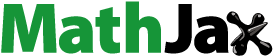
ABSTRACT
Waste management is an increasing global concern due to the rise in urbanization and industrialization. This study examines the co-pyrolysis of date pits (DP) and single-use plastics (polypropylene-PP and Styrofoam-PS) as a way to create value-added products. Single, binary, and ternary mixtures were pyrolyzed at three different heating rates to understand the synergy, kinetics, and thermodynamics. The results showed positive synergy and reduced activation energies during co-pyrolysis, due to varying reaction mechanisms. The positive values of ∆H, ∆G, and negative ΔS indicate endothermic non-spontaneous reactions and disorder in the products instead of the reactants during pyrolysis. Future studies should explore upscaling this process for sustainable energy production.
1. Introduction
Due to urbanisation, industrialisation, and associated waste generation, waste management is an increasing global concern. Predictions by the World Bank show the annual production of municipal solid waste (MSW) – comprised mainly of organic wastes, and plastics – will double by 2025 from 1.3 million tons in 2012 (Das et al. Citation2019). The most common methods of disposing of waste is landfilling and incineration. However, economical and straightforward, they pose severe health and environmental issues due to emissions to both air and water bodies and lack of management (Das et al. Citation2019; Foong et al. Citation2020). In addition to the associated environmental regulations, the lack of land space for landfilling presents an imperative need for other ways to treat waste (Parthasarathy et al. Citation2022). A thermochemical processing technology – pyrolysis – has recently gained attention due to its being environmentally friendly. The products received from pyrolysis include char, oil, and gas, depending on the operating conditions, such as feedstock type, heating rate, temperature, solid residence times, and particle size (Foong et al. Citation2020). The products have proved the technology economical, providing an alternative to landfilling.
Thermogravimetric analysis (TGA) is often used to understand and evaluate the effects of heating rates on the kinetic parameters and thermodynamic parameters, thereby providing useful guidance on designing pyrolysis systems (Mariyam et al. Citation2022). The data received from TGA reflects changes in materials’ weight loss over time and temperature (Elkhalifa et al. Citation2022). Several studies have conducted TGA analysis of various mixed feedstocks, including lignocellulosic biomass food wastes (Fermanelli et al., Citation2020) and swine manure with plastic wastes (Ro et al., Citation2014), depicting co-pyrolysis systems. A positive synergy was observed when pine sawdust was mixed with low-density polyethylene (LDPE) due to lowered activation energies (AE) and solid residues (Zheng et al. Citation2018). Subsequently, Wen et al. found that increasing the polyethylene (PE) fraction from 0 to 75% increased cardboard decomposition, with increasing AE with temperature (Wen et al. Citation2021). On the other hand, when sewage sludge (SS) and LDPE were co-pyrolyzed, it was found that the blending ratios of SS and LDPE showed that a 1:1 ratio of the biomass and plastic (SL:50) is optimal in decreasing AE, owing to radicals from LDPE accelerating the biomass pyrolysis (Zaker et al. Citation2021). Kinetic and thermodynamic studies using model-free kinetic models found that AE, Gibbs free energy, and lower enthalpy indicated great potential for bioenergy production. Therefore, plastics are suitable and attractive feedstocks for co-pyrolysis with biomass. Co-pyrolyzing microalgae (Dunaliella salina) with plastics such as polypropylene (PP), polystyrene (PS), polyethylene terephthalate (PET), and polyvinyl chloride (PVC) showed that the solid residues and AE decreased but differently for different plastics (PET > PP > PS > PVC) (Chen et al. Citation2021). Therefore, different plastics have different effects when co-pyrolyzed. Other studies show that co-pyrolyzing several plastics with pinewood produced synergistic effects aiding in better carbon conversion efficiencies and volatiles yield due to higher AE from biomass decomposition (Burra and Gupta Citation2018). The TGA studies demonstrated that although some plastics showed synergy during the TGA of PET and black polycarbonate (BPC), others do not show such behavio (PP).
Several reviews have been published to direct the research using plastic wastes via pyrolysis (Yuan et al. Citation2022; Papari, Bamdad, and Berruti Citation2021). Studies finalise the positive outcomes of mixing biomass and plastics for products generation.
The wastes of interest in this research are date pits (DP) and single-use plastics – polypropylene (PP) and expanded polystyrene (PS). Dates are significant as date palms are among the few fruits bearing trees in arid regions. There are about 120 million date palm trees globally, and 10% of the date fruit comprises DP, which is often thrown away (Tahir, Al-Obaidy, and Mohammed Citation2020). On the other hand, plastic is a modern synthetic product whose production has increased from 1.7 million metric tonnes in 1950–360 million tonnes metric tonnes in 2018, with almost half of it being single-use plastics and 40% of it being used for packaging materials (Chen et al. Citation2021). Both PP and PS or Styrofoam are extensively used in the packaging industry, especially for food and beverages. Styrofoam is often used for takeaway food packaging and cutlery, and on the other hand, PP is widely used as cold beverage holders and lids. Both date and plastic wastes have immense potential for product generation and are ideal candidates for co-pyrolysis using sustainable feedstock blends. Recently, there have been TGA studies published on date wastes (Parthasarathy et al. Citation2022; Raza et al. Citation2022), PP (Jiang et al. Citation2022; Dubdub and Al-Yaari Citation2020), Styrofoam or expanded PS (Varma et al. Citation2021; Zhang et al. Citation2021) and co-pyrolysis of biomass and plastics (as discussed above). However, mixing DP with two different single-use plastics in binary and ternary blends has not been studied previously. This study is essential to understand the thermal degradation behaviour of feeds individually and together under the influence of different heating rates under inert conditions. Additionally, to the best of our knowledge, based on literature surveys, this paper reflects the gap in studying the kinetics and thermodynamics of blending DP in binary and ternary mixtures with single-use plastics.
Most studies focus on iso-conversational models, such as Flynn and Wall, Kissinger, Ozawa, and Friedman due to the lack of reaction model assumption. However, the models are built on the assumption that there is a constant conversion value as a temperature function. Also, they cannot provide kinetic descriptions of complex feeds, so model-free methodologies are preferred (Dhyani and Bhaskar Citation2018). This study's primary focus will be to understand the reaction mechanism based on a model-fitting approach using non-isothermal models to understand the complex effects of mixing biomass (DP) with single-use-plastics of PP and PS. Since most studies focus on model-free kinetics, understanding reaction mechanisms when mixing biomass and plastics becomes imperative. Although an advantage of the model-based approach is utilising a single heating rate, this study also focuses on the change in reaction mechanisms due to the heating rate. The Coats Redfern is an integral model-fitting method utilised to understand seventeen reaction mechanisms based on reaction order, diffusion, geometric, and nucleation models; hence, the effect of an important operating parameter on the reaction mechanism due to complex feed co-pyrolysis is studied. The data from this study will help provide valuable information in designing pyrolysis systems of biomass with two single-use plastics since the TGA shows the kinetics, thermodynamics, volatiles, and char yields, therefore, assisting in sustainable energy production via co-pyrolysis of common wastes.
Therefore, this research work has three main objectives: (i) to understand the thermal decomposition behaviour and synergistic effects and using the three wastes individually (DP, PP, and PS) and in binary and ternary blends during co-pyrolysis; (ii) to study the kinetics of the single, binary and ternary blends using equal masses at different heating rates and applying model-based reaction models of Coats Redfern; and (iii) to study the thermodynamics parameters of the samples under the conditions for the best kinetic fit mechanisms.
2. Materials and methods
According to the objectives of this study, the pyrolysis and co-pyrolysis of DP, PP and PS are studied, and the methodology follows the order (i) material preparation and characterisation (Section 2.1) (ii) synergistic studies (section 2.2) (iii) Model based kinetic studies (Section 2.3) (iv) thermodynamic analysis (section 2.4).
