFS as a holistic approach to farm supply management, has the potential to significantly reduce the dependency on external market-based supplies of food (Kumar et al., Citation2018) and enhance the food security of small farm holdings.
3.4. GHG emissions and carbon footprints at the farm-gate level
The assessment of GHG emissions from different farm enterprises within the crop-livestock IFS shows a wide variability of emissions over the years (). This was primarily due to variations in the amount of biomass produced in different crops and agro-forestry components, recycled organic waste and the amount of C sequestered in the soil organic carbon pool (Vermeulen et al., Citation2012). In general, although the total GHG emissions increased with the addition of more components, particularly livestock, net GHG emissions decreased due to a significant increase in the C sink over the years in the IFS. Total GHG emissions increased from 3.21 Mg CO2eqyr−1 in rice monocrop to 6.46 Mg CO2eqyr−1 at the end line, indicating a two-fold increase. However, the inclusion of CSA practices enhanced the C sink through waste recycling and compost additions, standing biomass, green manuring, mulching and improvement in soil C levels. This resulted in a 10-fold increase in the C sink from a meager 0.53 Mg CO2eqyr−1 in 2011–2012–5.93 Mg CO2eqyr−1 at the end line with mitigation benefits of 5.40 Mg CO2eqyr−1. Consequently, net GHG emissions from the system progressively decreased to 0.43 Mg CO2eqyr−1 by the end line. In the present study, manure/crop residue additions and agro-forestry plantations such as Glyricidia sepium, arecanut (Areca catechu L.) and banana along the farm boundaries resulted in huge mitigation benefits.
The study also found significant differences in GHG emissions and C sinks among different components of crop-livestock IFS. Livestock was the largest contributor to total GHG emissions, at 53%, followed by rice paddies (lowland rice) at 31% at the end line. The greatest emissions from livestock were primarily due to enteric emissions and manure management, while the use of synthetic N fertilizers and anaerobic reduction from rice cultivation are the major sources of emissions from crop components (Lipper et al., Citation2014; Sharma & Sharma, Citation2018). In contrast, the diversification of rice with suitable crop rotation and land management in the form of raised beds and furrows significantly decreased GHG emissions. This was evidenced by the significantly lower GHG generated by dry season crops (11%),followed by rice and vegetable cultivation in raised beds (5%),as a percentage of total emissions, indicating their potential to reduce GHG emissions. This was mainly due to the prevention of waterlogging during the wet season and the conversion of paddy lands to arable cultivation by forming raised beds. Similar results of the highest contribution of livestock to total GHG emissions at the smallholder farm level in western Kenya (Seebauer, Citation2014) and western coastal zones of India (Paramesh et al., Citation2019) were also reported.
Although agriculture is shown tobe a net contributor to GHG emissions, increasing food production by the efficient utilization of available resources has been stressed for a long time. Likewise, it is equally important to assess the emission intensity to evaluate the efficiency of the production system. Therefore, in the present study, GHG emission intensity was estimated. It was interesting to observe that the emission intensity was significantly reduced from 1.12 kg CO2eq kg−1 of food produced by the rice monocrop in 2011to 0.18 kg CO2eq kg−1 of food produced by end line (). The most noticeable feature was a steep decline in emission intensity after crop diversification (0.42 kg CO2eq kg−1), followed by a moderate decline with the integration of livestock components. This means that the emission intensity was lower for the crop-livestock IFS than for the rice monocrop system, indicating the contribution of the integrated system to emission reduction while maintaining the highest level of productivity. The lower GHG emissions from climate-smart practices were primarily due to the efficient flow of nutrients and energy (recycling) and the increase in the carbon sink within the system. Given that food production is of the utmost importance under emerging climate change situations, IFS provides scope to identify synergies and trade-offs among food security, adaptation and mitigation measures as a basis for informing and reorienting policy in response to climate change (Lipper et al., Citation2014).
