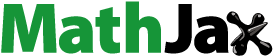
ABSTRACT
Photon extraction is one of the major issues limiting outcoupling efficiency in perovskite light-emitting diodes (PeLEDs). Light outcoupling in PeLEDs is typically limited by the disparity in refractive indices among the consisting layers and the difficulty of producing directed emitters in the emitting layers. Here, anisotropic nanorod-based perovskite with colloidal cesium lead bromide was introduced to address the issue via the oil–water interface chemical stripping transition method. It was confirmed that the horizontal transition dipole moments of 73% and refractive index value of 1.86 of the ANRs-based perovskites enhance outcoupling efficiency in PeLEDs. Finally, we achieved green-emitting ANR-PeLEDs with a current efficiency of 45.9 cd/A, power efficiency of 40.0 lm/W, and external quantum efficiency of 18.4% (turn-on voltage 3.3 V), with Commission Internationale de l'Eclairage (CIE) coordinates of (0.073, 0.71). These findings suggest further research into the anisotropic 1D shape of perovskite, which could improve the optoelectrical performance of PeLEDs.
1. Introduction
Metal halide perovskites (MHPs) with outstanding color purity, tailorable chromaticity, near-unity photoluminescence quantum yield (PLQY), and facile chemical processability have been proposed as a novel light source for next-generation flat-panel displays [Citation1–6]. MHPs have especially been attracted due to their narrow full width at a maximum (FWHM) of less than 20 nm, which can cover BT.2020 color space, reproducing the natural colors beyond the present light sources like organic LEDs (OLEDs) and quantum LEDs [Citation7–9]. However, perovskite light-emitting diodes (PeLEDs) face a practical barrier to being dominant in display and lighting applications beyond the present mainstream OLEDs due to their intrinsic high optical constant of the perovskite emitter consisting of heavy metal atoms and crystal structure [Citation10–13]. The structure of PeLEDs is typically formed by replacing the emitter with perovskite compared to OLEDs except for the transporting layers. This modification would make the refractive index contrast high between the perovskite and transport layer, resulting in low light extraction efficiency of PeLEDs by trapping light inside the device, which is over 80% of the total generated light in the emitting layer. Indeed, the external quantum efficiency (EQE) of PeLEDs has recently improved and exceeded 20% [Citation12], attributed to interfacial engineering [Citation14], ligand engineering [Citation15,Citation16], cation/anion exchange, and surface passivation [Citation17]. However, the refractive mismatching among the layers in PeLEDs still makes the efficiency gap between PeLEDs and OLEDs almost double under the assumption that without using any outcoupling enhancement structures, although the internal quantum efficiency of PeLEDs reaches near unity like OLEDs. Moreover, in OLEDs’ configuration, the horizontally oriented transition dipole moments (TDMs) would outcouple more light within the critical angle for the glass-air interface [Citation18,Citation19]. The isotropically or vertically oriented TDMs in perovskite emitters at the device level are linked with trapping and stacked through the dissipating pathways of different optical modes of generated radiation, and horizontal TDM is anticipated to further improve the upper limit of external quantum efficiency. Therefore, enhanced outcoupling is one of the essential factors in extracting the outstanding performance of PeLEDs.
Introducing anisotropic-shaped perovskite emitters in PeLEDs is a potent way to explore and improve light outcoupling [Citation20]. Compared with cubic perovskite nanocrystals (NCs), the optical TDMs of low-dimensional structures, such as nanorods and nanoplatelets, are in-plane preferentially aligned to the substrate surface [Citation21–24], which enables them to stand out in improving outcoupling in PeLEDs [Citation25]. Notably, the enhanced optical outcoupling directly related to EQE of PeLEDs could be mediated by employing an anisotropic emitting layer (EML) in preferentially in-plane–oriented TDMs, without any light-outcoupling management configuration such as matching index grading, hollow fiber, micro lens, photonic crystals, and pattern nanostructure [Citation13,Citation26–29]. There have been few reports on the effects of high horizontal TDM in quasi-two-dimensional (Qi-2D) nanoplatelets. For example, Sargent group quantified the in-plane orientation of TDMs for a suite of layered perovskites. It demonstrated that the butylammonium cations single crystal is highly anisotropic at about 80% of in-plane orientation compared to phenethylammonium cations Qi-2D perovskite [Citation30]. In addition, the numerical finite-difference time-domain calculations revealed that 30-50% efficiency values comparable to OLEDs could be obtained from in-plane TDMs with anisotropic emission. C.-J. Shih group reported that a scalable 2D superlattice system comprised of anisotropic nanocrystals maintained a horizontal dipole of up to 71% and enhanced the theoretical light outcoupling efficiency of over 30%. The PeLED shows 24% efficiency [Citation12]. Additionally, Y. Jin group represented an effective method of exploiting a high TDMs ratio of 84% and photoluminescent quantum yield (PLQY) of 75% in 2D nanoplatelet, resulting in an outcoupling efficiency of 31% [Citation22,Citation31,Citation32].
Notably, high horizontal TDM has been dominantly explored in 2D or quasi-2D perovskites even though one-dimensional nanorods have much potential to get a high anisotropy, and light extraction would be outstanding from the nanorods. More importantly, the prevailing influence of the quantum-confined Stark effect in layered perovskites skews the quantum/potential well, subjected to the applied external electric field, contrary to bulk materials, where the Franz-Keldysh effect gives a broadening of the band-edge primarily [Citation33,Citation34]. This (Stark) broadening triggers the shift of energy level of the hole and electron, decreasing the gap between these levels. These changes are accompanied by a net decrease in exciton binding energy due to reducing the Coulombic interaction as the electron and hole become spatially separated [Citation35]. Unfavorably, the bulky organic cation on the surface of layered 2D perovskites impedes the efficient charge transfer. Thus, owing to their low non-radiative recombination rates, larger photon absorption coefficient, higher charge conductivity, and controlled aspect ratio in anisotropic 1D geometry, obtaining high-performance PeLEDs with enhanced photon extraction, are an exciting avenue. Although earlier studies have witnessed research endeavors in disclosing the high horizontal TDM ratio with perovskite cubic nanocrystals [Citation22] and 2D nanoplatelets [Citation30], the relevant studies of 1D nanorods counterparts still need to be completed.
