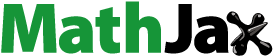
Abstract
This study utilized cellulose-based external light extraction films to enhance the outcoupling efficiency of organic light-emitting diodes (OLEDs). These films were created through a straightforward fabrication employing the biopolymers hydroxyethyl cellulose (HEC) and tannic acid (TA). We observed that the hydrogen bonding between HEC and TA could spontaneously generate a random microtextured surface on the HEC: TA films. Furthermore, the surface morphology of the HECTA film can be adjusted by varying the TA content. When applying the HECTA films as an external light-extraction layer for OLEDs, we noticed a remarkable improvement in the external quantum efficiency and current efficiency of the OLEDs up to 54% compared to those without the HECTA film. Additionally, the HECTA film displayed an outstanding ability to absorb ultraviolet light. Therefore, it is anticipated that the HECTA film can significantly contribute to prolonging the stability of OLEDs.
1. Introduction
Organic light-emitting diodes (OLEDs) present numerous advantages in lighting and displays, such as a broad color gamut and rapid response time [Citation1–3]. Despite these benefits, enhancing outcoupling efficiency remains a critical focus of research. OLEDs consist of organic multilayer structures, each with a distinct refractive index (n). Light transitioning from a higher refractive index layer to a lower one surpasses the critical angle, leading to total internal reflection (TIR). Consequently, light generated by the emission material layer becomes trapped in the substrate mode at the glass/air interface and in the waveguide mode at the indium tin oxide (ITO)/organic interface. This results in an external light extraction efficiency of approximately 20%, increasing power consumption and reducing OLED lifetime [Citation4–7].
Numerous studies propose enhancing OLED light-extraction efficiency through internal and external outcoupling films featuring light-scattering structures. Strategies include substrate optical modification and the application of high-refractive-index materials [Citation8], such as microlens arrays (MLA) [Citation9,Citation10], wrinkles [Citation11,Citation12], pyramids [Citation3], and nanostructures [Citation13,Citation14]. Among these, external light-extraction technology stands out as a direct method applicable to OLED external substrates. This approach utilizes materials with a refractive index similar to that of glass (n = ∼1.5) to mitigate TIR and increase light emission through light-scattering structures. However, current techniques, involving high-cost and intricate processes like photolithography and printing [Citation15], often employ toxic solvents and non-recyclable petrochemical plastic materials, contributing to pollution and potential health risks [Citation16,Citation17]. Therefore, adopting biomaterials and energy-efficient processes becomes imperative for addressing these concerns.
One representative biomaterial is hydroxyethyl cellulose (HEC) (Figure (a)). Derived mainly from plants and biowaste, HEC boasts biodegradability and thermal and chemical stability. Notably, HEC exhibits high transparency and a refractive index similar to that of glass (nHEC = 1.51), making it an ideal material for external light extraction films. The incorporated additives that enhance cross-linking through hydrogen bonding with HEC's hydroxyl groups further improve its mechanical properties [Citation18–20]. Tannic acid (TA), a polyphenol extracted from plants, is one such additive. As illustrated in Figure (b), TA comprises a central glucose molecule and ten gallic acid moieties, possessing 25 hydroxyl groups and 10 carbonyl groups, thereby affording a plethora of hydroxyl groups that have noncovalent interactions with biopolymers. Notably, TA forms two or three hydrogen bonds, indicating stronger crosslinking than the initial hydrogen bond [Citation21,Citation22]. Consequently, the added TA to the HEC matrix reinforces the mechanical properties of the HEC, owing to robust bonding interactions. TA is a dendritic polyphenol molecule characterized by robust intermolecular cohesion at cross-linking points [Citation23]. These interactions play a pivotal role in shaping the film's surface characteristics. Moreover, TA's UV-absorbing groups, including keto groups and active phenolic rings, are expected to enhance luminance degradation resistance and extend OLED lifetime by mitigating UV exposure effects [Citation24].
This study fabricated external light-extraction films, referred to as HECTA films, for flexible OLEDs based on cellulose. Using a simple process with HEC and TA biomaterials, the HECTA film spontaneously formed a random microstructure through interactions between HEC and TA. The random microstructure increased proportionally with TA content, resulting in a notable 54% improvement in the external quantum efficiency (EQE) and current efficiency (CE) of the OLEDs.
2. Experiment
2.1. Fabrication of HECTA film
HEC (CSA: 9004-62-0, nHEC: 1.51) and TA (Mw =1701.20, CSA: 1401-55-4, nTA: 1.704) used in this study were purchased from Sigma Aldrich Company, Korea. Figure shows a schematic of the HECTA film fabrication. The HECTA solution was prepared by adding HEC at a fixed concentration of 5 wt%, and TA at 0.5 wt% or 1.0 wt%, respectively, in deionized (DI) water with stirring at 30°C for 1 h. Subsequently, the HECTA solution was poured into a Petri dish and placed on a hotplate at 30°C overnight for drying. The surface of the HECTA film was analyzed using an optical microscope (OM) (Olympus BX-43) and field emission scanning electron microscope (FE-SEM) (S-4800). Fourier-transform infrared (FT-IR) spectra of HECTA film were recorded in 4000–400 cm-1 using an FT-IR spectrometer (HYPERION 3000, Bruker Optiks). The optical transmittance of the HECTA film was measured from 200 nm to 800 nm using a UV-Vis-NIR spectrophotometer (Lambda 1050, Perkin Elmer). We analyzed the current density–voltage and electroluminescence characteristics of OLED using the source meter (Keithley 2400) and photo research (LMS PR 650).