3.5. Contribution of CSA technologies to climate-linked challenges and total farm production
CSA practices such as diversification of agro-ecosystems by way of polyculture, agro-forestry, and crop-livestock IFS accompanied by organic soil management, water conservation, harvesting and general enhancement of farm-level agro-biodiversity strengthened the resilience of smallholder farms. the changes in several climatic variables and extreme events during the experimental period (), CSA practices most likely contributed to climate resilience to withstand diverse, severe, and location-specific challenges and helped to achieve sustained production and enhanced farm-level food and nutritional security. The analysis of the mitigation effect of different CSA practices on climate-linked challenges and to total farm production showed a significant and positive contribution of these practices to climate resilience (). Crop diversification through raised beds (r2 = 1.298**), enterprise diversification, crop rotation, green manuring, in-situ rainwater harvesting, contingency cropping and organic waste recycling made significant contributions to the performance of the crop-livestock IFS. These practices also provided other environmental services. For example, green manuring and organic manure additions not only enhanced the soil C pool from 7.6 g kg−1 at the beginning of the experiment to 9.8 g kg−1 at the end line but also improved the soil nutrient content (Mureithi et al., Citation2003) and greatly reduced the need for synthetic N fertilizers that contribute to GHG emissions; i.e. the application of compost and green manure application to crops contributed approximately 63 kg N, 37 kg P2O5 and 42 kg K2O annually, respectively, with a concomitant reduction in the application of synthetic fertilizers. Neate (Citation2013) also documented similar success stories from around the world. Similarly Gowing, et al. Citation2020 emphasized the role of crop-livestock systems in achieving an acceptable soil nitrogen and phosphorus balance through better use of available livestock manure in typical smallholder farms of Kenya.
Table 5. Contribution of CSA practices to total farm production.
Alternatively, contributions from bio-formulations, mulching and boundary planting included in the crop-livestock IFS were not significant but were important at minimizing the yield loss in adverse situations, reducing the moisture stress on crops and increasing the C sink, thereby reducing net GHG emissions (Sissoko et al., Citation2013). Furthermore, mulching increased soil aggregation (Mulumba & Lal, Citation2008) and protected the soil from direct impact by rainfall, greatly reducing the loss of nutrients and organic matter through soil erosion. These measures most likely contributed to the sustainability of the crop-livestock system (Barton et al., Citation2004). However, some of these advantages are clearly visible and difficult to quantify. Attempts to quantify these effects under island agro-ecosystem might divert the main focus of the current investigation but nevertheless, deserve future research attention.
4. Conclusions
The present study clearly demonstrated the potential for raising the agricultural production and food security of smallholder farmers by managing climate risk at the farm level while improving the resource-use efficiency, productivity and sustainability of the production system by integrating suitable agricultural practices through farming systems approach. CSA practices such as enterprise diversification, crop rotation, green manuring, mulching and organic waste recycling significantly improved farm production. Furthermore, improved land management by making raised beds and furrows facilitated rainwater harvesting and intensive vegetable cultivation, resulting in greater diversity and efficient resource use. The integration of animal components further enhanced the production and sustainability of the system against the climate change under island conditions. The study also indicated a decrease in net GHG emissions and emission intensity with the integration and stabilization of different components. Based on the results, it is suggested that the crop-livestock IFS approach can be up scaled to similar situations in the tropical region as an adaptation strategy to cope with the unfolding climate change scenarios.
Declaration of competing interest
The authors declare that they have no known competing financial interests or personal relationships that could have appeared to influence the work reported in this paper.
Acknowledgements
We sincerely acknowledge the ICAR-IIFSR, Modipuram for financial and technical support for carrying out this study. We also sincerely acknowledge the Director, and all other staff of ICAR-Central Island Agricultural Research Institute, Port Blair for extending all possible support during the investigation.
Highlights...docx
Download MS Word (15.2 KB)Additional information
Notes on contributors
T. P. Swarnam
Dr T. P. Swarnam is working as Head, Division of Natural Resource Management, ICAR-CIARI, Port Blair, India. She has more than 20 years of research experience in island ecosystem, integrated farming system, tribal farming and resource management. Her research interests are organic farming systems, waste recycling, organic novel products, valorisation and climate smart agriculture.
A. Velmurugan
Dr A. Velmurugan is specialized in soil science and resource management. He has worked on soil resource assessment, land degradation management, island and coastal ecosystem, climate change and adaptation, biogeochemical cycles and natural resource management using remote sensing and GIS. Currently he is engaged in research management at Indian Council of Agricultural Research, New Delhi, India.
T. Subramani
T. Subramani is an agronomist, specialized in water management and working in ICAR-CIARI, Port Blair. He has been engaged in farming system studies in islands, nutrient management of high value crops, water management and stress agronomy.
N. Ravisankar
N. Ravisankar is an agronomist, engaged in integrated farming system research. He has rich experience in coordinating and upscaling of IFS and organic production systems. Currently serving as Project coordinator of AICRP – IFS.