Herein, colloidal CsPbBr3 anisotropic nanorods (ANRs) were synthesized following the hot-injection method of initially non-luminescent nanopolyhedrons (NPDs) Cs4PbBr6 nanocrystals (NCs), and then oil–water chemical transformation into highly-luminescent CsPbBr3 nanorods. The reaction between the NPDs NCs in cyclohexane solution and water interface provides a semi-porous membrane that acts as the space for lead cations to insert into NPDs NCs. Owing to the anisotropic growth of 1D geometry, the ANRs exhibited a horizontal TDM of 73%. ANR-LEDs showed a turn-on-voltage at 3.3 V and maximum current efficiency (CE) of 45.9 cd/A, corresponding to an EQE of 18.4%. Further advancements in ANR-LEDs will be prompted by the improved horizontal to vertical TDMs ratio and low optical constant in 1D-ANRs.
2. Experimental section
Lead Bromide (PbBr2, 98%), Cesium carbonate (Cs2CO3, 99.9%), oleic acid (OA, 90%), 1-octadecene (ODE, 90%), n-hexane, oleylamine (OLM, 70%), and ethyl acetate (EA, anhydrous 99.8%) were purchased from Sigma-Aldrich. Poly(ethylene dioxythiophene): polystyrene sulfonate (PEDOT:PSS, AI 4083) was purchased from Ossila. Poly[N,N′-bis(4-butylphenyl)-N,N′-bis(phenyl)-benzidine] (poly-TPD) was purchased from American Dye Source, Inc. All chemicals were used as received without additional purification.
2.1 Anisotropic colloidal nanorods synthesis and purification method
The anisotropic CsPbBr3 nanorods are synthesized by the hot-injection method with the assistance of surface ligand modification of non-luminescent Cs4PbBr6 NPDs at the oil–water interface [Citation36]. The stoichiometric amount of Cs-oleate was injected into a 100 mL three-neck flask containing 0.2 mmol PbBr2, 10 mL ODE, 1 mL OA, and 1 mL OLM at 140°C. The reaction is terminated with ice water after 10 s. The mixture was centrifuged at 8000 rpm for 5 min to separate the NPDs Cs4PbBr6 NCs, and the precipitate was redispersed in 10 mL cyclohexane for an oil–water reaction.
2.1.1 Oil–Water transformation reaction
To prepare CsPbBr3 nanorods, 2 mL of Cs4PbBr6 NCs is contained inside a 2 mL water bottle for an oil–water transformation reaction. Subsequently, the top greenish solution was decanted, and 0.25 mL of ethyl acetate was added. The product was immediately centrifuged at 10,000 rpm for 5 min, and CsPbBr3 and the nanorods were isolated from the supernatant and redispersed in 2 mL hexane. This centrifuging process was repeated twice. Eventually, the nanorods were redispersed into 1 mL hexane and centrifuged at 5000 rpm for 3 min to make a high-quality product. Note that the amount of ethyl acetate used for washing is the maximum that nanorods can tolerate, whereas larger amounts could quench perovskite nanorods or rapid phase transformation. Notably, owing to the low density of native ligands, the stability of Cs4PbBr6 becomes lower, resulting in the formation of nanorods through a self-organized process. It is assumed that nanorods can sustain minimum energy status in this process. Thus, the van der Waals force and a relatively strong dipole–dipole attraction could drive self-organization. UV-Vis-IR absorption spectrum of assembled nanorods film was tested using a PerkinElmer LAMBDA-900 spectrophotometer. PLQY was measured by Quantaurus-QY Absolute PL quantum yield spectrometer (Hamamatsu, C11347-11). The PLQY measurement was initiated after washing the ANRs twice with ethyl acetate. The structure of the nanorod crystal was analyzed by an X-ray diffractometer (X'Pert-PRO MRD, Phillips). The nanorods’ shape was confirmed using a field-emission transmission electron microscope (FE-TEM, JEM-2100F), and the cross-sectional TEM images of the ANRs-LEDs were obtained from a Cs-corrected TEM (JEM-ARM 200F, JEOL) installed in the Center for University-wide Research Facilities (CURF) at Jeonbuk National University. The chemical analysis of nanorods was performed by X-ray Photoelectron Spectrometer (XPS). The spin-coated nanorods film on precleaned Si Substrate was utilized for the atomic force microscope (AFM, Nanoscope Multimode IV a Bruker). The refractive indices of the different layers were measured by variable angle spectroscopic ellipsometry (VASE, ESM-300, J.A. Woollam Co.). The n and k values for nanorods film on a silicon substrate (thickness: 55 nm∼58.3 nm) were measured using a spectroscopic ellipsometer in the reflection measurement mode with the incident angle set at 65o. The angle-dependent PL spectra of the perovskite films (deposited on quartz substrate) were collected using a molecular orientation characteristic measurement system SM245 (Spectraproducts, Korea). The range of angles is 0° to 90°. The excitation wavelength is 325 nm.