2.1 Materials preparation and characterisation
DP was extracted from commercially purchased dates (Doha Dates, National Food Co.) washed with distilled water, and dried at 323.15 K for 24 h before grinding using an electric grinder. PP and PS were purchased as food packaging materials from the market. Both PP and PS were washed, dried, and ground into small pieces for experimentation purposes. All samples were sieved to 710 µm using the sieve bank supplied by Haver & Boecker, Germany. For mixed wastes, each feedstock was mixed in equal proportions (50 wt.% each) to yield a total of seven samples in this study: single (DP, PP, PS), binary (DP:PP, DP:PS, PP:PS), and ternary (DP:PP:PS).
The proximate analysis of the samples was conducted using a thermogravimetric analyzer (SDT650, TA Instruments). A standard procedure of ASTM D7582-12 was followed, and the moisture, volatiles, fixed carbon, and ash were determined. Approximately 9 ± 0.1 mg of the samples was heated from room temperature to 378.15 K in an inert nitrogen environment for 30 min to evaluate the moisture content. Furthermore, the temperature was increased to 1223.15 Kat a rate of 30 K/min, and the sample was kept at this temperature for seven minutes under isothermal conditions. Then oxygen was used to combust the samples for 10 min to evaluate the ash content. The amount of fixed carbon was calculated on a weight basis by subtracting the measured components (moisture, volatile matter, and ash) from the original mass.
The ultimate analysis was conducted using a CHNS elemental analyzer (EA3000 CHNS elemental analyzer, EuroVector). A standard procedure of ASTM D 3176–8 was followed to analyse the weight percentage of carbon, hydrogen, nitrogen, oxygen, sulfur, chlorine, and ash in each sample of feedstock. Triplicate samples of about 0.5–1.5 mg of each mass were measured and analyzed. The oxygen content was calculated by subtracting the elements’ weight and ash content obtained from the samples’ total mass.
Before the TGA studies, the samples were sieved using a 710 µm sieve (Haver & Boecker, Germany). The analysis was conducted at 10, 20, and 30 K/min heating rates from room temperature to 1173.15 K using a thermogravimetric analyzer (SDT650, TA Instruments) for the single, binary and ternary samples amounting to a total of 21 runs. The binary and ternary mixtures employed the feeds at 1:1 and 1:1:1 ratio. The runs were performed under an N2 atmosphere purged at 100 ml/min. Each run was conducted three times to ensure the reproducibility and accuracy of results. The data from the TGA and derivate thermogravimetric (DTG) curves obtained from the analysis help study the feeds’ synergistic, kinetics and thermodynamics.
2.2 Synergistic studies
The synergistic effects are studied based on the additive formula in Equationequation (1(1)
(1) ) employed previously to understand the impact of biomass and various plastics (Jiang et al. Citation2022; Hassan, Hameed, and Lim Citation2020; X. Wang et al. Citation2019; Alam et al. Citation2020) for two feedstocks. Pyrolysis usually produces char and volatiles.
(1)
(1) Where X1, and X2 are mass fractions of individual feedstocks in the blend and W1, W2, are the residual weight % from the TGA of the individual samples. The equation has further been updated to include three feedstocks for the ternary samples using Equationequation (2
(2)
(2) ):
(2)
(2)
X3 and W3 are the mass fraction and residual weight % of the third feedstock in the ternary blend. While a ΔW (difference in weight percentage of experimental and calculated TGA data) greater than zero reflects a positive synergistic effect (promotion) in the solid-state, less than zero reflects a passive synergy (inhibition). ΔW at the final temperature (ΔWfinal) and throughout the temperatures are analyzed in this study.
Alternatively, overall synergistic effects during co-pyrolysis of the studied feedstocks were studied using a simple statistical analysis through the root mean square error (RMSE) as in Equationequation (3(3)
(3) ). An RMSE value of zero reflects no synergistic effect during the co-pyrolysis process. The correlation coefficient (r) is calculated using Equationequation (4
(4)
(4) ) and should be between – 1 and 1. In the case that the value of r equals 1 and less than 1, in this way, the absence and presence of a synergistic effect is confirmed.
(3)
(3)
(4)
(4)
2.3 Model-based kinetic studies
The rate kinetics help to understand the energy requirement for the pyrolysis system and help determine the reactor size (Varma et al. Citation2021). The following equation describes the general kinetic equation for the kinetic behaviour of biomass, and plastic transformation is heterogeneous in solid states and at a linear heating rate (Zheng et al. Citation2018).
(5)
(5) Developing kinetic models to describe the pyrolysis process via kinetic parameters such as activation energy (AE) and pre-exponential or frequency factor (A) is essential for a pyrolysis reactor design. Although many models using iso-conversional methods of Kissinger – Akahira – Sunose (KAS), Flynn – Wall – Ozawa (FWO), Friedman, and Starink have been employed to study co-pyrolysis of various biomass with different types of plastics (Zaker et al. Citation2021; Özsin, Pütün, and Pütün Citation2019; Varsha et al. Citation2021; Singh et al. Citation2021; Alam et al. Citation2020), this study uses a model-fitting correlation model, namely, the Coats-Redfern (integral) model (CR). This model is chosen due to its applicability in predicting and correlating the effect of single heating rates. Table S1 shows the different solid-state kinetic models based on the CR models used in this study and employed by other studies (Elkhalifa et al. Citation2022; Coats and Redfern Citation1964).
The rate of non-isothermal solid decomposition can be depicted by equations (Equation6(6)
(6) ) and (Equation7
(7)
(7) ).
(6)
(6)
(7)
(7) where A (min−1) is the pre-exponential factor, AE (kJ/mol) is the activation energy, k (T) is the reaction rate constant, t (min) is time, T (K) is the absolute temperature, R (J/mol K) is the universal gas constant, α is the pyrolysis conversion factor given by the Equationequation (7
(7)
(7) ). The value of α and T is determined from the TGA and DTG data. ∝ is expressed as:
(8)
(8) Where
is the weight of the sample before decomposition (kg),
is the weight of the sample at a time t (kg), and
is the final weight after decomposition (kg). For a constant heating rate, β = dT/dt, Equationequation (8
(8)
(8) ) is the repositioned and integrated to give an equation based on the original CR equation (Coats and Redfern Citation1964):
(9)
(9)
Α linear regression model is developed on Microsoft Excel, and the linear regression formula is in the form of . The linear relationship between ln g (
) /T2 and I/T provides the AE and A from the slope and intercept of the equation, respectively. The authors studied seventeen reaction mechanisms of the CR models, based on reaction order, power law, diffusion, nucleation, and geometrical contraction models, which are shown in Table S1. Satisfactory AE and A (reported in kJ/mol and s−1) values were received from models which depicted the highest coefficient of determination, R2,, to the fitted regression line. The modeling is performed in regions with the highest weight loss, as depicted in the DTG curves.
The effect of mixing biomass and plastic feeds on the degradation of the pseudo components was also analyzed using the deconvoluted DTG curves. The deconvolution of the feeds using asymmetric peak fitting function – bi-gaussian – are represted in equations Equation10(10)
(10) and Equation1
(1)
(1) 1. The asymmetrical peaks obtainedalign with hemicellulose, cellulose and lignin as part of the biomass degradation. The parameters of single conversion rate curves (pseudo-component curves) were defined by adjusting the fitting results with the global curve (summed experimental DTG curve) using the OriginPro software version OriginPro 2022b).
(10)
(10)
(11)
(11) y0 is the baseline where the bi-gaussian variable function at x0, x is the independent variable, xc is the centre value or the abscissa of the maximum reaction rate, and w1 and w2 is the left and right half peak width.