N. Subash
N. Subash is specialised in agricultural meteorology, climate change impact on farming system and simulation modelling. He was involved in integrated farming system research and coordination of network programmes.
A. S. Pawar
A. S. Pawar has been actively involved in integrated farming system research for more than 25 years. He also served as Director, ICAR- Indian Institute of Farming System Research, Modipuram, India. He was engaged in research coordination and management.
P. Perumal
P. Perumal is a livestock management specialist. He has been involved in livestock management under stressful conditions and reproduction studies. He has rich experience in animal production under island condition.
I. Jaisankar
I. Jaisankar, is agroforestry specialist, engaged in multipurpose tree production, silvi-pastoral system, biodiversity conservation and bioprospecting under island ecosystem.
S. Dam Roy
S. Damroy has been engaged in fisheries production, conservation and technology generation. His main interest was enhancing fisheries production both marine and fresh water fisheries. He also served as Director, ICAR- Central Island Agricultural Research Institute, Port Blair, India.
References
- AbhaChhabra, M., SushmaPanigrahy, K. R., & Parihar, J. S. (2013). Greenhouse gas emissions from Indian livestock. Climatic Change, 117, 329–344. https://doi.org/10.1007/s10584-012-0556-8
- Adjimoti, G. O., & Kwadzo, G. T. (2018). Crop diversification and household food security status: Evidence from rural Benin. Agriculture & Food Security, 7(1), 82–91. https://doi.org/10.1186/s40066-018-0233-x
- Barton, A. P., Fullen, M. A., Mitchell, D. J., Hocking, T. J., Liu, L., Wu Bo, Z., Zheng, Y., & Xia, Z. Y. (2004). Effects of soil conservation measures on erosion rates and crop productivity on subtropical ultisols in yunnan province, China. Agriculture, Ecosystems & Environment, 104(2), 343–357. https://doi.org/10.1016/j.agee.2004.01.034
- Campbell, B. M., Thornton, P., Zougmoré, R., van Asten, P., & Lipper, L. (2014). Sustainable intensification: What is its role in climate smart agriculture? Current Opinion in Environmental Sustainability, 8, 39–43. https://doi.org/10.1016/j.cosust.2014.07.002
- Carletto, G., Ruel, M., Winters, P., & Zezza, A. (2015). Farm-level pathways to improved nutritional status: Introduction to the special issue. The Journal of Development Studies, 51(8), 945–957. https://doi.org/10.1080/00220388.2015.1018908
- Darnhofer, I., Fairweather, J., & Moller, H. (2010). Assessing a farm's sustainability: Insights from resilience thinking. International Journal of Agricultural Sustainability, 8(3), 186–198. https://doi.org/10.3763/ijas.2010.0480
- Gajender Y., Jat H.S., Raju R., Yadav R.K., Singh, S.K. Chaudhari, S.K., Sharma, D.K., Gurbachan Singh, Sharma, P.C., 2021. Enterprise mix diversification: An option for ecologically sustainable food and nutritional security of small holders in Indo-Gangetic plains. International Journal of Agricultural Sustainability, 20:1, 31–41. https://doi.org/10.1080/14735903.2021.1912978
- Gangwar, B., & Bandyopadhyay, A. K. (1996). Agricultural production manual for andaman and nicobar islands. Central Agricultural Research Institute. p. 86.
- Gowing, J. W., Golicha, D. D., & Sanderson, R. A. (2020). Integrated crop-livestock farming offers a solution to soil fertility mining in semi-arid Kenya: evidence from Marsabit County. International Journal of Agricultural Sustainability, 18(6), 492–504. https://doi.org/10.1080/14735903.2020.1793646
- ICMR, 2011. Dietary guidelines for Indians – a manual, National Institute of Nutrition, Indian Council of Medical Research, The Ministry of Health and Family Welfare, GOI and WHO, Rome. p. 127
- IFAD, 2013. Smallholders, food security, and the environment. International Fund for Agricultural Development. Via Paolo di Dono, 44–00142 Rome, Italy. p. 56. www.ifad.org
- IPCC. (2006). IPCC guidelines for national greenhouse Gas inventories - 2006, prepared by the national greenhouse Gas inventories programme. In H. S. Eggleston, L. Buendia, K. Miwa, T. Ngara, & K. Tanabe (Eds.), Intergovernmental panel on climate change (pp. 1–20). IPCC/IGES. https://www.ipcc-nggip.iges.or.jp/public/2006gl/
- IPCC. (2007). Climate change 2007: Impacts, adaptation and vulnerability. In M. L. Parry, O. F. Canziani, J. P. Palutikof, P. J. van der Linden, & C. E. Hanson (Eds.), Contribution of working group II to the fourth assessment report of the intergovernmental panel on climate change (pp. 469–506). Cambridge University Press.