2.2 LED device fabrication
Indium tin oxide (ITO) patterned glass substrates were consecutively sonicated in acetone and isopropyl alcohol. Then, they were dried using a nitrogen gun and placed in an oven at 60 °C to remove residual solvents for 15 min. Before device fabrication, the cleaned substrates were treated using a plasma cleaner. PEDOT: PSS solutions (P VP Al 4083, filtered through a 0.45 µm PVDF filter) were spin-coated onto the ITO-coated glass substrates at 4,000 rpm for 1 min and baked at 140°C for 30 min. The PEDOT: PSS-coated substrates were transferred into a nitrogen-filled glove box. Next, poly[N,N′-bis(4-butylphenyl)-N,N′-bis(phenyl)-benzidine] (poly-TPD) ∼ 4 mg/mL in chlorobenzene was spin-coated at a speed of 2,500 rpm for 45 s, and the film was baked at 120°C for 20 min. A 60-nm-thick nanorod film was realized by spin-coated at a spin speed of 500 rpm for 5 s and 2,000 rpm for 45 s, 10 µl of 2 mg/mL 2,2′,2″-(1,3,5-benzinetriyl)-tris(1-phenyl-1-H-benzimidazole) (TPBi) in methyl acetate was dropped onto the nanorod layer at a speed of 4000 rpm. All the devices were moved to an organic chamber connected to a glovebox, and TPBi (40 nm) was evaporated at a rate of 1 Å s−1. Finally, LiF/Al (1 nm/120 nm) were sequentially deposited using thermal evaporation under a high base vacuum pressure of 10−7 Torr at a rate of 0.1 Å s−1/ 4 Å s−1. (The evaporator is located at the 3D Convergence Center of Inha University.) The optoelectronic properties of ANR-LEDs were analyzed using a semiconductor parameter analyzer (Keithley 237) connected with a spectrophotometer (Photo Research PR-670). Before the measurement, all the devices were encapsulated with a glass lid using ultraviolet-curing epoxy resin in a glovebox filled with N2 gas. The thickness of PEDOT:PSS, Poly-TPD, and EML are 40, 15, and 60 nm, confirmed by an alpha step.
3. Results and discussion
To achieve a preferentially oriented 1D geometry in perovskite, colloidal CsPbBr3 ANRs were synthesized using the hot-injection method starting from initially non-luminescent NPDs Cs4PbBr6 NCs. This involved the oil–water reaction transformation, resulting in luminescent CsPbBr3 nanorods. The process included CsX stripping, as illustrated in Scheme 1 [Citation36–38]. Real images depicting the change from Cs4PbBr6 NCs to intermediate stages (oil–water ligand modulation) and finally to CsPbBr3 nanorods are also provided. During the CsX stripping reaction, the native alkylamine ligands partially detached from the surface of Cs4PbBr6 hexagon NCs, reducing their stability due to the removal of the ligands. Consequently, the system experienced self-organization to get stabilized as a form of the CsPbBr3 NRs, as more [PbBr6]4- octahedrons are formed.
Scheme 1. The schematic illustration of the chemical transformation of Cs4PbBr6 into CsPbBr3 nanorods due to ligand-regulated reactions at the water-oil interface. The bottom is the images of Cs4PbBr6 NCs to the intermediate stage (oil-water ligand modulation) to CsPbBr3 nanorods.

The homogeneous shape and mono-dispersity of anisotropic nanorods subjected to chemical stripping transformation were characterized by field-emission transmission electron microscopy (FE-TEM). Figure demonstrates the single crystalline nanorods with variable length and width, owing to ligand-modification associated with oil–water reaction times (e.g. times for 11–44 hrs.). Figures (a–c) reveal the ligand-modification time for 44 h, which holds an average length and width of 26 and 12 nm, corresponding to an aspect ratio (AR) of 2.2. In addition, the aspect ratios of 2.0 and 1.2 are associated with ligand-modification times of 33 and 22 h, respectively, as represented in Figure (d–i). The results showed the importance of the chemical stripping transformation of hexagonal NPDs NCs (Figure S1, Supporting Information) into high-quality nanorods. Thus, water treatment is highly dynamic within the perovskite lattice, stimulating surface atom reordering during the CsX stripping.
Figure 1. (a) Field-emission transmission electron microscope (FE-TEM) image, (b-c) the histogram of the average length and width of the CsPbBr3 nanorod for 44 h of ligand-modification times, (d) FE-TEM image, (e-f) the histogram of the average length and width of the nanorods for 33 h of ligand modification times, (g) FE-TEM image, and (h-i) the histogram of the average length and width of the nanorod for 22 h of ligand modification times.

X-ray diffraction (XRD) characterization of CsPbBr3 nanorods (mixed phase and final nanorods) and Cs4PbBr6 nanocrystals were conducted (Figure a) to verify the phase transformation of rhombohedral nanocrystals into nanorods. The characteristic peaks located at 14.95°, 21.37°, 30.45°, 37.5°, and 43.63° were assigned to (100), (110), (200), (210), and (211) diffractions of cubic perovskite phase (JCPDS No. 54-0752), and there is no extra peak ascribed to impurity or rhombohedral Cs4PbBr6 NCs. The enlarged XRD patterns of different crystal planes (100, 110, 200, 210, and 211) disclose the chemical transformation of the hexagonal NPDs NCs into nanorods, owing to ligand-regulated reactions at the water–oil interface (Figure b–f) [Citation36]. Furthermore, the optical properties of nanorods were analyzed, and PLQY as a function of chemical stripping transformation is plotted in Figure (g). The results indicated that PLQY increased to 60% as a function of the chemical stripping reaction (i.e. from 0 to 44 h). Subsequently, the value dropped to 44% after 55 h of chemical stripping reaction, attributed to the moisture sensitivity of perovskite. In terms of color purity, the nanorods exhibited narrow PL emission with a full-width at half maximum (FWHM) of 23 nm, and the corresponding absorption and PL spectra of the CsPbBr3 nanorods are displayed in Figure (h). The assembled film exhibited pure green emission with a PL peak of ∼517 nm, revealing a bandgap of 2.41 eV. The absorption spectra of Cs4PbBr6 nanocrystals and CsPbBr3 nanorods as a function of chemical stripping transformation are plotted in Figure S2a-c. Before the reaction, we observed a clear absorption peak at around 314 nm, responsible for the Cs4PbBr6 NCs (Figure S2b). However, after 44 h of the oil–water ligand reaction, this peak disappeared since the non-luminescent Cs4PbBr6 NCs turned into the luminescent CsPbBr3 (Figure S2c). PL spectra as a function of chemical stripping transformation are plotted in Figure S2d.