2.4 Thermodynamic analysis
From the values of kinetic parameters of the best fit model (highest R2 values), the thermodynamic properties such as a change in enthalpy (ΔH), Gibbs free energy (ΔG), and change in entropy (ΔS) were determined by equations (12), (13), and (14). Thermodynamic parameters help evaluate the heating and cooling requirements during co-pyrolysis. Several studies have determined the parameters from the kinetics of mixed feeds (W. Wang et al. Citation2022; Rasam et al. Citation2020; Naqvi et al. Citation2019).
(12)
(12)
(13)
(13)
(14)
(14)
Where Tm is the peak decomposition temperature (K), kb denotes the Boltzmann constant (1.38 × 10−23 J/K), and h represents the Planck constant (6.63 × 10−34 Js).
3. Results and discussion
The results and discussion follow the objectives (section 1) and the materials and methodology section (section 2). The characterisation of the single, binary and ternary feedstocks (section 3.1) is followed by the discussion of the thermal decomposition behaviour retrieved from the TGA and DTG curves (section 3.2). Furthermore, section 3.3, 3.4, and 3.5 discuss the results of synergistic effects; reaction mechanisms, and kinetics using Coats Redfern models; and thermodynamics using the kinetic parameters from the best fit models, respectively.
3.1 Characterisation of feedstocks
shows the proximate and ultimate analysis of the single, binary and ternary feeds. Since DP is biomass, its constituents are different from waste plastics. The proximate analysis shows the DP has higher moisture, ash, and fixed carbon content but lower volatiles than the plastics (PP and PS). Most of the plastics comprised volatiles, ∼98 and ∼99% of PP and PS, with little moisture (0.76% for PS), respectively. DP and the plastics have 80-90% volatiles when mixed in equal proportions. Also, fixed carbonand moisture of the binary biomass-plastic mixture are less than individual DP and more than PP and PS. When the plastics are mixed in equal proportions, they have the same constituents. Finally, when all three are mixed, the moisture is 1.69%, and the majority are volatiles (90.76%) with some ash (1.01%) and 5.64% of fixed carbon. The ash content is much lower than the DP value in the biomass plastic mixtures,
Table 1. The proximate and ultimate analyses of feeds.
The ultimate analysis reflected the mixed behaviour pattern (refer to ). DP had the lowest carbon content (∼45.95%) and the highest nitrogen content (∼4.27%). All the feedstocks did not contain any sulfur, and the plastics did not have any nitrogen. The carbon content increased in the order of DP > PP > PS in the range of 45.95–91.42%, and the hydrogen content increased in the order of DP > PS > PP in the range of 13.26–6.20%. The most significant difference between the biomass and plastic samples (in addition to the carbon content) was the difference in the oxygen content. While DP had ∼31% of oxygen, the oxygen content in plastics was less than 1%. When DP is mixed with the plastics, the oxygen content is around 17-18%, the carbon content is approximately 67-69%, and the nitrogen is about 2%. Binary plastic mixtures have similar constituents as single plastics in terms of carbonWhen all three feedstocks were mixed, the carbon content was around 82%, which is more than DP and less than individual plastics; however, since most of the mixture is plastics, the carbon content is high. No nitrogen was detected when all three were mixed.
3.2 Thermal decomposition behaviour of single, binary, and ternary
The thermal degradation behaviour is reflected in the TGA and DTG curves of the single feeds (a-f), binary (g-l) and ternary feeds (m-n). Table S2 shows the onset, peaks, and end temperature of the detected peaks in the DTG curves. The peak ranges reflect the various stages in the degradation process of the feed. The curves also indicate how the feeds behave during pyrolysis and co-pyrolysis in nitrogen atmosphere conditions.
Figure 1. TGA (a, c, e, g, i, k, m) and DTG (b, d, f, h, j, l, n) curves of the single, binary and ternary biomass and plastic feeds.
For the single feed, degradation occurs in a single stage. For the plastics, a linear degradation behaviour (maximum weight loss in one stage between 600 and 770 K), is reflected in an inverted S-shape which has been reported previously for single o-pyrolysis of sewage sludge and low-density polyethylene – A thermogravimetric study of thermo-kinetics and thermodynamic parameters (Burra and Gupta Citation2018; Zaker et al. Citation2021) and binary plastic mixtures (Dubdub and Al-Yaari Citation2021). The same behaviour is also seen in the binary plastic mixture in this study ( h).
Alternatively, DP behaves as a typical biomass feed as seen in other publications in three major stages (Parthasarathy et al. Citation2022; Elkhalifa et al. Citation2020; Zhou et al. Citation2018) – room temperature to 1173.15 (the studied end temperature) following dehydration (first step), lignin, cellulose, hemicellulose, protein, fat degradation (one endotherm reflects this step), and char degradation (final step). DP majorly degrades between 441.0 and 790.6 K (Stage I), with the onset temperature and end temperature increasing with increasing heating rates. Unlike plastics, the DTG curve shows small peaks between 600 K and the end temperature. However, the peaks are not distinctly separated; the degradation is assumed to occur by a one-step process. The total weight loss for the DP at the final temperature for all samples is 74-78%. On the other hand, the total weight loss for PP and PS is 100% at all heating rates. As seen in Table S2, the peak temperatures and the weight loss at the peak temperatures increase with increasing heating rates. With increasing temperatures, char yield – reflected in the weight percentage during the TGA analysis – decreases considerably for all the samples. It is observed that plastics are better suited for volatiles (gas and oil) production at higher temperatures (>700 K) rather than char due to the absence of residual solids. The TGA data show us that the potential for char production (refer Equationequation 5(5)
(5) ) usingDP even at the finaltemperatures range from ∼19-17% with increasing heating rates. The effect of the heating rate was observed in not only the residual char yield but also the maximum weight loss at peak temperatures. The exact char yield quantification from the residual solid yields in TGA should be verified against pyrolysis reactor studies (not in the scope of this study).
The effect of mixing biomass and plastic (1:1 wt.%) is observed in the TGA and DTG curves. Since biomass (DP) and plastics (PP and PS) reflect different behaviours, mixing them shows distinct behaviour. The DTG curves of the binary and ternary mixtures of biomass and plastics show two distinct peaks, reflecting two main degradation stages. Two distinct peaks were shown when cardboard and PE were mixed (Wen et al. Citation2021), microalgae and different plastics (including PP and PS) (Chen et al. Citation2021), Eucalyptus and single-use plastics (PS and LDPE) (Vanapalli et al. Citation2021), and cyanobacteria and PP (L. Li et al. Citation2021). The first peak reflects biomass degradation from 503 - 638 K, and the second peak reflects plastics (PP degradation occurring between 629 and 734 K, and PS degradation between 617 and 744 K). Additionally, it is observed that when DP is mixed with plastics in equal measure, biomass degradation starts and ends at lower temperatures compared to single-feed degradation. Alternatively, plastic degradation occurs at almost the same temperatures as single plastic degradation. Subsequently, the binary plastic mixtures show one main peak, as the single plastics. However, there seems to be an embedded small peak at around 716, 731, and 742 at 10, 20, and 30 K/min heating rates, respectively, which is absent in the individual DTG curves. Since the individual PP degradation occurred at slightly higher and wider temperature ranges, the second embedded peak could be attributed to its degradation in the binary mixture. Also, since the peak is not distinctly separate, the degradation is considered a single step, and the kinetics are calculated for one stage.