2.2. OLED device fabrication and characteristics evaluation
Figure illustrates OLED structures with and without the HECTA film. The fabrication of the bottom emission OLED (BEOLED) structure was fabricated as follows: indium tin oxide (ITO) (150 nm) / Dipyrazino[2,3-f: 2’,3'-h]quinoxaline-2,3,6,7,10,11-hexacarbonitrile (HAT-CN) (5 nm) / N, N′-Di(1-naphthyl)-N, N′-diphenyl-(1,1′-biphenyl)−4,4′-diamine (NPB) (55 nm) / Tris-(8-hydroxyquinoline) aluminum (Alq3) (40 nm) / LiF (1 nm) / Al (100 nm). An ITO-patterned glass substrate that is 150 nm thick underwent ultrasonic cleaning with acetone for 20 min. Subsequently, the substrate was further cleaned by boiling in isopropyl alcohol (IPA) at 220°C for 20 min. Following the cleaning steps, the glass substrates were subjected to UV-ozone treatment and transferred to a thermal vacuum evaporator. Each organic layer was deposited under a high vacuum of 1.0 × 10−6 torr, while the metal electrode was deposited at a rate of 0.5 Å/s. To prevent the ingress of oxygen and moisture, the deposited substrate was transferred to a glovebox under a nitrogen (N2) atmosphere after deposition. Subsequently, the encapsulation process was carried out.
After applying the HECTA film to the outer substrate of the fabricated BEOLED, the current density–voltage-luminance (J-V-L) characteristics were analyzed using a source meter (Keithley 2400). Before attaching the HECTA film, a refractive index matching oil (n = 1.5200± 0.002) was used to reduce the refractive index of the air layer between the HECTA film and the outside of the OLED devices. The structure of the fabricated OLED device is as follows:
Reference: Al / LiF/ Alq3/ NPB/ HAT-CN/ ITO.
HECTA_0.5: Al / LiF/ Alq3/ NPB/ HAT-CN/ ITO/ HECTA_0.5 film.
HECTA_1.0: Al / LiF/ Alq3/ NPB/ HAT-CN/ ITO/ HECTA_1.0 film.
3. Result and discussion
The surface morphologies of the HECTA films were investigated by incorporating different ratios of TA, as depicted in Figures and . All HECTA films exhibited flexible characteristics, rendering them suitable as external light-extraction films adaptable to flexible devices. Furthermore, an observed trend revealed that haze increased proportionally with TA concentration. TA serves a key part in the spontaneous formation of random microstructures. Elevated haze levels indicate increased surface scattering by these structures, suggesting that employing HECTA films as the external light-extraction layer in OLEDs could enhance outcoupling efficiency.
Figure 5. Optical microscope images of (a) HECTA_0, (b) HECTA_0.5, and (c) HECTA_1.0 film and field emission scanning electron microscope images of (d) HECTA_0, € HECTA_0.5, and (f) HECTA_1.0 film.
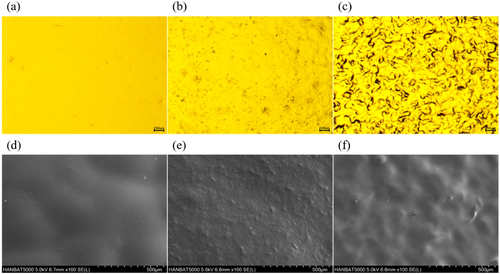
To validate the high-haze surfaces of the HECTA films, optical microscopy and field-emission scanning electron microscopy were employed, as presented in Figure . Consequently, all HECTA films exhibited random microtextured surfaces, with an increase in TA concentration, resulting in a more predominantly random microstructure morphology. Particularly, the HECTA_1.0 film, characterized by the highest TA content, displayed large microstructures (Figure (c) and (f)). The formation of these microstructures is attributed to the robust interaction between HEC and TA. In essence, the presence of random microtextured surfaces in the HECTA film is ascribed to the heightened cohesion of TA in regions where HEC and TA are crosslinked.
As illustrated in Figure , we utilized an FT-IR spectrometer to investigate the interactions between HEC and TA, analyzing the factors contributing to the formation of random microtextured surfaces on the HECTA films. New peaks at 1705 and 1709cm−1 were observed in the HECTA_0.5 and HECTA_1.0 films compared to the HECTA_0 film. These peaks correspond to the C = O stretching vibrations of the carbonyl groups, confirming the presence of hydrogen bonds between the hydroxyl (O-H) groups of HEC and the carbonyl groups of TA [Citation24]. Moreover, the spectra of the HECTA films, with and without TA, exhibited broad and prominent O-H peaks at 3385 3383, and 3381 cm−1, respectively. The shift in wavenumber suggests a change in the O-H bond length, indicating that forming hydrogen bonds increases the O-H bond distance. Consequently, a redshift occurs, resulting in a lower wavenumber shift in the peak. Therefore, the observed lower wavenumber shift in the HECTA_0.5 and HECTA_1.0 film spectrum confirms the formation of hydrogen bonds between HEC and TA [Citation25].