Figure 2. (a) XRD pattern of nanopolyhedrons Cs4PbBr6 NCs and CsPbBr3 nanorods (mixed phase and final nanorods). Enlarged XRD pattern of (b) (100) crystal plane, (c) (110) crystal plane, (d) (200) crystal plane, (e) (210) crystal plane, and (f) (211) crystal plane of Cs4PbBr6 NCs and CsPbBr3 nanorods (mixed phase and final nanorods). (g) PLQY as a function of different ligand-modification times (0, 11, 22, 33, 44, and 55 hr), and (h) the absorption and photoluminescent (PL) spectra of CsPbBr3 nanorods (44 hr).

Figure (a) reveals the surface elemental composition of the CsPbBr3 nanorods based on X-ray photoelectron spectroscopy (XPS) analysis. The binding energy peaks of Cs 3d3/2 and Cs 3d5/2 are located at 739 and 725.04 eV, while the binding energy of Pb 4f7/2 and Pb 4f5/2 was positioned at 139 and 144 eV. Further, the 69.03 and 70.24 eV peaks correspond to Br 3d5/2 and Br 3d3/2, respectively. The corresponding XPS spectra of Cs4PbBr6 NCs and CsPbBr3 NRs are displayed in Figure S3. Compared with pristine CsPbBr3 NCs or QDs, all the peaks of Cs 3d, Pb 4f, and Br 3d of the CsPbBr3 NRs are shifted toward higher energy, resulting from the formation of the stable crystal structure [Citation39,Citation40]. Notably, the Br 3d spectrum of non-luminescent Cs4PbBr6 NCs has a single Gaussian-shaped binding energy distribution, while the luminescent CsPbBr3 NRs is composed of two peaks, as illustrated in Figures (d) and S3d. The results confirm that the chemical transformation successfully occurred from the hexagonal Cs4PbBr6 NCs into CsPbBr3 nanorods during the reaction [Citation41,Citation42].
Figure 3. (a) XPS spectra of CsPbBr3 nanorods film. High-resolution XPS spectra of (b) Cs 3d, (c) Pb 4f, and (d) Br 3d of nanorods.

The angle-dependent PL (ADPL) was additionally performed to disentangle the horizontal TDM (Θ) in a colloidal nanorods-based thin film (Figure a). The horizontal TDM is defined as Θ = p∥︀/ (p∥︀ + p⊥), where p∥︀ and p⊥ represent contributions from the horizontal and vertical components of the optical TDMs. The higher value of Θ makes more light extraction from the vertically stacked LEDs [Citation10,Citation13]. In this study, we employed the ADPL measurement to determine the intensity of p-polarized emission at various detection angles. We used the optical simulation to fit the experimental data using the classical dipole radiation model [Citation43–48]. Therefore, we can evaluate the value of Θ. Figure (a) includes the experimental (dotted black line) and the fitted data (black line), which were perfectly matched. For the comparison, isotropic and perfect horizontal Θ cases are also indicated. The result shows that the nanorod-based perovskite emitter has the Θ value of 73%, which is higher than for an isotropic geometry with a value of 67% (red line). This analysis emphasizes the anisotropic nature of as-prepared colloidal nanorods, which is beneficial to improve the outcoupling efficiency of perovskite LEDs. In addition to our finding in 1D geometry, a comparison with earlier reported TDM values in Qi-2D and 0D-QD-based perovskites are listed in Table S1. The ADPL at different ligand-modifications times is also indicated in Figure S4.
Figure 4. (a) Angle-dependent photoluminescence intensity of the nanorods, where isotropic (red) and perfect horizontal (blue) orientation cases are added for comparison; and (b) refractive index (n) and extinction coefficient (k) of the thin film of anisotropic nanorods

The discrepancy of large refractive indices between the sandwich structure of high-refractive-index perovskite emitter (n > 2.3) and low-refractive-index carrier-injecting layers (n ∼ 1.5) generally confine 70-80% generated photons. Then, the high extinction coefficient (k) combined with divergence in n values in multilayered PeLEDs configuration impedes outcoupling efficiency. Thus, the optical constants of the perovskite emissive layer are the key to manipulating light outcoupling in PeLEDs. Figure (b) exhibits the n and k curves of anisotropic nanorods measured by an ellipsometer. Intriguingly, the nanorod-based emitting layer has an n of around 1.86 at 517 nm. The nature of capping ligands makes the n value lower than pure inorganic CsPbBr3 having a value of over 2.0 [Citation10,Citation49]. The low n value is due to the long alkylamine ligand (OA, OLA) and the amount of unreacted nucleating agent (OA, OLA, and ODE) ligands in the colloidal dispersion. Similarly, the n value of 1.82 at 620 nm was recently reported from organic–inorganic MAPb(BrxI1-x)3 NCs capped with alkylamine ligands [Citation50]. Additionally, the Maxwell–Garnett formula predicts the n of 1.87 for FA0.5MA0.5PbBr3 anisotropic NCs superlattice and ligands composite with the n of 1.65 at 530 nm [Citation12].