Furthermore, the embedded peak is also seen in the ternary samples, which show two district peaks like the binary biomass plastic mixtures. In essence, the plastic degradation occurring at later temperatures is confirmed when the embedded peak from the binary plastic mixtures is observed in the ternary mixtures at higher temperatures. The weight loss in all binary and ternary mixtures ranges between 90.60 - 100%. The results show that mixing biomass and plastics is excellent due to maximum product generation, especially volatiles. Char could be produced at lower temperatures, and considerable volatiles could be generated with minimum solid residues at higher temperatures. Another study discussed the hydrogenation reaction between the unsaturated products from plastics and biomass, yielding lower char production when microalgae were co-pyrolyzed with PP, PS, and PET in binary blends (Chen et al. Citation2021). Subsequently, another study states that plastic suppresses residue char formation due to polymerisation inhibition and crosslinking reactions when pyrolyzed with biomass (Wen et al. Citation2021). Hence, such mechanisms could also be the reason for the reduced char yield in our study.
Due to multiple overlapping peaks, the deconvolution procedure of asymmetrical functions is utilised for analysing the biomass degradation. In this study, the bi-gaussian function has been applied to study the thermal degradation behaviour of the biomass (DP), plastics PP and PS when mixed with binary and ternary mixtures to enhance the discussion. Table S3 shows the deconvolution parameters of the components at a heating rate of 30 K/min. shows the deconvoluted DTG curves for the studied feeds fitted with R2 values ranging from 0.988 for DP to 0.999 for DP:PP:PS. The biomass consists of three pseudo-components (hemicellulose, cellulose, and lignin). The binary and ternary biomass-plastic feeds have 4 and 5 pseudo-components since plastic constitutes the fourth and fifth components. The biomass components in DP of hemicellulose degradation occur first at around 575 K, followed by cellulose (666 K) and lignin (747 K).
Figure 2. Deconvoluted DTG curves fitting with bi-gaussian functions for single (a-c), binary (d-f) and ternary (g) feeds.

The degradation of pseudo-hemicellulose occurs at temperatures close to 575 K for the binary and ternary mixtures. The cellulose degradation occurs at slightly higher temperatures when pyrolyzed with plastics. The most variation occurs during the lignin degradation since it occurs around the same temperature as the plastics. The PP, PS degradation is at 752 and 735 K; these temperatures shift to lower temperatures for the binary mixtures (PP at 742 K and PS at 710 K). Plastics are degraded at similar temperatures in the binary and ternary biomass and plastic feed. However, the lignin degradation occurs at different expected temperatures (∼707 K), especially when mixed with PS. While the single PS degrades at 706 K, both lignin and PS degrade around 707 K during the DP:PS degradation. The degradation of PS through random scission produces free radicals, which interact with the free radicals produced from the homolytic cleavage resonance of lignin, thereby interrupting lignin radical coupling. This mechanism has also been proposed by an earlier co-pyrolysis study of lignocellulosic biomass and PS (Vanapalli et al. Citation2021). The tendency of PS to interact with the lignin portion to enhance biomass conversion to produce volatiles and reduce char formation by forming stable low molecular aromatics which aid in the synergistic effects and similar peak degradation temperatures.
3.3 Synergistic effects
A positive and passive synergistic effect in the solid-state is reflected when ΔWfinal is greater and less than zero, respectively. This study observed a positive synergy when DP is mixed with the plastics (binary and ternary mixtures) in the solid-state at lower heating rates (10 and 20 K/min) and a passive synergy at higher heating rates (30 K/min) and the final temperatures (Table S4). Alternatively, when the plastics are mixed, there is a passive synergy at all heating rates due to the absence of char yield. Furthermore, the negative values reduce heating rates, reflecting reduced passivity with increasing heating rates. The positive synergistic effect shows promise in increasing char yield when mixing biomass and plastics, especially at lower heating rates. This behaviour reflects lower volatiles generation than mixed plastic samples since positive synergy occurs in the solid state. However, the overall synergy in mixed plastic samples observed in the RMSE and correlation coefficient values shows synergy in both solid and non-solid-state conditions, hence providing benefits in mixing waste for gas and oil production. The correlation coefficient is always less than 1 and not equal to 1, and the RMSE values are always higher than zero showing the presence of synergy at all heating rates and for all studied feeds.
The interactions between the volatiles with the char from earlier biomass degradation, unreacted feedstock, and the volatiles (from biomass) all contribute to the synergy when biomass and plastics are mixed (Liu et al. Citation2020). As the proximate analysis shows, the volatiles content is significantly higher for the plastics than for the DP. However, the difference in the exact components when mixing the two different plastics requires further analysis and is not in the scope of this study. A previous study assigned the synergistic effects due to the volatiles from textile dyeing sludge interacting with the alkane groups from the polyolefin cracking when mixed with PP or PE (medical plastic wastes) (Ding et al. Citation2021). Subsequently, another study stated that the oligomer radicals from PP with the OH from cellulose (biomass) created long alcohol chains, which lowers AE and char yields (Burra and Gupta Citation2018).
This study showed differences in synergy when the biomass was mixed with the two different plastics – the RMSE values increase more when mixed with PS (rather than PP), reflecting more synergy. The ΔWfinal is also higher for DP: PS than DP:PP at 10 and 20 K/min, which shows that adding biomass to the PS causes more char yields than PP, although individual plastics produce no chars (refer to ). Adding PP and PS to DP at higher temperatures has shown better carbon conversion of biomass at 30 K/min, aiding in volatiles generation, therefore reducing char residues. Such observation was also seen when pinewood was mixed with PP and PET in binary mixtures (Burra and Gupta Citation2018) and microalgae with different plastics, including PP and PS (Chen et al. Citation2021). Furthermore, blending PS has previously exhibited synergistic and inhibitory effects when mixed with Eucalyptus biomass. While the inter-radical chemical interactions aided in synergistic effects, the inhibitory effects on the biomass due to the plastics resulted in chars with better thermal properties, increased surface areas, and lower carbon contents (Vanapalli et al. Citation2021). The inhibitory effects arose with increasing temperature after the degradation of plastic, which had condensed to form a polymer coat on the surface of the char (absent in biochars). Such effects could have occurred in our study, which is reflected in the increased char production during co-pyrolysis with respect to the zero residual weights for the individual plastic pyrolysis. In this study, with the increase in temperature, the synergistic effects of promotion and inhibition vary considerably for the different feeds. When the ΔW is positive, there is a promoting effect, while a negative value shows an inhibiting effect. Figure S1 shows the synergistic effects in the solid state of the feeds over the temperature. The synergistic effects reduce considerably as the heating rate increases for all feeds. The inhibiting effect in the binary plastics is highest at 700, 500, and 400 K as the heating rate increases from 10 to 30 K/min. The highest inhibiting effect occurred between 600 and 800 K in the case of DP:PS, which confirms the radicals interaction discussion in section 3.2. Alternatively, the promoting effects are highest in the case of DP:PS and DP:PP:PS at 700 and 500 K for 10 and 20 K/min, respectively. The complex nature of volatiles-biomass interaction with increasing heating rates requires investigation using molecular analytical tools such as TGA coupled with Fourier Transform Infrared Spectroscopy (FTIR).
Table 2. Kinetic parameters of feeds at various heating rates at the first major mass loss region (Stage I).
The decreased solid residue with increasing heating rates reflects the enhanced volatiles and carbon conversion degree of synergy reported previously in the literature(Burra and Gupta Citation2018; Jiang et al. Citation2022). As seen in Table S2, pyrolysis solid residual yields are between 19.06 - 17.25 wt.% for the single DP, reducing to as high as 11.14 (DP: PS at 10 K/min) and low 4.96 (DP: PP: PS at 30 K/min). Additionally, an apparent synergistic effect is observed at peak degradation temperatures. When the plastics are mixed in binary mixtures, the maximum weight loss rate is reduced significantly compared to individual pyrolysis degradation at all heating rates. Although the final residual weight is 0% for all samples and the weight loss rates ranges were similar for PP and PS, the weight loss heating rates vary when the plastics are mixed, proving synergistic effects during degradation (also observed in the deconvolution peak in d). Alternatively, the reduced degradation temperature ranges (seen in DTG curves) and decreased maximum weight loss rate for the first peak in the binary DP and plastic mixtures (reflecting biomass degradation) compared to the individual DP degradation reflect synergy as well.