As a result, the spontaneous formation of the random microtextured surface on the HECTA films can be facilitated through two types of O-H bonding interactions: (1) between the O-H group of HEC and the C = O group of TA and (2) between the O-H group of HEC and the O-H group of TA.
To analyze the optical properties of the HECTA films with random microtextures, we measured the total transmittance (Tt), diffuse transmittance (Td), and haze using a UV-Vis-NIR spectrophotometer (Figure ). The diffuse transmittance (Td) and haze were calculated using Equations (1) and (2):
(1)
(1)
(2)
(2) Figure (a) presents the total transmittance measurements of the HECTA films. The total transmittance (Tt) values of HECTA films without TA (HECTA_0) to HECTA films with 1.0 wt% TA added (HECTA_1.0) were 99.12, 92.72, and 86.60%, respectively. These findings suggest that the HECTA films are well suited to external light extraction films. Figure (b) and (c) depict measurements of diffuse transmittance (Td) and haze for the HECTA film. As the TA concentration increased, diffuse transmittance and haze of the HECTA films increased. These trends are attributed to the irregular microtextured structure formed through hydrogen bonding between HEC and TA. The average haze values for HECTA_0, HECTA_0.5, and HECTA_1.0 films were 1.24, 11.87, and 27.99, respectively. Notably, the HECTA_1.0 exhibited higher haze due to the spontaneous formation of a random microstructure. Increased TA concentration resulted in a distinctive microtextured surface, inducing light scattering through this random microstructure. Thus, the HECTA film, characterized by a refractive index similar to that of glass and a spontaneously formed random microstructure, is expected to enhance the low light-extraction efficiency of OLEDs.
Figure 7. (a) total transmittance (Tt), (b) diffuse transmittance (Td), and (c) haze of HECTA_0-1.0 films.
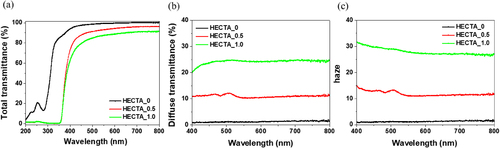
Moreover, Figure (a) reveals a significant decrease in transmittance in the UV region (200–400 nm) for the HECTA film with TA. This distinctive phenomenon arises from the abundant presence of phenolic groups in TA. While the HECTA_0 film without TA exhibited limited UV-absorbing capabilities, registering approximately 51% transmittance at 313 nm, the HECTA_0.5 and HECTA_1.0 films with TA demonstrated remarkable UV-absorbing capacity, achieving approximately 0% transmittance. The molecular configuration of TA includes various UV-absorbing functional groups, such as phenol, ketones, and other chromophores, effectively blocking UV radiation. Given that extended exposure of OLEDs to UV light can lead to issues like pixel shrinkage, reduced luminance, and shortened lifespan, the UV protection provided by the HECTA film can significantly extend the lifetime of OLEDs.
To assess the suitability of the HECTA film as an external light-extraction layer for enhancing the outcoupling efficiency of OLEDs, Figure graphs the luminance and efficiency of reference and OLED with HECTA_0.5 and HECTA_1.0 films. The application of HECTA films to OLEDs enhanced luminance, external quantum efficiency, and current efficiency. This improvement can be attributed to the surface scattering effect of a random microstructure facilitated by O-H bonding centered on TA. The HECTA film's refractive index, similar to that of glass, coupled with its light-scattering structure, effectively reduces the incident angle below the critical angle, facilitating the extraction of light that would otherwise be lost in the substrate mode. As depicted in Figure (b) and (c), the EQE and CE of OLED with HECTA films surpassed those of the reference. The EQE for HECTA_0.5 film exhibited an increase of approximately 52.1% compared to the Reference, while HECTA_1.0 film demonstrated a growth of approximately 54.2% relative to the reference. Similarly, the CE of HECTA_0.5 and HECTA_1.0 films increased by 51.82% and 54.01%, respectively, surpassing those of the reference. Table provides a comprehensive summary of the performance comparison between the reference, HECTA_0.5 and HECTA_1.0 films. Notably, OLED, featuring the HECTA_1.0 film, showcased the highest EQE and CE. The enhancement in EQE and CE was a consequence of the proportional increase in random microstructures with rising TA concentrations. As depicted in Figure , the augmentation in TA concentration led to a corresponding increase in the random microstructure of the HECTA film. The HECTA_1.0 film, distinguished by a greater number of larger random microstructures compared to the HECTA_0.5 film, played a pivotal role in improving the outcoupling efficiency of OLEDs by facilitating the extraction of a substantial amount of light through its light-scattering structures.
Figure 8. (a) Current density-voltage-luminance characteristic, (b) external quantum efficiency (EQE) - luminance characteristic, and (c) current efficiency (CE) – luminance characteristics of reference and OLED with HECTA films.
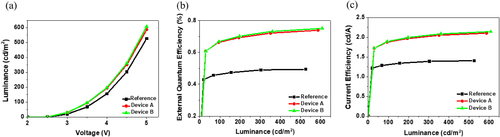
Table 1. Comparison of OLED performance with and without HECTA films.