The anisotropic nanorod-based thin film was then adopted as an EML to evaluate the electroluminescence (EL) performance. Before the device fabrication, we checked the topography of the EML using atomic force microscopy (AFM) (Figure S5 a-b). The root-mean-square (RMS) roughness of the nanorods-based film was 0.46 nm. The smooth surface morphology of the perovskite nanorods-based thin film is expected to be desirable for the EML in LEDs [Citation51]. The ANR-LEDs were fabricated based on the device architecture of ITO/PEDOT:PSS/Poly-TPD/nanorod-based EML/TPBi/LiF/Al. Figure (a) displays the energy level diagram, presenting the corresponding valence band maximum (VBM) and conduction band minimum (CBM) values taken from the reported literature [Citation37]. The schematic illustration of PeLEDs and the thickness of functional layers are depicted in Figure (b,c). The current density–voltage-luminance (J-V-L) curves of the device are illustrated in Figure (d). The ANR-LEDs displayed a turn-on voltage of 3.3 V. The maximum brightness of 186 cd/m2 was obtained at a driving voltage of 4.8 V, corresponding to a current density value of 1.12 mA/cm2. Furthermore, the EQE-J characteristic of ANR-LEDs is shown in Figure (e). Notably, the best device showed a maximum EQE of 18.4% at a current density of 0.04 mA/cm2 and a 19 cd/m2 luminance. The histogram of average EQE and luminance disclosing the reproducible performance of ANR-LEDs (Figure f,g). The peak current efficiency (CE) of 45.9 cd/A and power efficiency (PE) of 40.0 lm/W were obtained with a J value of 0.04 mA/cm2 (Figure h). The EL spectra, as a function of applied voltage, exhibited a well-defined green emission peak centered at 513 nm, indicating excellent color stability of nanorods under high operating voltage from 3.3 to 5.1 V (Figure i). The EL spectra of the ANR-LEDs showed a slightly blue-shifted characteristic by 4 nm at high current owing to free carrier emission, which is congruent with PeLEDs [Citation52]. The inset of Figure (i) is an ANR-LED operating image under 4.8 V, corresponding to a CIE 1931 color coordinate of (0.073, 0.71) (Figure S6). To address the challenge of low luminance in the current ANR-LEDs, controlling the thickness of EML would be necessary to form a very thick layer of more than several hundred nanometers. A thick EML might trigger the photon-recycling process [Citation53,Citation54] to enhance the luminance, as it can randomize the light propagation direction trapped in the waveguide mode [Citation55]. However, forming a reproducible thick film using ANR-perovskites is another challenge, and this is under investigation. Table summarizes the comparison of the optoelectronic properties of CsPbBr3-based PeLEDs among the previously reported ANRs-based PeLEDs, and the results show that the obtained EQEs are one of the highest among the reported perovskite LEDs based on pure CsPbBr3 emitter.
Figure 5. (a) The energy level diagram of functional layers in PeLEDs, (b-c) schematic illustration and cross-sectional TEM images of PeLEDs, (d) current density-voltage-luminance characteristics, (e) external quantum efficiency vs. current density curve, (f-g) histogram of maximum external quantum efficiency and luminance values. (h-i) current efficiency-power efficiency vs. current density, and EL spectra of ANR-LEDs. The inset of Figure (i) is the operation image of the LED under 4.8 V.

Table 1. Comparison of optoelectronic properties of PeLEDs based on CsPbBr3.
A single-carrier typed hole-only device (HOD) was fabricated to quantify the defect density and the evolution of the space-charge-limited current (SCLC) at different biases in anisotropic nanorods. Thermally deposited MoOx (10 nm) was employed as an electron blocking layer and Al (120 nm) as an electrode with complete devices configuration of ITO/PEDOT:PSS/Poly-TPD/Nanorods/MoOx/Al (Figure ). All the logarithmic J-V curves consist of three dominant regions, i.e. ohmic, the trap-filled limit (TFL), and Child. In the ohmic region, n = 1, current linearly increases with voltage, indicating the trap-state filling process. In the trap-filling region, the current display a rapid non-linear increasing behavior in the high voltage stage. The starting point of the second stage means the limitation of trap filling, where the voltage in this point (VTFL) is proportional to the trap density and can be stated as , where e, nt, ϵ0, ϵ, and L are the elementary charge, trap density, vacuum dielectric constant, relative dielectric constant and thickness of the perovskite film (∼ 60 nm). The HOD based on AR∼2.2 exhibits a VTFL of 0.72 V, corresponding to a hole-trap density of 4.8 × 1017 cm−3. Further, the hole-trap densities of 1.3 × 1018 cm−3 and 1.82 × 1018 cm−3 are related to HOD based on AR∼ 2.0, and 1.2, respectively. These results suggest that ANRs-based on the aspect ratio 2.2 experienced a remarkable reduction in trap-state densities (Table S2) [Citation7,Citation10].
4. Conclusion
In summary, we synthesized colloidal CsPbBr3-based ANRs through the ligand-regulated transformation method. The oil–water interface between the Cs4PbBr6 cyclohexane solution and water provides a semi-porous membrane that acts as the space for lead cations to insert into nano polyhedrons NCs to form nanorod shapes. The synthesized ANRs exhibited an enhanced horizontal TDM of 73% and a refractive index of 1.86 at 517 nm. We fabricated ANRs-based LEDs by employing 1D-ANRs as emitters. The ANR-LEDs achieved a maximum current efficiency of 45.9 cd/A and a maximum EQE of 18.4%. The results are attributed to the enhanced light outcoupling using the ANR-based perovskite EML.
Disclosure statement
No potential conflict of interest was reported by the author(s).
Additional information
Funding
Notes on contributors

Muhammad Imran Saleem
Muhammad Imran Saleem received his PhD degree from the School of Physics Beijing Institute of Technology, China. Since 2022, he has been working as a postdoctoral researcher at the Department of Materials Science and Engineering at Inha University, Incheon, South Korea. His research focuses on perovskite optoelectronic devices.

Jeong-Hwan Lee
Jeong-Hwan Lee received his PhD from the Department of Materials Science and Engineering at Seoul National University under the supervision of Prof. Jang-Joo Kim in 2014. During 2014–2017, he carried out his postdoctoral work in Imec, Belgium. Then, he joined the faculty at Inha University in 2017. His research interests have centered on organic semiconductors for electronics and photonics, which are regarded as new materials for information processing. His current research topics include electroluminescence and sensing by using various material systems from organic to organic/inorganic hybrid materials. In electroluminescence, the fundamental physics of materials and EL devices, degradation mechanism, new device structures to improve EL efficiency, and novel devices such as lasers are investigated.