3.4 Reaction mechanisms and kinetic parameters using coats redfern models
The Coats-Redfern model showed the reaction mechanism for the single, binary, and ternary mixtures at the three heating rates (Table S1). The major weight loss regions are considered for the kinetic parameter studies, as reported in Table S2. For single feeds and binary plastic feeds, only one stage is studied, while for biomass plastic binary and ternary mixtures, the kinetic parameters for the two distinct weight loss regions are studied (Stage I and Stage II).
The solid-state reaction mechanisms by plotting linear regression models helped calculate AE and A. (first major weight loss region) and (second major weight loss region) show the kinetic parameters – AE and A – received from all models that showed R2 values higher than 0.8. Based on the main peaks associated with the significant degradation of the samples ( b, d, f, h, j, l, n) reflected in the main stages of degradation mentioned in Table S2, the kinetic parameters were calculated from the regression models and reported. While the AE suggests the minimum energy required for the reaction to occur, the A reflects the number of times the molecules hit to trigger reactions (Zaker et al. Citation2021). Ideally, the lower the AE, the faster the reaction occurs; therefore, lower values are favoured for pyrolysis (Zaker et al. Citation2021), (Dubdub and Al-Yaari Citation2021). Additionally, a higher than and less than E + 9 s−1 show dependence and independence on the surface area of the feeds.
Table 3. Kinetic parameters of feeds at various heating rates at the second major mass loss region (Stage II).
The calculated AE for DP, PP and PS ranged between 3.87 - 46.44, 115.29 - 654.59, 25.59 - 519.08 kJ/mol, and the A factor ranged between E + 04 – E + 09, E + 04 – E + 35, E + 06 – E + 36 s−1. The range for the plastics is larger than the biomass for both the kinetic parameters. Furthermore, when the plastics are mixed, the range increases and reduces for the AE and A, respectively (30.42 - 424.66 kJ/mol and E + 06 – E + 29 s−1). Furthermore, the ranges are much lower during the two stages than the individual plastics values for binary and ternary biomass-plastic mixtures. Moreover, the AE for the Stage I, for DP: PP, DP:PS, and DP:PP:PS ranged from 2.79 - 121.49, 3.50 - 137.51, 4.98 - 105.01, and Stage II ranged between 27.18–114.20, 29.10–179.22, 31.34– 182.63 kJ/mol. It is observed that the ranges in Stage I are higher than the values in Stage II – however, the highest values are still much lower compared to the AE required for plastics degradation. Furthermore, although the range for ternary wastes is smaller than the binary biomass-plastic wastes, the lowest energy required is still higher than the binary biomass-plastic and single biomass mixtures. It is evident in other publications that the overall AE has increased when plastic is mixed with biomass (with respect to the latter). However, it depends on the type of biomass and plastic and the blending ratios (refer to ). For example, mixing more plastic with textile dyeing sludge was shown to increase the AE to values close to the values for the individual pyrolysis of plastics and much higher than the biomass (Ding et al. Citation2021).
Table 4. Average activation energy (AE) results and studied models from various publications.
Similarly, increasing PVC increased AE from 34.17–183.58 kJ/mol (Tang et al. Citation2018). Iso-conversational modeling results from pine needles and Styrofoam co-pyrolysis showed AE, higher than the former and lower than the latter. Also, the more plastic, the higher the AE (Mishra, Sahoo, and Mohanty Citation2019). It is worth noting that such behaviour has rarely been opposed; for example, when LLDPE and bamboo sawdust were mixed, the AE reduced with respect to the biomass (refer to ) (Alam et al. Citation2020), mainly attributed to the structure and arrangement of the polymer leading to higher thermal reactivity in the blends. However, our feeds follow typical biomass-plastic co-pyrolysis behaviour. Additionally, although the lowest values range from E + 03 for the biomass-plastic mixtures, the highest values are E + 09, E + 10, and E + 08 for Stage I for DP:PP, DP:PS, and DP:PP:PS, respectively, and E + 11 for Stage II for all feeds. The A values show lower ranges (similar to AE ranges) when the feeds are co-pyrolyzed relative to individual plastic samples.
The highest R2 values for the samples were amongst the diffusion models (D1, D2, and D4), Mampel power law (P2/3), first-order reaction (F1), and the nucleation models (A2, A3, and A4) had the highest R2 values. The diffusion model – Ginstlinge Brounshtein (D4) was the most common fit reaction mechanism, including Stage I for DP, DP: PP, DP: PS, DP: PP: PS, and the DP: PS for Stage II, for all heating rates. Using the Coats Redfern models, another study has reported a similar reaction mechanism for date palm fibres kinetics (Raza et al. Citation2022). Additionally, plastics (both single and binary) followed one of the following mechanisms: P2/3 and D2, D1, F1, A2, A3, A4 – the last four being the highest R2 for both the 10 and 20 K/min for PP:PS degradation and 30 K/min for PS. The majority of the Stage II mechanisms followed the Power Law (P2/3), with the exceptions of DP: PP – 10, DP: PP – 20, and DP: PS 10 K/min with D1, D3, and D3, respectively.
For the single feeds, with a single main degradation step, the AE for the biomass is significantly lower than the plastics, which was also seen in other biomass-plastic studies (Chen et al. Citation2021). The biomass energy for different heating rates ranges from 15.99-16.85 kJ/mol, while the PP and PS energies range from 256–533 and 205.32-519.08 kJ/mol. The highest AE arose when the reaction mechanism follows the power-law – P2/3. Other studies have reported AE of 249.7 kJ/mol for LDPE; 312 for PS, 337 for PP, 341 for LDPE, and 445 for HDPE (H. Li et al. Citation2005); 151.5 for PVC, 226.8 for PET, and 210.8 for PS (Özsin, Pütün, and Pütün Citation2019); 295.65 for PP and 254.55 for LDPE (Uzun and Yaman Citation2017) degradation using various kinetic models, and owing to the thermal stability of plastic samples.
Additionally, the AE is lower, especially at lower heating rates, for the binary plastics than the single plastics, which showed an easier degradation pathway when mixing feeds. The lower values are due to differences in reaction mechanisms, with the highest R2 values, in single and binary feeds. Subsequently, the two separate stages of degradation for DP: PP, DP: PS, and DP: PP: PS yields separate kinetic parameters. In Stage I, the reaction mechanisms for every heating rate follow D4, which reflects biomass degradation at lower temperatures, followed by plastics in Stage II. The values are slightly lower for DP: PP (average – 10.98 kJ/mol) than DP: PS (11.30 kJ/mol); however, both are lower than the single DP AE. Therefore, confirming quicker reaction mechanisms when mixed with plastics. Furthermore, the ternary mixtures follow the D4 reaction mechanism in Stage II – but the AE is lower than in the binary mixtures (average – 10.31 kJ/mol).
Compared to Stage I, Stage II has much higher AE, which confirms plastic degradation occurring at higher temperatures. However, the values are much lower than the individual plastic feeds. Like Stage II, the AE is lower for DP: PP than DP:PS, averaging 87.46 and 110.9 kJ/mol. Alternatively, the ternary mixtures have higher values than the binary mixtures (average – 167.9 kJ/mol). The higher values in Stage II of ternary samples further reflect the two-plastic degradation at higher temperatures. Additionally, the binary plastic mixtures PP: PS averaged 216.4 kJ/mol.