- IPCC, 2014.Climate Change 2014: Impacts, adaptation, and vulnerability. Working group 2 contribution to the fifth assessment report of the intergovernmental panel on climate change, Geneva, Switzerland, 151 pp.
- Joshi, P. K., & Tyagi, N. K. (2019). Small farm holders and climate change: Overcoming the impacts in India. In B. Pal, A. Kishore, P. Joshi, & N. Tyagi (Eds.), Climate smart agriculture in south Asia (pp. 49–72). Springer.
- Kumar, B. M., George, S. J., & Chinnamani, S. (1994). Diversity, structure and standing stock of wood in the homegardens of Kerala in peninsular India. Agroforest. Syst, 25, 243–262.
- Kumar, S., Bhatt, B. P., Dey, A., Shivani Kumar, U., & Idris, M. (2018). Integrated farming system in India: Current status, scope and future prospects in changing agricultural scenario. Indian J AgricSci, 88, 13–27.
- Kurgat, B. K., Stöber, S., Mwonga, S., Lotze-Campen, H., & Rosenstock, T. S. (2018). Livelihood and climate trade-offs in Kenyan peri-urban vegetable production. Agricultural Systems, 160, 79–86. https://doi.org/10.1016/j.agsy.2017.10.003
- Lipper, L., Thornton, P., Bruce, M., Campbell, T. B., AdemolaBraimoh, M. B., Caron, P., Cattaneo, A., Garrity, D., Henry, K., Hottle, R., Jackson, L., Jarvis, A., Kossam, F., Mann, W., Nancy McCarthy, A., Neufeldt, H., Remington, T., ThiSen, P., Sessa, R., … Torquebiau, F. (2014). Climate-smart agriculture for food security. Nature Climate Change, 4(12), 1068–1072. www.nature.com/natureclimatechange.
- Loboguerrero, A. M., Bruce, M., Campbell, P., Cooper, J. M., Hansen, J. W., Rosenstock, T., & Wollenberg, E. (2019). Food and earth systems: Priorities for climate change adaptation and mitigation for agriculture and food systems. Sustainability, 11(5), 1372. https://doi.org/10.3390/su11051372
- Mandal, S., Burman, D., Maitra, N. J., Ghoshal, T. K., Velmurugan, A., Mandal, B., & Mahanta, K. K. (2018. ). Doubling farmers’ income through land-shaping technologies in coastal-salt affected areas. Indian Farming, 68(03), 31–34.
- Mango, N., Makate, C., Mapemba, L., & Sopo, M. (2018). Role of crop diversification in improving household food security in central Malawi. Agric. Food Secur, 7. https://doi.org/10.1186/s40066-018-0160-x.
- MOEF. (2004). India’s initial national communication to the united nations framework convention on climate change. New Delhi: NATCOM report. Ministry of environment and forests, government of India.
- Mulumba, L. N., & Lal, R. (2008). Mulching effects on selected soil physical properties. Soil and Tillage Research, 98(1), 106–111. https://doi.org/10.1016/j.still.2007.10.011
- Mureithi, J. G., Gachene, C. K. K., & Ojiem, J. (2003). The role of green manure legumes in smallholder farming systems in Kenya: The legume research network project. Trop. Subtrop. Ecosyst, 1, 57–70.
- Neate, P. (2013). Climate smart agriculture success stories from farming communities around the world. CCAFS; Technical Centre for Agricultural and Rural Cooperation. https://cgspace.cgiar.org/rest/bitstreams/24750/retrieve.
- Paramesh, V., Parajuli, R., Chakurkar, E.B., Sreekanth, G.B., Chetan Kumar, H.B., Gokuldas, P.P., Mahajan, G.R., Manohara, K.K., Viswanatha, K.R., Ravisankar, N., 2019. Sustainability, energy budgeting, and life cycle assessment of crop-dairy-fish-poultry mixed farming system for coastal lowlands under humid tropic condition of India. Energy 188:116101. https://doi.org/10.1016/j.energy.2019.116101
- Ravisankar, N., Pramanik, S. C., Jeyakumar, S., Singh, D. R., NabisatBibi, S. N., & Tapan, K. B. (2007). Study on integrated farming system (IFS) under different resource conditions of island ecosystem. Farming System Research & Development, 13(1), 1–9.