References
- Q.A. Akkerman, G. Rainò, M.V. Kovalenko, and L. Manna, Nat. Mater. 17, 394–405 (2018).
- K. Lin, J. Xing, L.N. Quan, F.P.G. de Arquer, X. Gong, J. Lu, L. Xie, W. Zhao, D. Zhang, C. Yan, W. Li, X. Liu, Y. Lu, J. Kirman, E.H. Sargent, Q. Xiong, and Z. Wei, Nature 562 (7726), 245–248 (2018).
- Z.-K. Tan, R.S. Moghaddam, M.L. Lai, P. Docampo, R. Higler, F. Deschler, M. Price, A. Sadhanala, L.M. Pazos, D. Credgington, F. Hanusch, T. Bein, H.J. Snaith, and R.H. Friend, Nat. Nanotechn 9 (9), 687–692 (2014).
- M. Sulaman, S. Yang, A. Bukhtiar, P. Tang, Z. Zhang, Y. Song, A. Imran, Y. Jiang, Y. Cui, L. Tang, and B. Zou, Adv. Funct. Mater. 32(28), 2201527 (2022).
- W.H. Jeong, Z. Yu, L. Gregori, J. Yang, R.S. Ha, W.J. Jang, H. Song, H.J. Park, D.E. Jung, H.M. Song, H.S. Park, J.H. Snaith, A. Boretti, F. De Angelis, D. Meggiolaro, J. Lee, H. Choi, and R.B. Lee, J. Mater. Chem. A 9 (47), 26750–26757 (2021).
- H. Song, J. Yang, W.H. Jeong, J. Lee, H.T. Lee, W.J. Yoon, H. Lee, J.A. Ramadan, J.D.R. Oliver, C.S. Cho, G.S. Lim, W.J. Jang, Z. Yu, T.J. Oh, D.E. Jung, H.M. Song, H.S. Park, R.J. Durrant, J.H. Snaith, U.S. Lee, R.B. Lee, and H. Choi, Adv. Mater 35 (8), 2209486 (2023).
- C. Wei, W. Su, J. Li, B. Xu, Q. Shan, Y. Wu, F. Zhang, M. Luo, H. Xiang, Z. Cui, and H. Zeng, Adv. Mater 34 (10), 2107798 (2022).
- V. Kovalenko Maksym, L. Protesescu, and I. Bodnarchuk Maryna, Science 358 (6364), 745–750 (2017).
- X. Shen, K. Kang, Z. Yu, H.W. Jeong, H. Choi, H.S. Park, D.S. Stranks, J.H. Snaith, R.H. Friend, and R.B. Lee, Joule 7 (2), 272–308 (2023).
- S. He, N. Kumar, H. Beng Lee, K.-J. Ko, Y.-J. Jung, J. Il Kim, S. Bae, J.-H. Lee, and J.-W. Kang, Chem. Eng. J 425, 130678 (2021).
- D.J. Kim, S.-H. Baek, W.-S. Kim, K.-H. Ahn, and J.-H. Lee, J. Mater. Chem C 10 (22), 8602–8608 (2022).
- S. Kumar, T. Marcato, F. Krumeich, Y.-T. Li, Y.-C. Chiu, and C.-J. Shih, Nat. Commun 13 (1), 2106 (2022).
- S. Jeon, L. Zhao, Y.-J. Jung, J.W. Kim, S.-Y. Kim, H. Kang, J.-H. Jeong, B.P. Rand, and J.-H. Lee, Small 15 (8), 1900135 (2019).
- L. Zhang, F. Yuan, J. Xi, B. Jiao, H. Dong, J. Li, and Z. Wu, Adv, Funct. Mater 30 (40), 2001834 (2020).
- H. Li, H. Lin, D. Ouyang, C. Yao, C. Li, J. Sun, Y. Song, Y. Wang, Y. Yan, Y. Wang, Q. Dong, and W.C.H. Choy, Adv. Mater 33 (15), 2008820 (2021).
- J. Pan, Y. Shang, J. Yin, M.D. Bastiani, W. Peng, I. Dursun, L. Sinatra, A.M. El-Zohry, M.N. Hedhili, A.-H. Emwas, O.F. Mohammed, Z. Ning, and O.M. Bakr, J. Am. Chem. Soc 140 (2), 562–565 (2018).
- L. Lei, D. Seyitliyev, S. Stuard, J. Mendes, Q. Dong, X. Fu, Y.-A. Chen, S. He, X. Yi, L. Zhu, C.-H. Chang, H. Ade, K. Gundogdu, and F. So, Adv. Mater 32 (16), 1906571 (2020).
- H.-J. Cheon, S.-J. Woo, S.-H. Baek, J.-H. Lee, and Y.-H. Kim, Adv. Mater 34 (50), 2207416 (2022).
- H.J. Park, J.-H. Jang, J.-H. Lee, and D.-H. Hwang, ACS Appl. Mater. Interf 14 (30), 34901–34908 (2022).
- H. Zhou, S. Yuan, X. Wang, T. Xu, X. Wang, H. Li, W. Zheng, P. Fan, Y. Li, L. Sun, and A. Pan, ACS Nano 11 (2), 1189–1195 (2017).
- Y. Gao, M.C. Weidman, and W.A. Tisdale, Nano Lett 17 (6), 3837–3843 (2017).
- M.J. Jurow, T. Morgenstern, C. Eisler, J. Kang, E. Penzo, M. Do, M. Engelmayer, W.T. Osowiecki, Y. Bekenstein, C. Tassone, L.-W. Wang, A.P. Alivisatos, W. Brütting, and Y. Liu, Nano Lett 19 (4), 2489–2496 (2019).