The A of the single, binary and ternary mixtures behave similarly to AE. Moreover, for PS-30, PP: PS-10 and 20 – with multiple highest R2 reaction mechanisms – the A 1.95E + 13, 7.09E + 10, and 1.65E + 11 s1, respectively. Furthermore, the biomass feed (at E + 09 s−1) reflects lower values for single plastic feeds (E + 12 – E + 36 s−1). The binary plastic mixtures are much lower than the single plastic feeds, like the AE values (E + 10 to E + 18 s−1). For Stage, I, for the binary biomass and plastic mixtures, the A for heating rates are at E + 09 s−1.
Furthermore, the values become lower to E + 08 s−1 for ternary mixtures. Subsequently, for Stage II, the A varies with different heating rates. DP: PP ranges from E + 03 to E + 05 s−1, and DP: PS from E + 06 to E + E11 s−1. Finally, the values range from E + 04 to E + 09 s−1 for the ternary mixtures. Additionally, values higher than and less than E + 9 s−1 show dependence and independence on the surface area of the feeds. It is clear from the results that biomass degradation does not rely on surface area, but the plastics are otherwise when pyrolyzed individually. Furthermore, the reliance on the surface is completely reduced since all the values are equal to or less than E + 9 in binary and ternary mixtures (at both stages).
3.5 Thermodynamic parameters
The AE and A values of the best fit models were employed to calculate the thermodynamic parameters from equations 12-14. shows the change in enthalpy (ΔH), Gibbs free energy (ΔG), and change in entropy (ΔS) values. Since ΔH is positive for all samples, it shows that energy should be provided outside to help the reaction occur. Positive values of ΔH and ΔG reflect endothermic and non-spontaneous reactions for all samples and all studied reaction mechanisms.
Table 5. Thermodynamic parameters of single, binary and ternary biomass and plastic equi-weight blends at various heating rates.
Furthermore, the ΔG values ranged from 51.46–52.78 kJ/mol for the biomass (DP), and 203.64–224.14 for the plastics at different heating rates. The ΔG values of the binary plastic mixtures are lower than all heating rates of PP and the lesser heating rates of PS. As previously discussed in the the plastic degradation stage, the Stage II values of the biomass and plastics mixtures show values of ΔH and ΔG greater than 59.93 and 187.6 kJ/mol, respectively. Furthermore, the various heating rates’ Stage I ΔH, and ΔG values for the biomass plastic mixtures range from 5.55–6.79 kJ/mol and 53.54–55.74 kJ/mol, respectively.
Also, the negative values of ΔS reflect the disorder of products less than the initial reactants. The results show that it is negative for most single, binary, and ternary feeds. However, for the different heating rates of PP and the 30 K/min for PS and PP:PS feeds, the ΔS values were positive. Positive ΔG, ΔH, and negative ΔS have previously been reported in date palm fibres (Raza et al. Citation2022), vegetable wastes (Elkhalifa et al. Citation2022), and high ash sewage sludge (Naqvi et al. Citation2019). Alternatively, biomass-plastic (Zaker et al. Citation2021) and biomass-biomass mixtures (Parthasarathy et al. Citation2022) have shown positive ΔG and H values, but both negative and positive ΔS values due the complicated nature products generation, which needs to be studied using advanced molecular techniques (not in the scope of this study).
4. Conclusion
Co-pyrolysis blends of biomass and plastics are increasingly being studied to improve efficient waste management and value-added product generation. This study observed a positive synergy when DP is mixed with the plastics (PP and PS) in the solid-state at lower heating rates (10 and 20 K/min) and a passive synergy at higher heating rates (30 K/min). Alternatively, when the plastics are mixed, there is a passive synergy at all heating rates due to the absence of any char yield. Additionally, the diffusion model Ginstlinge Brounshtein (D4) was the most common best fit reaction mechanism for Stage I for DP, DP: PP, DP: PS, DP: PP: PS, and the DP: PS for Stage II, for all heating rates, followed D4 reaction mechanism. In Stage I, the activation energy (AE) is slightly lower for DP: PP (average – 10.98 kJ/mol) than DP: PS (11.30 kJ/mol); however, both are lower than single DP with an AE (average 16.52 kJ/mol). Therefore, confirming quicker reaction mechanisms and degradation of DP when mixed with plastics.
Furthermore, Stage II of the mixtures’ degradation aligns with the degradation of the plastic in both the binary and ternary samples due to a relatively higher AE than Stage II and DP. Like Stage I, the AE is lower for DP: PP than DP:PS, averaging 87.46 and 110.93 kJ/mol compared to individual plastics. Additionally, the binary plastic mixtures PP: PS averaged 216.43 kJ/mol. Alternatively, the ternary mixtures have higher values than the binary mixtures (average – 167.93 kJ/mol). The higher values in Stage II of ternary samples further reflect the two-plastic degradation at higher temperatures.
The pre-exponential factors (A) of the single, binary and ternary mixtures behave similarly to AE. It is clear from the results that biomass degradation does not rely on surface area, but the plastics are otherwise when pyrolyzed individually. Furthermore, the reliance on the surface is reduced significantly since all the values are equal to or less than E + 9 in binary and ternary mixtures (at both stages). Positive values of ΔH and ΔG reflect endothermic and non-spontaneous reactions under all conditions and samples. Furthermore, the ΔS was negative for the DP and plastic mixtures, reflecting disorder due to bonds breaking is lower than the initial reactants. However, for the three heating rates of PP and the 30 K/min for PS and PP:PS feeds, the ΔS values were positive, showing varying disorders amongst the studied feeds. Future studies should utilise the current study to upscale the co-pyrolysis of DP and SUPs and analyze the generation of energy generation products (oil, gas and oil). Additionally, mixing biomass is desirable due to the decreased energy requirement for biomass-plastics binary and ternary mixtures. However, future studies should employ model-free kinetics to validate the model-based kinetic and thermodynamic values from this study.
Acknowledgements
Open Access funding provided by the Qatar National Library.
Declaration of statement
The authors report there are no competing interests to declare.
Acknowledgment
This publication was made possible by NPRP – Standard (NPRP-S) 11 cycle grant – NPRP11S-0117-180328 from the Qatar National Research Fund (a member of Qatar Foundation). The findings herein reflect the work and are solely the responsibility of the authors.
Disclosure statement
No potential conflict of interest was reported by the author(s).
Data availability statement
All data used in this study are available upon request.
Additional information
Funding
References
- Alam, Mahboob, Anjireddy Bhavanam, Ashirbad Jana, Jaimin kumar S. Viroja, and Nageswara Rao Peela. 2020. “Co-Pyrolysis of Bamboo Sawdust and Plastic: Synergistic Effects and Kinetics.” Renewable Energy 149: 1133–1145. doi:10.1016/j.renene.2019.10.103.
- Burra, K. G., and A. K. Gupta. 2018. “Kinetics of Synergistic Effects in Co-Pyrolysis of Biomass with Plastic Wastes.” Applied Energy 220 (February): 408–418. doi:10.1016/j.apenergy.2018.03.117.
- Chen, Yuan, Abhishek Kumar Awasthi, Fan Wei, Quanyin Tan, and Jinhui Li. 2021a. “Single-Use Plastics: Production, Usage, Disposal, and Adverse Impacts.” Science of the Total Environment 752: 141772. doi:10.1016/j.scitotenv.2020.141772.
- Chen, Rongjie, Shiyu Zhang, Xiaoxiao Yang, Guanghao Li, Hui Zhou, Qinghai Li, and Yanguo Zhang. 2021b. “Thermal Behaviour and Kinetic Study of Co-Pyrolysis of Microalgae with Different Plastics.” Waste Management 126: 331–339. doi:10.1016/j.wasman.2021.03.001.