4. Conclusion
We have developed an outcoupling film to significantly enhance the external light extraction efficiency of OLEDs. This HECTA film, fabricated with the biomaterials HEC and TA, was created straightforwardly. TA, possessing numerous O-H groups, autonomously generated random microstructures through robust bonding with HEC. Upon applying the HECTA film characterized by a randomly textured surface to OLEDs, we observed an impressive improvement of over 54% in EQE and CE compared to the reference. Consequently, we anticipate widespread adoption of the HECTA film in the OLED industry due to its remarkable capability to enhance outcoupling efficiency.
Disclosure statement
No potential conflict of interest was reported by the author(s).
Additional information
Funding
Notes on contributors
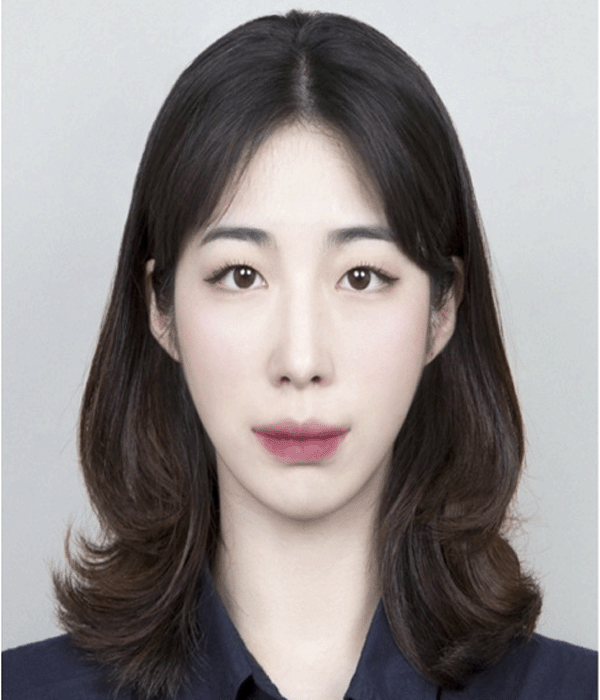
Sora Han
Sora Han received her BS from Hanbat National University, South Korea, in 2023. She is currently taking an MS in the Department of Creative Convergence Engineering at Hanbat National University.
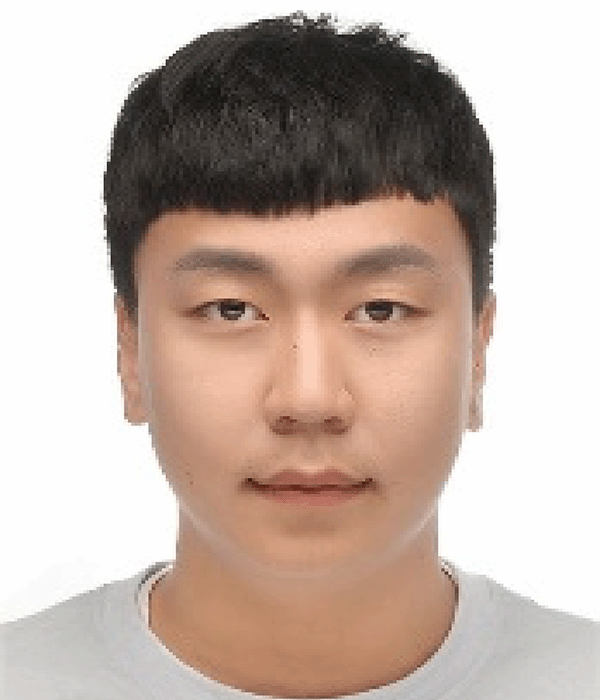
Baeksang Sung
Baeksang Sung received his BS and MS from Hanbat National University, South Korea, in 2020, and 2022, respectively. He is currently a PhD student in the Department of Creative Convergence Engineering at Hanbat National University.
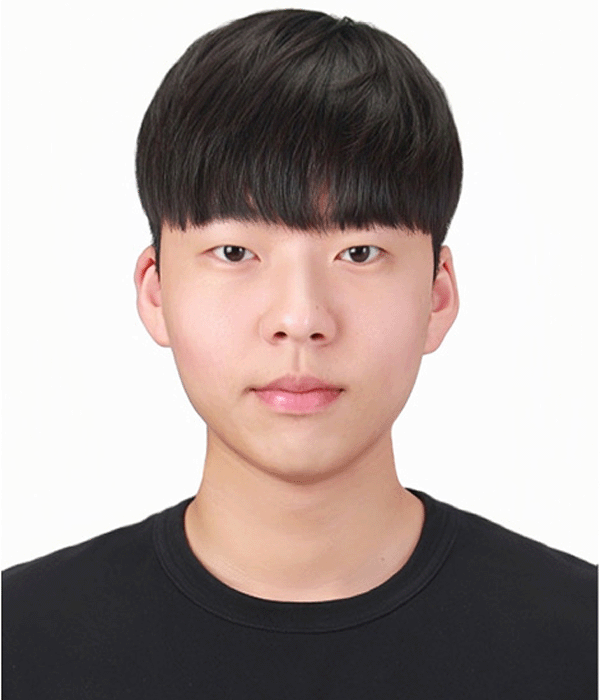
Hyunjun Jang
Hyunjun Jang is currently taking an MS degree in the Department of Creative Convergence Engineering at Hanbat National University.