- J. Jagielski, S.F. Solari, L. Jordan, D. Scullion, B. Blülle, Y.-T. Li, F. Krumeich, Y.-C. Chiu, B. Ruhstaller, E.J.G. Santos, and C.-J. Shih, Nat. Commun 11 (1), 387 (2020).
- R. Scott, J. Heckmann, A.V. Prudnikau, A. Antanovich, A. Mikhailov, N. Owschimikow, M. Artemyev, J.I. Climente, U. Woggon, N.B. Grosse, and A.W. Achtstein, Nat. Nanotechn 12 (12), 1155–1160 (2017).
- S. Wang, J. Yu, M. Zhang, D. Chen, C. Li, R. Chen, G. Jia, A.L. Rogach, and X. Yang, Nano Letters 19 (9), 6315–6322 (2019).
- B. Zhao, S. Bai, V. Kim, R. Lamboll, R. Shivanna, F. Auras, J.M. Richter, L. Yang, L. Dai, M. Alsari, X.-J. She, L. Liang, J. Zhang, S. Lilliu, P. Gao, H.J. Snaith, J. Wang, N.C. Greenham, R.H. Friend, and D. Di, Nat. Photon 12 (12), 783–789 (2018).
- Q. Zhang, M.M. Tavakoli, L. Gu, D. Zhang, L. Tang, Y. Gao, J. Guo, Y. Lin, S.-F. Leung, S. Poddar, Y. Fu, and Z. Fan, Nat. Commun 10 (1), 727 (2019).
- Y. Shen, L.-P. Cheng, Y.-Q. Li, W. Li, J.-D. Chen, S.-T. Lee, and J.-X. Tang, Adv. Mater 31 (24), 1901517 (2019).
- D. Zhang, Q. Zhang, B. Ren, Y. Zhu, M. Abdellah, Y. Fu, B. Cao, C. Wang, L. Gu, Y. Ding, K.-H. Tsui, S. Fan, S. Poddar, L. Shu, Y. Zhang, D.-B. Kuang, J.-F. Liao, Y. Lu, K. Zheng, Z. He, and Z. Fan, Nat. Photon 16 (4), 284–290 (2022).
- G. Walters, L. Haeberlé, R. Quintero-Bermudez, J. Brodeur, S. Kéna-Cohen, and E.H. Sargent, J. Phys. Chem. Lett 11 (9), 3458–3465 (2020).
- J. Cui, Y. Liu, Y. Deng, C. Lin, Z. Fang, C. Xiang, P. Bai, K. Du, X. Zuo, K. Wen, S. Gong, H. He, Z. Ye, Y. Gao, H. Tian, and B. Zhao, J. Wang and Y. Jin, Sci. Adv 7 (41), 8458 (2021).
- M.J. Jurow, T. Lampe, E. Penzo, J. Kang, M.A. Koc, T. Zechel, Z. Nett, M. Brady, L.-W. Wang, A.P. Alivisatos, S. Cabrini, W. Brütting, and Y. Liu, Nano Lett 17 (7), 4534–4540 (2017).
- D.A.B. Miller, D.S. Chemla, T.C. Damen, A.C. Gossard, W. Wiegmann, T.H. Wood, and C.A. Burrus, Phys. Rev. Lett 53 (22), 2173–2176 (1984).
- S. Schmitt-Rink, D.S. Chemla, and D.A.B. Miller, Adv, Phys 38 (2), 89–188 (1989).
- G. Walters, M. Wei, O. Voznyy, R. Quintero-Bermudez, A. Kiani, D.M. Smilgies, R. Munir, A. Amassian, S. Hoogland, and E. Sargent, Nat. Commun 9 (1), 4214 (2018).
- Y. Tang, C. Yin, Q. Jing, C. Zhang, Z.-G. Yu, Z. Lu, M. Xiao, and X. Wang, Nano Lett 22 (7), 2907–2914 (2022).
- D. Yang, P. Li, Y. Zou, M. Cao, H. Hu, Q. Zhong, J. Hu, B. Sun, S. Duhm, Y. Xu, and Q. Zhang, Chem. Mater 31 (5), 1575–1583 (2019).
- J. Li, Q. Jing, S. Xiao, Y. Gao, Y. Wang, W. Zhang, X.W. Sun, K. Wang, and T. He, J. Phys. Chem. Lett 11 (12), 4817–4825 (2020).
- M. Zhang, Z.-Q. Tian, D.-L. Zhu, H. He, S.-W. Guo, Z.-L. Chen, and D.-W. Pang, New J. Chem 42 (12), 9496–9500 (2018).
- H. Wang, X. Zhang, Q. Wu, F. Cao, D. Yang, Y. Shang, Z. Ning, W. Zhang, W. Zheng, Y. Yan, S.V. Kershaw, L. Zhang, A.L. Rogach, and X. Yang, Nat. Commun 10 (1), 665 (2019).
- W. Zhu, Z. Xia, B. Shi, and C. Lü, Mater, Today Chem 24, 100880 (2022).
- X. Chen, F. Zhang, Y. Ge, L. Shi, S. Huang, J. Tang, Z. Lv, L. Zhang, B. Zou, and H. Zhong, Adv. Funct. Mater 28 (16), 1706567 (2018).
- S.-Y. Kim, W.-I. Jeong, C. Mayr, Y.-S. Park, K.-H. Kim, J.-H. Lee, C.-K. Moon, W. Brütting, and J.-J. Kim, Adv. Funct. Mater 23 (31), 3896–3900 (2013).
- J. Frischeisen, D. Yokoyama, C. Adachi, and W. Brütting, Appl. Phys. Lett 96 (7), 073302 (2010).
- H. Shin, J.-H. Lee, C.-K. Moon, J.-S. Huh, B. Sim, and J.-J. Kim, Adv. Mater 28 (24), 4920–4925 (2016).
