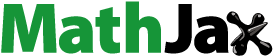
Abstract
Renewable energy requires long-duration energy storage technologies, of which vanadium flow battery is regarded as the most suitable candidate for safe and long-duration energy storage applications. However, vanadium flow batteries still suffer from capacity decay and low power density, which arise from membrane degradation and high resistance. Herein, a sulfonated poly (4,4′-diphenylether-5,5′-bibenzimidazole) (SOPBI) membrane is reported for use in vanadium redox flow batteries. The prepared SOPBI membranes show an area resistance of 0.63 Ω cm2 at room temperature after doped with 3 M H2SO4. Moreover, membranes with 3 M H2SO4 doping exhibit tensile stress at break of 15.1 MPa. Benefiting from the SOPBI membrane, the all-vanadium redox flow battery delivers a superior energy efficiency of 77.7% at 140 mA cm−2 while operating stably at a current density of 120 mA cm−2 for 200 cycles.
1. Introduction
With rapidly increased renewable energy usage, long-duration energy storage becomes an essential component of the grid [Citation1–3]. Compared to conventional lithium ion batteries, long-duration energy storage for grid requires an absolutely safe technology. Aqueous flow batteries with the merits of great safety and flexibility in power and capacity design is regarded as the most suitable technology for long-duration energy storage applications [Citation4, Citation5]. Depending on the type of redox active species used, redox flow batteries (RFBs) can be classified into different categories, including all-vanadium RFB [Citation6], iron-chromium RFB [Citation7], zinc-based RFB [Citation8] and organic RFB [Citation9]. Of all these flow batteries, the all-vanadium RFB uses the same element in both half-cells, which overcomes the drawback of cross-contamination across the membrane and is considered to be the most advanced RFB for long-duration energy storage.
In a typical all-vanadium RFB, there are a few key components, such as electrode, bipolar plate and membrane. Compared to electrode and bipolar plate, the membrane plays the most critical role in determining the flow battery performance. For instance, a good membrane should on one hand resist in vanadium ion diffusion across the membrane, which can allow a high current efficiency to be achieved [Citation10]. On the other hand, the membrane is designed to be thin enough in order to lower the cell resistance and thus enhance the voltage efficiency [Citation11]. Over the years, many different types of membranes have been proposed for VFB use. Nafion membrane is the most widely used cation exchange membrane in real applications, which has demonstrated strong resistance to V5+ oxidation as well as long-term stability [Citation12]. However, both energy efficiency and voltage efficiency are still far from expectation. Besides, the high cost of Nafion hinders further large-scale deployment of VFBs in applications. Therefore, it is necessary to develop novel membrane with low cost and high performance. In recent years, PBI membranes show a great promise in VFB application with both good mechanical property and low price [Citation13–15]. However, compared with Nafion, the pristine PBI membrane with poor conductivity in H2SO4 aqueous solution. Hence, it is urged to modify PBI membrane to realize low area resistance and high ions selectivity.
In this study, we propose a novel membrane based on sulfonated poly(4,4′-diphenyl ether-5,5′-benzimidazole) (SOPBI). The zwitterionic structure of SOPBI provides not only a superior water hygroscopicity owing to the acid − base pair formed through the hydrogen bond between sulfonic acid and amino groups, but also offers ion migration channels for ionic transport. Benefiting from such structure, the SOPBI membrane possesses both high proton conductivity and ion selectivity, dramatically improving output performance of vanadium flow battery. The flow cell with SOPBI membrane demonstrates a superior energy efficiency of 77.7% at 140 mA cm−2 and achieves long-term stable cycling over 200 cycles at 120 mA cm−2.
2. Experimental
2.1. Materials
4,4′-Oxybisbenzoic Acid (OBBA, 98.0%), 3,3′-Diaminobenzidine (DAB, 99.0%), Methanesulfonic acid (MSA, 99.0%) and N, N-dimethylformamide (DMF) were bought from Aladdin. We purchased phosphorus pentoxide, sodium hydroxide, sulfuric acid (H2SO4, 98.0%) and vanadyl sulfate (VOSO4·5H2O, 98.0%) from Sinopharm Chemical Reagent Beijing Co.
2.2. SOPBI membranes synthesis and preparation
Eaton reagent: MSA (200 g) and P2O5 (20 g) was added into a three-necked flask (250 mL) equipped with a mechanical stirring at room temperature and stirred until P2O5 was completely dissolved.
The synthesis process of SOPBI is illustrated in . For the synthesis of OPBI, firstly, OBBA, DAB and Eaton reagent was added to the three necked flask and stirred at 80 °C under N2 atmosphere. After the solution was mixed thoroughly, the temperature was heated up to 100 °C for 1h. Then, the mixture was reacted at around 140 °C for 1 h. The resulted viscosity solution was diluted by slowly addition of 500 ml deionized water. A fine precipitate was formed when the acidic solution was added dropwise into deionized water. The precipitate was washed with water and dried in a vacuum oven at 80 °C for 12 h.
Subsequently, 1 g OPBI and 10 ml sulfuric acid was added to 50 ml flask at 80 °C for 5 h. The produced polymer (SOPBI) was precipitated by dropwise addition of 10% sodium hydroxide solution under stirring. The polymer was collected by filtration and dried in a vacuum oven at 60 °C for 24 h.