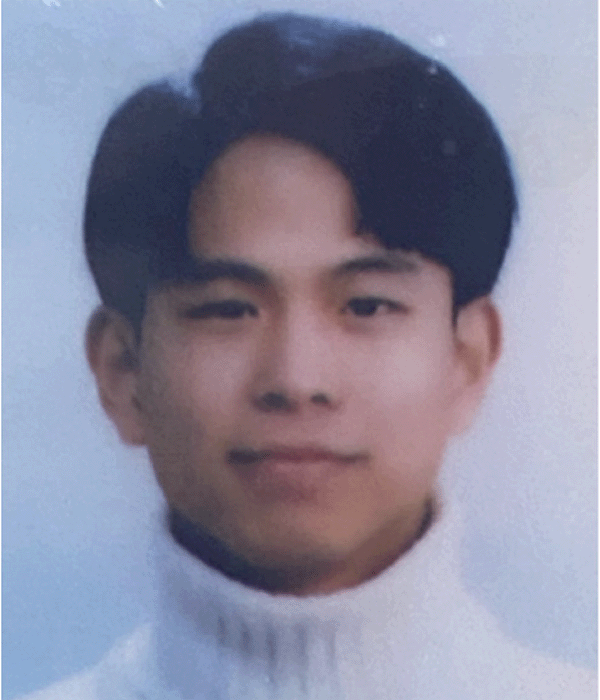
Jooho Lee
Jooho Lee is currently taking an MS degree in the Department of Creative Convergence Engineering at Hanbat National University.
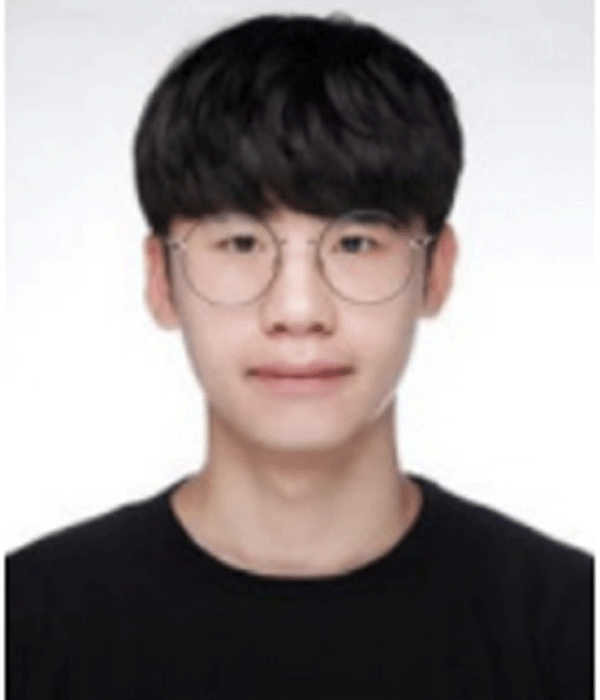
Seungwan Woo
Seungwan Woo received his BS from Hanbat National University, South Korea in 2022. He is currently taking an MS in the Department of Creative Convergence Engineering at Hanbat National University.
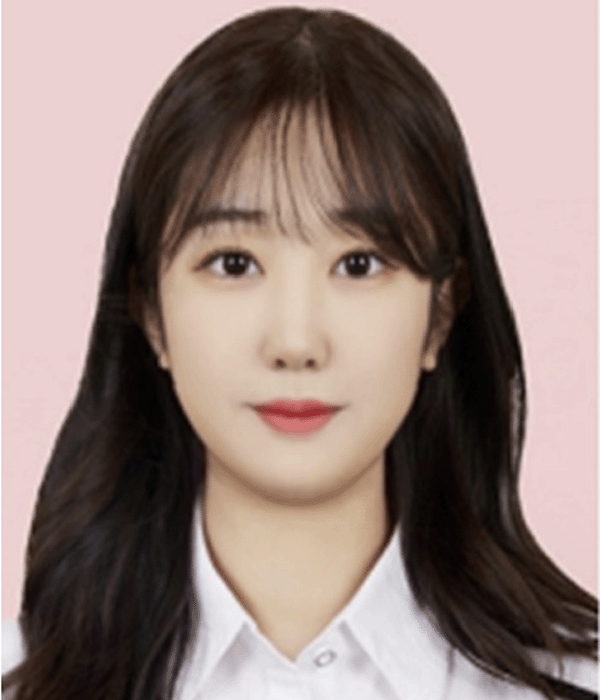
Dasol Kim
Dasol Kim received her BS from Hanbat National University, South Korea, in 2023. She is currently taking her MS in the Department of Creative Convergence Engineering at Hanbat National University.