- W.L. Barnes, J. Mod. Opt 45 (4), 661–699 (1998).
- K.A. Neyts, J. Opt. Soc. Am. A 15 (4), 962–971 (1998).
- W. Lukosz, and R.E. Kunz, J. Opt. Soc. Am 67 (12), 1607–1615 (1977).
- S. Kumar, J. Jagielski, T. Marcato, S.F. Solari, and C.-J. Shih, J. Phy. Chem. Lett 10 (24), 7560–7567 (2019).
- Y. Hassan, J.H. Park, M.L. Crawford, A. Sadhanala, J. Lee, J.C. Sadighian, E. Mosconi, R. Shivanna, E. Radicchi, M. Jeong, C. Yang, H. Choi, S.H. Park, M.H. Song, F. De Angelis, C.Y. Wong, R.H. Friend, B.R. Lee, and H.J. Snaith, Nature 591 (7848), 72–77 (2021).
- Q. Wan, W. Zheng, C. Zou, F. Carulli, C. Zhang, H. Song, M. Liu, Q. Zhang, L.Y. Lin, L. Kong, L. Li, and S. Brovelli, ACS Energy Lett 8 (2), 927–934 (2023).
- J. Dong, F. Lu, J. Wang, Y. Zhang, X. Ma, J. Zhou, D. Han, Z. Zang, and N. Wang, Chem. Eng. J 429, 132347 (2022).
- C. Cho, B. Zhao, G.D. Tainter, J.-Y. Lee, R.H. Friend, D. Di, F. Deschler, and N.C. Greenham, Nat. Commun 11 (1), 611 (2020).
- L.M. Pazos-Outón, M. Szumilo, R. Lamboll, J.M. Richter, M. Crespo-Quesada, M. Abdi-Jalebi, H.J. Beeson, M. Vrućinić, M. Alsari, H.J. Snaith, B. Ehrler, R.H. Friend, and F. Deschler, Science 351 (6280), 1430–1433 (2016).
- J. Chen, P. Ma, W. Chen, and Z. Xiao, Nano Letters 21 (19), 8426–8432 (2021).
- J. Guo, Q. Hu, M. Lu, A. Li, X. Zhang, R. Sheng, P. Chen, Y. Zhang, J. Wu, Y. Fu, G. Sun, W.W. Yu, and X. Bai, Chem. Eng. J 427, 131010 (2022).
- Y. Wei, Y. Xu, Q. Wang, J. Wang, H. Lu, and J. Zhu, Chem. Commun 56 (40), 5413–5416 (2020).
- Y. Han, S. Park, C. Kim, M. Lee, and I. Hwang, Nanoscale 11 (8), 3546–3556 (2019).
- Y. Ye, J. Wang, Y. Qiu, J. Liu, B. Ye, Z. Yang, Z. Gong, L. Xu, Y. Zhou, Q. Huang, Z. Shen, W. Wu, S. Ju, L. Yu, Y. Fu, F. Li, and T. Guo, Nano Energy 90, 106583 (2021).
- S.A. Veldhuis, Y.F. Ng, R. Ahmad, A. Bruno, N.F. Jamaludin, B. Damodaran, N. Mathews, and S.G. Mhaisalkar, ACS Energy Lett 3 (3), 526–531 (2018).
- J.-S. Yao, J.-C. Zhang, L. Wang, K.-H. Wang, X.-C. Ru, J.-N. Yang, J.-J. Wang, X. Chen, Y.-H. Song, Y.-C. Yin, Y.-F. Lan, Q. Zhang, and H.-B. Yao, J. Phys. Chem. Lett 11 (21), 9371–9378 (2020).
- L. Yang, B. Fu, X. Li, H. Chen, and L. Li, J. Mater. Chem. C 9 (6), 1983–1991 (2021).
- R. Gonzalez-Rodriguez, E. Hathaway, Y. Lin, J.L. Coffer, and J. Cui, Nanoscale 14 (17), 6417–6424 (2022).
- Z. Shi, S. Li, Y. Li, H. Ji, X. Li, D. Wu, T. Xu, Y. Chen, Y. Tian, Y. Zhang, C. Shan, and G. Du, ACS Nano 12 (2), 1462–1472 (2018).
- X. Zhang, H. Lin, H. Huang, C. Reckmeier, Y. Zhang, W.C.H. Choy, and A.L. Rogach, Nano Lett 16 (2), 1415–1420 (2016).
- Y. Dong, Y.-K. Wang, F. Yuan, A. Johnston, Y. Liu, D. Ma, M.-J. Choi, B. Chen, M. Chekini, S.-W. Baek, L.K. Sagar, J. Fan, Y. Hou, M. Wu, S. Lee, B. Sun, S. Hoogland, R. Quintero-Bermudez, H. Ebe, P. Todorovic, F. Dinic, P. Li, H.T. Kung, M.I. Saidaminov, E. Kumacheva, E. Spiecker, L.-S. Liao, O. Voznyy, Z.-H. Lu, and H.E. Sargent, Nat. Nanotechn 15 (8), 668–674 (2020).
- D. Zhang, Y. Fu, H. Zhan, C. Zhao, X. Gao, C. Qin, and L. Wang, Light: Sci, Appl 11 (1), 69 (2022).
- Q. Wan, W. Zheng, C. Zou, F. Carulli, C. Zhang, H. Song, M. Liu, Q. Zhang, L. Y Lin, L. Kong, L. Li, and S. Brovelli, ACS Energy Lett 8 (2), 927–934 (2023).
- X. Li, M. Haghshenas, L. Wang, J. Huang, E. Sheibani, S. Yuan, X. Luo, X. Chen, C. Wei, H. Xiang, G. Baryshnikov, L. Sun, H. Zeng, and B. Xu, ACS Energy Lett 8 (3), 1445–1454 (2023).