- Coats, A. W., and J. P. Redfern. 1964. Kinetic Parameters from Thermogravimetric Data [12].” Nature. Nature Publishing Group. doi:10.1038/201068a0.
- Das, Subhasish, S. H. Lee, Pawan Kumar, Ki Hyun Kim, Sang Soo Lee, and Satya Sundar Bhattacharya. 2019. “Solid Waste Management: Scope and the Challenge of Sustainability.” Journal of Cleaner Production 228: 658–678. doi:10.1016/j.jclepro.2019.04.323.
- Dhyani, Vaibhav, and Thallada Bhaskar. 2018. Kinetic Analysis of Biomass Pyrolysis. Waste Biorefinery: Potential and Perspectives. Elsevier B.V. doi:10.1016/B978-0-444-63992-9.00002-1.
- Ding, Ziyi, Jingyong Liu, Huashan Chen, Shengzheng Huang, Fatih Evrendilek, Yao He, and Li Zheng. 2021. “Co-Pyrolysis Performances, Synergistic Mechanisms, and Products of Textile Dyeing Sludge and Medical Plastic Wastes.” Science of the Total Environment 799: 149397. doi:10.1016/j.scitotenv.2021.149397.
- Dubdub, Ibrahim, and Mohammed Al-Yaari. 2020. “Pyrolysis of Mixed Plastic Waste: I. Kinetic Study.” Materials 13 (21): 4912–4915. doi:10.3390/ma13214912.
- Dubdub, Ibrahim, and Mohammed Al-Yaari. 2021. “Thermal Behavior of Mixed Plastics at Different Heating Rates: I. Pyrolysis Kinetics.” Polymers 13 (19), doi:10.3390/polym13193413.
- Elkhalifa, Samar, Omar Elhassan, Prakash Parthasarathy, Hamish Mackey, Tareq Al-Ansari, and Gordon McKay. 2020. “Thermogravimetric Analysis of Individual Food Waste Items and Their Blends for Biochar Production.” In 30 European Symposium on Computer Aided Process Engineering, edited by Sauro Pierucci, Flavio Manenti, Giulia Luisa Bozzano, and Davide B T - Computer Aided Chemical Engineering Manca, 48:1543–1548. Elsevier. doi:10.1016/B978-0-12-823377-1.50258-5.
- Elkhalifa, Samar, Prakash Parthasarathy, Hamish R. Mackey, Tareq Al-Ansari, Omar Elhassan, Said Mansour, and Gordon McKay. 2022. “Biochar Development from Thermal TGA Studies of Individual Food Waste Vegetables and Their Blended Systems.” Biomass Conversion and Biorefinery 0123456789), doi:10.1007/s13399-022-02441-0.
- Fermanelli, Carla S, Agostina Córdoba, Liliana B. Pierella, and Clara Saux. 2020. “Pyrolysis and copyrolysis of three lignocellulosic biomass residues from the agro-food industry: A comparative study.” Waste Management 102: 362–370. http://dx.doi.org/10.1016/j.wasman.2019.10.057.
- Foong, Shin Ying, Rock Keey Liew, Yafeng Yang, Yoke Wang Cheng, Peter Nai Yuh Yek, Wan Adibah Wan Mahari, Xie Yi Lee, et al. 2020. “Valorization of Biomass Waste to Engineered Activated Biochar by Microwave Pyrolysis: Progress, Challenges, and Future Directions.” Chemical Engineering Journal 389 (February): 124401. doi:10.1016/j.cej.2020.124401.
- Hassan, H., B. H. Hameed, and J. K. Lim. 2020. “Co-pyrolysis of Sugarcane Bagasse and Waste High-Density Polyethylene: Synergistic Effect and Product Distributions.” Energy 191: 116545. doi:10.1016/j.energy.2019.116545.
- Jiang, Liyang, Zhen Zhou, Huan Xiang, Yang Yang, Hong Tian, and Jiawei Wang. 2022. “Characteristics and Synergistic Effects of Co-Pyrolysis of Microalgae with Polypropylene.” Fuel 314 (November 2021): 122765. doi:10.1016/j.fuel.2021.122765.
- Li, Lixin, Jie Wang, Chao Jia, Ying Lv, and Yan Liu. 2021. “Co-Pyrolysis of Cyanobacteria and Plastics to Synthesize Porous Carbon and Its Application in Methylene Blue Adsorption.” Journal of Water Process Engineering 39 (May 2020): 101753. doi:10.1016/j.jwpe.2020.101753.
- Li, Haiying, Shuting Zhang, Xinhua Zhao, and Suzuki Eiji. 2005. “Pyrolysis Characteristics and Kinetics of Municipal Solid Waste.” Transactions of Tianjin University 11 (5): 353–359.
- Liew, Jia Xin, Adrian Chun Minh Loy, Bridgid Lai Fui Chin, Ahmed AlNouss, Muhammad Shahbaz, Tareq Al-Ansari, Rajesh Govindan, and Yee Ho Chai. 2021. “Synergistic Effects of Catalytic Co-Pyrolysis of Corn Cob and HDPE Waste Mixtures Using Weight Average Global Process Model.” Renewable Energy 170: 948–963. doi:10.1016/j.renene.2021.02.053.
- Liu, Xuan, Kiran G. Burra, Zhiwei Wang, Jinhu Li, Defu Che, and Ashwani K. Gupta. 2020. “On Deconvolution for Understanding Synergistic Effects in Co-Pyrolysis of Pinewood and Polypropylene.” Applied Energy 279 (x): 115811. doi:10.1016/j.apenergy.2020.115811.
- Mariyam, Sabah, Muhammad Shahbaz, Tareq Al-Ansari, Hamish R. Mackey, and Gordon McKay. 2022. “A Critical Review on Co-Gasification and Co-Pyrolysis for Gas Production.” Renewable and Sustainable Energy Reviews 161 (March): 112349. doi:10.1016/j.rser.2022.112349.
- Mishra, Ranjeet Kumar, Abhisek Sahoo, and Kaustubha Mohanty. 2019. “Pyrolysis Kinetics and Synergistic Effect in Co-Pyrolysis of Samanea Saman Seeds and Polyethylene Terephthalate Using Thermogravimetric Analyser.” Bioresource Technology 289 (June): 121608. doi:10.1016/j.biortech.2019.121608.
- Naqvi, Salman Raza, Rumaisa Tariq, Zeeshan Hameed, Imtiaz Ali, Muhammad Naqvi, Wei Hsin Chen, Selim Ceylan, et al. 2019. “Pyrolysis of High Ash Sewage Sludge: Kinetics and Thermodynamic Analysis Using Coats-Redfern Method.” Renewable Energy 131: 854–860. doi:10.1016/j.renene.2018.07.094.
- Özsin, Gamzenur, Ayşe Eren Pütün, and Ersan Pütün. 2019. “Investigating the Interactions Between Lignocellulosic Biomass and Synthetic Polymers During Co-Pyrolysis by Simultaneous Thermal and Spectroscopic Methods.” Biomass Conversion and Biorefinery 9 (3): 593–608. doi:10.1007/s13399-019-00390-9.
- Papari, Sadegh, Hanieh Bamdad, and Franco Berruti. 2021. “Pyrolytic Conversion of Plastic Waste to Value-Added Products and Fuels: A Review.” Materials 14 (10), doi:10.3390/ma14102586.
- Parthasarathy, Prakash, Tareq Al-Ansari, Hamish R. Mackey, K. Sheeba Narayanan, and Gordon McKay. 2022a. “A Review on Prominent Animal and Municipal Wastes as Potential Feedstocks for Solar Pyrolysis for Biochar Production.” Fuel 316 (January): 123378. doi:10.1016/j.fuel.2022.123378.