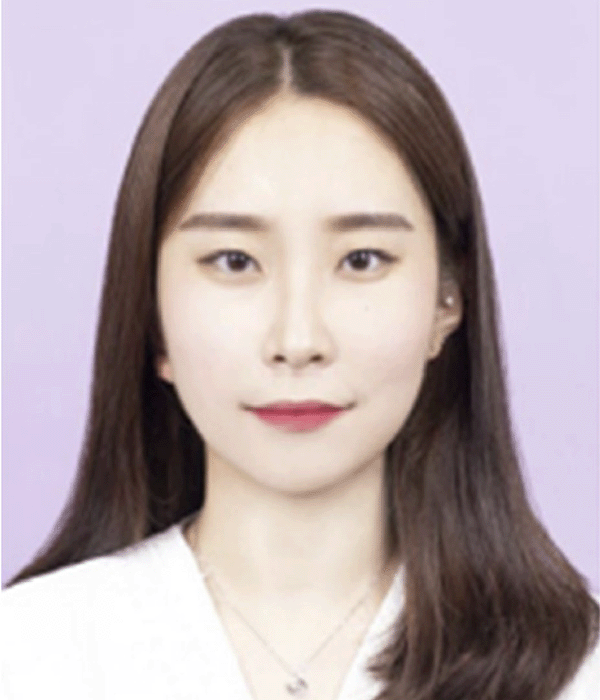
Jinhwa Kim
Jinhwa Kim received her BS from Hanbat National University, South Korea, in 2023. She is currently an MS student in the Department of Creative Convergence Engineering at Hanbat National University.
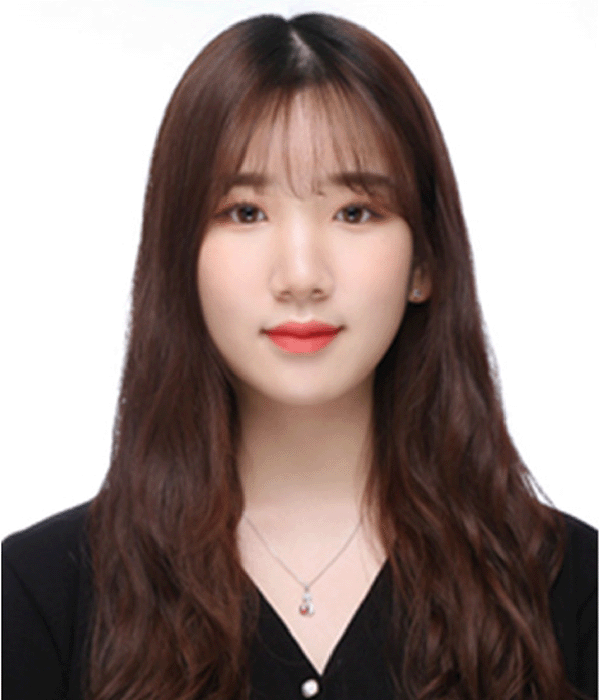
Hye-Ri Joe
Hye-Ri Joe is currently taking a BS-MS integrated degree in the Department of Creative Convergence Engineering at Hanbat National University.
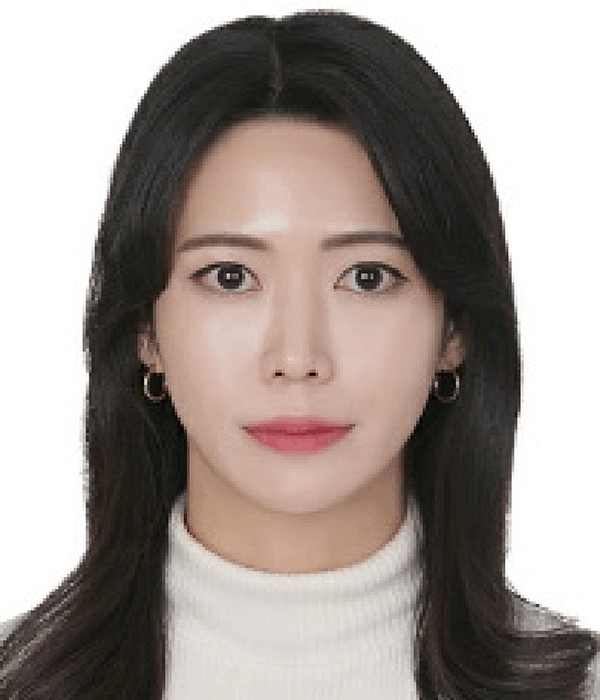
Joo Won Han
Joo Won Han received her BS, MS, and Ph.D. in Engineering from Pukyong National University, South Korea, in 2015, 2018, and 2021, respectively. She has been a post-doc researcher at Pukyong National University, Busan, South Korea, since 2021. She is currently working on highly conductive materials for organic electronic devices.
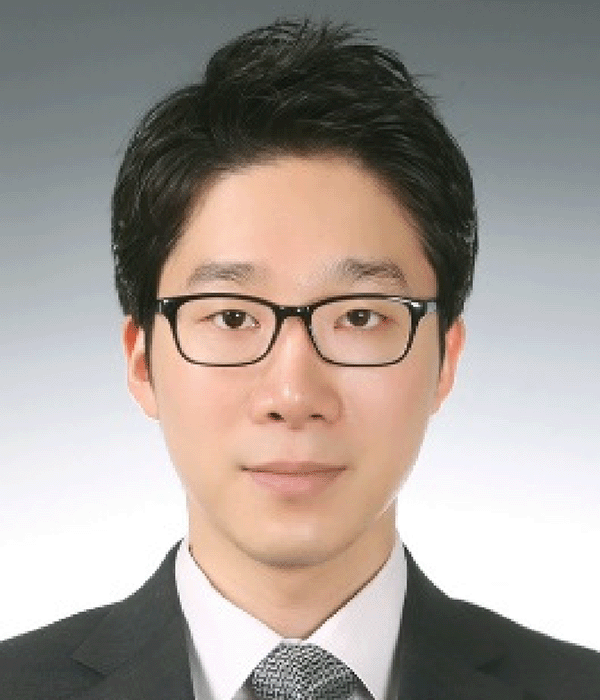
Yong Hyun Kim
Yong Hyun Kim is a professor at Pukyong National University in Korea. He received his PhD from Technische Universität Dresden, Germany, in 2013, under the supervision of Prof. Karl Leo in the Institut für Angewandte Photophysik (IAPP). After that, he worked as a post-doctoral associate at the University of Minnesota and was promoted to professor in 2014. He rejoined IAPP as a visiting professor to take a sabbatical leave in 2019. His research focuses on conductive polymers and novel device architectures for organic electronics. He published over 90 peer-reviewed papers, including his pioneering research in highly conductive polymer materials for organic optoelectronic devices.