- Richards, M.B., Wollenberg, E. Buglion-Gluck, S., 2015. Agriculture’s contributions to national emissions; Ccafs info brief; CGIAR research program on climate change, agriculture and food security (CCAFS): Copenhagen, Denmark. https://hdl.handle.net/10568/68841 (accessed on 5th March 2020).
- Sanjeev, K., Subhash, N., Shivani, S., Singh, S. S., & Dey, A. (2012). Evaluation of different components under integrated farming system (IFS) for small and marginal farmers under semi-humid climatic environment. ExplAgric, 399–413. https://doi.org/10.1017/S0014479712000087
- Sansoucy.R., 1995. Livestock - a driving force for food security and sustainable development. World Animal Review, 84/85, pp. 5-17. FAO. Accessed 20 January, 2020. http://www.fao.org/3/v8180t/v8180T07.htm
- Seebauer, M. (2014). Whole farm quantification of GHG emissions within smallholder farms in developing countries. Environmental Research Letters, 9(3), Article 0035006. 13pp. https://doi.org/10.1088/1748-9326/9/3/035006
- Sharma, U. C., & Sharma, V. (2018). Greenhouse gases emissions from agriculture sector in northeastern region of India. Acta Scientific Microbiology, 1, 36–43.
- Simpson, E. (1949). Measurement of diversity. Nature, 163(4148), 688. https://doi.org/10.1038/163688a0
- Sissoko, F., Affholder, F., Autfray, P., Wery, J., & Rapidel, B. (2013). Wet years and farmers’ practices may offset the benefits of residue retention on runoff and yield in cotton fields in the Sudan-Sahelian zone. Agricultural Water Management, 119(1), 89–99. https://doi.org/10.1016/j.agwat.2012.12.012
- Subash, N., Dutta, D., & Ravisankar, N. (2018). IFS-GHG Estimation Tool Ver. 1.0. A green house gases estimation tool for integrated farming system models. AICRP-IFS, ICAR-IIFSR, Modipuram, Meerut, India.
- Swamy, M., & Bhattacharya, S. (2006). Budgeting anthropogenic greenhouse gas emission from Indian livestock using country-specific emission coefficients. Curr. Sci, 91(10), 1340–1353. https://www.jstor.org/stable/24094058
- Swarnam, T. P., Velmurugan, A., Zacharia, G., & Dam Roy, S. (2014). Integrated farming system for sustainable livelihood in tribal areas of Nicobar Island, India. Journal of the Andaman Science Association, 19(1), 19–22.
- The Wageningen Statement. (2011). Science into Action: Wageningen Statement on Climate-Smart Agriculture, 26th October 2011. https://www.wur.nl/en/show/Science-into-Action-Wageningen-Statement-on-Climate-Smart-Agriculture.htm. Accessed on 10th January 2020.
- The World Bank. (2019). Climate-smart agriculture. https://www.worldbank.org/en/topic/ climate-smart-agriculture. Accessed on 15th March 2020.
- Tubiello, F. N. 2013. The FAOSTAT database of greenhouse gas emissions from agriculture. Environ. Res. Lett. 8, 1–10. https://iopscience.iop.org/article/10.1088/1748-9326/8/1/015009
- Urruty, N., Tailliez-Lefebvre, D., Huyghe, C. 2016, Stability, robustness, vulnerability and resilience of agricultural systems. A Review. Agron. Sustain. Dev. 36(1), 1–15. https://doi.org/10.1007/s13593-015-0347-5
- Velmurugan, A. (2018). The nature and characters of tropical islands. In C. Sivaperuman, A. Velmurugan, A. K. Singh, & I. Jaisankar (Eds.), Biodiversity and climate change adaptation In tropical islands (pp. 3–30). Elsevier-Academic Press.
- Velmurugan, A., Swarnam, T. P., & Lal, R. (2015). Effect of land shaping on soil properties and crop yield in tsunami inundated coastal soils of southern andaman island. Agriculture, Ecosystems & Environment, 206, 1–9. https://doi.org/10.1016/j.agee.2015.03.012
- Vermeulen, S. J., Campbell, B. M., & Ingram, S. J. I. (2012). Climate change and food systems. Annual Review of Environment and Resources, 37, 195–222.