- Parthasarathy, Prakash, Mohammad Alherbawi, Snigdhendubala Pradhan, Tareq Al-Ansari, Hamish R. Mackey, and Gordon McKay. 2022b. “Pyrolysis Characteristics, Kinetic, and Thermodynamic Analysis of Camel Dung, Date Stone, and Their Blend Using Thermogravimetric Analysis.” Biomass Conversion and Biorefinery 0123456789), doi:10.1007/s13399-021-02249-4.
- Rasam, Sajjad, Ali Moshfegh Haghighi, Kolsoom Azizi, Antonio Soria-Verdugo, and Mostafa Keshavarz Moraveji. 2020. “Thermal Behavior, Thermodynamics and Kinetics of Co-Pyrolysis of Binary and Ternary Mixtures of Biomass Through Thermogravimetric Analysis.” Fuel 280 (July): 118665. doi:10.1016/j.fuel.2020.118665.
- Raza, Mohsin, Basim Abu-Jdayil, Ali H. Al-Marzouqi, and Abrar Inayat. 2022. “Kinetic and Thermodynamic Analyses of Date Palm Surface Fibers Pyrolysis Using Coats-Redfern Method.” Renewable Energy 183: 67–77. doi:10.1016/j.renene.2021.10.065.
- Ro, Kyoung S, Patrick G. Hunt, Michael A Jackson, David L. Compton, Scott R Yates, Keri Cantrell, and SeChin Chang. 2014. “Co-pyrolysis of swine manure with agricultural plastic waste: Laboratory-scale study.” Waste Management 34 (8): 1520–1528. http://dx.doi.org/10.1016/j.wasman.2014.04.001.
- Singh, Sanjay, Trilok Patil, Shyam P. Tekade, Manoj B. Gawande, and Ashish N. Sawarkar. 2021. “Studies on Individual Pyrolysis and Co-Pyrolysis of Corn Cob and Polyethylene: Thermal Degradation Behavior, Possible Synergism, Kinetics, and Thermodynamic Analysis.” Science of the Total Environment 783 (April): 147004. doi:10.1016/j.scitotenv.2021.147004.
- Tahir, Aola H.F., Abdul Hameed M.J. Al-Obaidy, and Faris H. Mohammed. 2020. “Biochar from Date Palm Waste, Production, Characteristics and Use in the Treatment of Pollutants: A Review.” IOP Conference Series: Materials Science and Engineering 737 (1), doi:10.1088/1757-899X/737/1/012171.
- Tang, Yijing, Qunxing Huang, Kai Sun, Yong Chi, and Jianhua Yan. 2018. “Co-Pyrolysis Characteristics and Kinetic Analysis of Organic Food Waste and Plastic.” Bioresource Technology 249 (August 2017): 16–23. doi:10.1016/j.biortech.2017.09.210.
- Uzun, Başak Burcu, and Elif Yaman. 2017. “Pyrolysis Kinetics of Walnut Shell and Waste Polyolefins Using Thermogravimetric Analysis.” Journal of the Energy Institute 90 (6): 825–837. doi:10.1016/j.joei.2016.09.001.
- Vanapalli, Kumar Raja, Jayanta Bhattacharya, Biswajit Samal, Subhash Chandra, Isha Medha, and Brajesh K. Dubey. 2021. “Inhibitory and Synergistic Effects on Thermal Behaviour and Char Characteristics During the Co-Pyrolysis of Biomass and Single-Use Plastics.” Energy 235: 121369. doi:10.1016/j.energy.2021.121369.
- Varma, Anil Kumar, Navneeta Lal, Ashwani Kumar Rathore, Rajesh Katiyar, Lokendra Singh Thakur, Ravi Shankar, and Prasenjit Mondal. 2021. “Thermal, Kinetic and Thermodynamic Study for Co-Pyrolysis of Pine Needles and Styrofoam Using Thermogravimetric Analysis.” Energy 218: 119404. doi:10.1016/j.energy.2020.119404.
- Varsha, S. S. V., Arun K. Vuppaladadiyam, Farrukh Shehzad, Hosein Ghaedi, S. Murugavelh, Weiguo Dong, and Elsa Antunes. 2021. “Co-Pyrolysis of Microalgae and Municipal Solid Waste: A Thermogravimetric Study to Discern Synergy During Co-Pyrolysis Process.” Journal of the Energy Institute 94: 29–38. doi:10.1016/j.joei.2020.10.010.
- Wang, Weimin, Guanqun Luo, Yuan Zhao, Yuanjun Tang, Kaige Wang, Xuan Li, and Yousheng Xu. 2022. “Kinetic and Thermodynamic Analyses of Co-Pyrolysis of Pine Wood and Polyethylene Plastic Based on Fraser-Suzuki Deconvolution Procedure.” Fuel 322 (March): 124200. doi:10.1016/j.fuel.2022.124200.
- Wang, Xuebin, Daoyang Ma, Qiming Jin, Shuanghui Deng, Hrvoje Stančin, Houzhang Tan, and Hrvoje Mikulčić. 2019. “Synergistic Effects of Biomass and Polyurethane Co-Pyrolysis on the Yield, Reactivity, and Heating Value of Biochar at High Temperatures.” Fuel Processing Technology 194 (May), doi:10.1016/j.fuproc.2019.106127.
- Wen, Yuming, Ilman Nuran Zaini, Shule Wang, Wangzhong Mu, Pär Göran Jönsson, and Weihong Yang. 2021. “Synergistic Effect of the Co-Pyrolysis of Cardboard and Polyethylene: A Kinetic and Thermodynamic Study.” Energy 229), doi:10.1016/j.energy.2021.120693.
- Yuan, Haoran, Chengyu Li, Rui Shan, Jun Zhang, Yufeng Wu, and Yong Chen. 2022. “Recent Developments on the Zeolites Catalyzed Polyolefin Plastics Pyrolysis.” Fuel Processing Technology 238 (June): 107531. doi:10.1016/j.fuproc.2022.107531.
- Zaker, Ali, Zhi Chen, Mohammed Zaheer-Uddin, and Jianbo Guo. 2021. “Co-Pyrolysis of Sewage Sludge and Low-Density Polyethylene – A Thermogravimetric Study of Thermo-Kinetics and Thermodynamic Parameters.” Journal of Environmental Chemical Engineering 9 (1): 104554. doi:10.1016/j.jece.2020.104554.
- Zhang, Wenlong, Juan Zhang, Yanming Ding, Qize He, Kaihua Lu, and Haiyan Chen. 2021. “Pyrolysis Kinetics and Reaction Mechanism of Expandable Polystyrene by Multiple Kinetics Methods.” Journal of Cleaner Production 285: 125042. doi:10.1016/j.jclepro.2020.125042.
- Zheng, Yunwu, Lei Tao, Xiaoqing Yang, Yuanbo Huang, Can Liu, and Zhifeng Zheng. 2018. “Study of the Thermal Behavior, Kinetics, and Product Characterization of Biomass and Low-Density Polyethylene Co-Pyrolysis by Thermogravimetric Analysis and Pyrolysis-GC/MS.” Journal of Analytical and Applied Pyrolysis 133: 185–197. doi:10.1016/j.jaap.2018.04.001.
- Zhou, Simiao, Lujia Han, Guangqun Huang, Zengling Yang, and Jizhen Peng. 2018. “Pyrolysis Characteristics and Gaseous Product Release Properties of Different Livestock and Poultry Manures: Comparative Study Regarding Influence of Inherent Alkali Metals.” Journal of Analytical and Applied Pyrolysis 134 (June): 343–350. doi:10.1016/j.jaap.2018.06.024.