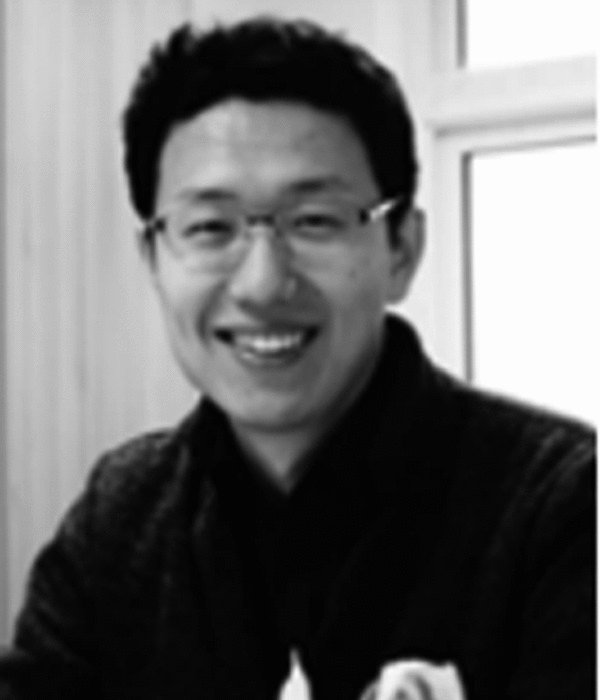
Jae-Hyun Lee
Jae-Hyun Lee received his BS, MS-PhD in Material Science and Engineering from Seoul National University, in 2011. He has been a professor at Hanbat National University, Daejeon, South Korea, since 2012 and worked on the optical and electrical characteristics of organic semiconductors for organic electronics.

Jonghee Lee
Jonghee Lee received his BS, MS, and PhD in Chemistry from the Korea Advanced Institute of Science and Technology (KAIST), Republic of Korea, in 2002, 2004, and 2007, respectively. He joined the Electronics Telecommunications Research Institute (ETRI), Republic of Korea, in 2007. Then, he moved to the Institut fur Angewandte Photophysik (IAPP) at the Technische Universitat Dresden in Germany as a post-doc researcher under an Alexander von Humboldt fellowship in 2010. After two years, he joined ETRI again in 2012. He has been an associate professor at Hanbat National University, Daejeon, South Korea, since 2018 and worked on organic light-emitting diodes (OLEDs) and bio-polymer-based optoelectronics.
References
- H.J. Jang, J.Y. Lee, G.W. Baek, J. Kwak, and J.-H. Park, Progress in the development of the display performance of AR, VR, QLED, and OLED devices in recent years, J. Inf. Disp 51 (1), 1–17 (2022).
- B. Sung, C.W. Joo, J.C. Yang, A. Gasonoo, S.W. Woo, J.-H. Lee, S. Park, and J. Lee, Application of optical property-enhancement film to improve efficiency and suppress angle dependence of top-emitting organic light-emitting diodes, J. Inf. Disp 24, 71–79 (2023).
- S.W. Woo, J. Lee, B. Sung, A. Gasonoo, J. Cho, S.-Y. Lee, S.J. Lee, Y.-S. Lee, S.-Y. Beak, J. kim, Y.H. Kim, J.-H. Lee, M.-H. Kim, and J. Lee, Improved out-coupling efficiency of organic light-emitting diodes using micro-sized perovskite crystalline template, Org. Electron. 108, 106580 (2022).
- S.-J. Zou, Y. Shen, F.-M. Xie, J.-D. Chen, Y.-Q. Li, and J.-X. Tang, Recent advances in organic light-emitting diodes: toward smart lighting and displays, Mater. Chem. Front. 4, 788–820 (2020).
- K. Saxena, V.K. Jain, and D.-S. Metha, A review on the light extraction techniques in organic electroluminescent devices, Opt. Mater. 32, 221–233 (2009).
- E.J. Bae, J.W. Kim, B.-K. Ju, D.-H. Baek, and Y.W. Park, Flexible external light extraction in organic light-emitting diodes by porous PDMS film fabricated by high-pressure steam process, Org. Electron. 108, 106575 (2022).
- J. Song, H. Lee, E.G. Jeong, K.C. Choi, and S. Yoo, Organic light-emitting diodes: pushing toward the limits and beyond, Adv. Mater. 32 (2020).
- T.-W. Khydroxyl, J.A. Spechler, K.M. Lee, C.B. Arnold, and B.P. Rand, Enhanced outcoupling in organic light-emitting diodes via a high-index contrast scattering layer, ACS Photon 2, 1366–1372 (2015).
- J.-H. Han, J. Moon, D.-H. Cho, J.-W. Shin, H.Y. Chu, J.-I. Lee, N.S. Cho, and J. Lee, Luminescence enhancement of OLED lighting panels using a microlens array film, J. Inf. Disp 19, 179–184 (2018).
- A. Kim, G. Huseynova, J. Lee, and J.-H. Lee, Enhancement of out-coupling efficiency of flexible organic light-emitting diodes fabricated on an MLA-patterned parylene substrate, Org. Electron. 71, 246–250 (2019).
- H. Cho, E. Kim, J. Moon, C.W. Joo, E. Kim, S.K. Park, J. Lee, B.-G. Yu, J.-I. Lee, S. Yoo, and N.S. Cho, Organic wrinkles embedded in high-index medium as planar internal scattering structures for organic light-emitting diodes, Org. Electron. 46, 139–144 (2017).
- S. Kang, C.W. Joo, B.-H. Ha, J. Kim, J. Lee, B.-G. Yu, N.S. Cho, J. Nhydroxyl, and J. Moon, Direct formation of random wrinkle on emission surface for improved light out coupling and stable angular spectrum of white organic light-emitting diodes, J. Lumin. 205, 66–71 (2019).
- J.-W. Shin, D.-H. Cho, J. Moon, C.W. Joo, S.K. Park, J. Lee, J.-H. Han, N.S. Cho, J. Hwang, J.W. Huh, H.Y. Chu, and J.-I. Lee,, Random nano-structures as light extraction functionals for organic light-emitting diode applications, Org. Electron. 15, 196–202 (2014).
- C.-H. Shin, E.Y. Shin, M.-H. Kim, J.-H. Lee, and Y. Choi, Nanoparticle scattering layer for improving light extraction efficiency of organic light-emitting diodes, Opti. Express. 23, 133–139 (2015).
- S. Yuan, Y. Hao, Y. Miao, Q. Sun, Z. Li, Y. Cui, H. Wang, and B. Xu, Enhanced light out-coupling efficiency and reduced efficiency roll-off in phosphorescent OLEDs with a spontaneously distributed embossed structure formed by a spin-coating method, RSC Adv. 7, 43987 (2017).
- S. Chandrasekaran, A. Cruz-Izquierdo, R. Castaing, B. Kandola, and J.L. Scott, Facile preparation of flame-retardant cellulose composite with biodegradable and water resistant properties for electronic device applications, Scientific Reports 13, 3168 (2023).
- Z. Fang, H. Zhang, S. Qiu, Y. Kuang, J. Zhou, Y. Lan, C. Sun, G. Li, S. Gong, and Z. Ma, Versatile wood cellulose for biodegradable electronics, Adv. Mater. Technol. 6, 2000928 (2021).
- A.S. Khurd, and B. Kandasubramanian, A systematic review of cellulosic material for green electronics devices, Carbhydroxylydrate Polym. Technol. Appl. 4 (2022).
- J.W. Han, A.F. Wibowo, J. Park, J.H. Kim, A. Prameswat, S.A.N. Entifar, J. Lee, S. Kim, D.C. Lim, M.-W. Moon, M.-S. Kim, and Y.H. Kim, Highly stretchable, robust, and conductive lab-synthesized PEDOT: PSS conductive polymer/hydroxyethyl cellulose films for on-skin health-monitoring devices, Org. Electron. 105, 106499 (2022).
- M. Hao, L. Li, S. Wang, F. Sun, Y. Bai, Z. Cao, C. Qu, and T. Zhang, Stretchable, self-healing, transient macromolecular elastomeric gel for wearable electronics, Microsyst. Nanoeng. 55, 5–9 (2019).
- W. Zhang, S. Roy, P. Ezati, D.-P. Yang, and J.-W. Rhim, Tannic acid: a green crosslinker for biopolymer-based food packaging films, Trends Food. Sci. Technol. 136, 11–23 (2023).
- W. Ge, S. Cao, F. Shen, Y. Wang, J. Ren, and X. Wang, Rapid self-healing, stretchable, moldable, antioxidant and antibacterial tannic acid-cellulose nanofibril composite hydrogels, Carbohydrate Polymers 224, 115147 (2019).
- H. Sun, X. Fang, Y. Zhu, Z. Yu, X. Lu, and J. Sun, Highly tough, degradable, and water-resistant bio-based supramolecular plastics comprised of cellulose and tannic acid, J. Mater. Chem. A 11, 7193–7200 (2023).
- M.A. Gwak, B.M. Hong, and W.H. Park, Hyaluronic acid/tannic acid hydrogel sunscreen with excellent anti-UV, antioxidant, and cooling effects, Int. J. Biol. Macromol 191, 918–924 (2021).
- Y.-N. Chen, C. Jiao, Y. Zhao, J. Zhang, and H. Wang, Self-assembled polyvinyl alcohol–tannic acid hydrogels with diverse microstructures and good mechanical properties, ACS Omega 3, 11788–11795 (2018).