The prepared polymer was dissolved in DMF with a concentration of 5 wt%. The solution was poured onto a Petri dish to cast the membrane by evaporating the solvent in an oven at 60 °C for 24 h.
2.3. Characterization
The 1H NMR spectra of SOPBI membranes were recorded on a Bruker AVANCE 600 MHz instrument using deuterated dimethylsulfoxide (DMSO‑d6) as the solvent. The Fourier transform infrared spectra (FT-IR) of SOPBI membranes were recorded by using the Bruker VERTEX70 spectrophotometer equipped with a wavenumber range of 4000 to 500 cm−1. The morphology images of membrane were taken by a SU-8000 scanning electron microscope (SEM) and JEM2100PLUS transmission electron microscopy (TEM, Japan, JEOL Ltd.), respectively. The sample was dyed by the chloroplatinic acid solution for taking the TEM images.
Mechanical properties of membranes were measured at RT by using a tensile strength instrument (CMT6502, SANS Company). Membrane samples were tailored to the dumb-bell shape with an initial dimension of 25 mm in length and 4 mm in width. The measurements were performed under ambient environment with a constant stretching rate of 5 mm min−1.
2.3.1. Ion exchange capacity, H2SO4 doping level and swelling ratio
The ion exchange capacity (IEC) of cation exchange membranes was determined by titration. The dried samples in acid form were immersed in 0.01 M KOH solutions to convert –SO3H into –SO3K. The remaining OH− was titrated with 0.01 M standard HCl solutions. The IEC value (meq/g) is calculated as follows:
(1)
(1)
where CKOH and CHCl are the concentrations of KOH and HCl, respectively; VKOH (mL) is the volume of KOH for soaking the membranes; VHCl (mL) is the consumed volume of HCl for neutralization the remained KOH, and m (g) is the weight of the dry membrane sample.
The membranes were immersed in 3 M H2SO4 at 80 °C for 24 h to determine the weight and sizes of full hydrated membrane samples. After dried at 80 °C for 48 h, the obtained weight and sizes of samples are regarded as the ones (Wdry and Sdry) of dry membranes. The H2SO4 doping level is calculated by the following equation:
(2)
(2)
Where
and
represent the weight of a membrane before and after saturated with water;
(3)
(3)
where Swet is the area or volume of the full hydrated membranes.
2.3.2. VO2+ permeability
The VO2+ permeability of membranes was determined in a diffusion cell. A membrane with an effective area of 1 cm2 was placed in between two compartments filled with 10 ml 1.0 M VOSO4/3 M H2SO4 solution in the chamber A, and 10 ml of 3.0 M H2SO4 solution in the chamber B. After that, a solution sample of 1 mL from the chamber B was collected at a time interval of 12 h and determined for vanadium ion concentration by the UV-VIS spectrometer (PerkinElmer, Lambda 365). And after each sampling, 1 mL of pristine solution (3.0 M H2SO4) was quickly added to chamber B for supplement.
2.3.3. Battery performance
A vanadium redox flow battery (VRFB) single cell was assembled by clamping a membrane between two typical commercial graphite felt electrodes (Company: Liaoning Jingu Carbon Material Co., Ltd, Product name: graphite felt electrode for flow battery, Thickness: 2.5 mm) with the effective area of 2.0 × 2.0 cm2, and then they were sandwiched by two graphite polar plates with serpentine flow channels. The compression ratio was controlled to be 20%. Two copper plates, two aluminium plates and two bakelite sheets were successively used to fix the aforementioned components. Electrolytes of 1.0 M V3+ and 1.0 M VO2+ in 3.0 M H2SO4 solution with the volume of 20 ml were used as the anolyte and catholyte, respectively. The electrolytes were cyclically pumped into the corresponding electrodes with a peristatic pump (Lead-Fluid, BT100S-1), and the corresponding flow rate was kept at 60 ml min−1. Moreover, the charge and discharge tests were carried out using the battery test system (Neware, CT-400-5V6A) with different current densities from 60 to 140 mA cm−2. The corresponding cut-off voltages were set at 0.9 and 1.65 V, respectively. The coulombic efficiency (CE), energy efficiency (EE) and voltage efficiency (VE) were calculated according to the following equations:
(4)
(4)
(5)
(5)
(6)
(6)
where V, I and t are voltage, current and time, respectively; the subscripts d and c represent the discharge and charge processes, respectively.
2.3.4. Self-discharge
The self-discharge test was carried out to confirm the high selectivity of the SOBPI membrane. In this test, 30 ml 1.0 M V2+/V3+ in a 3 M H2SO4 solution and 30 ml 1.0 M VO2+/VO2+ in a 3 M H2SO4 solution were cyclically pumped into negative and positive half cells, respectively. Self-discharge test was started at a state of charge (SOC) of 50%. And the test was ended when the open circuit voltage (OCV) was lower than 0.8 V.
2.3.5. Computational details
Quantum chemical calculations were conducted by using density functional theory (DFT) implemented in Gaussian 09 program. The structure optimization and single point energy were performed at B3LYP-GD3(BJ)/6-31G(d) level and B3LYP-GD3(BJ)/6-311G(d), respectively. The electrostatic potential was obtained by Multiwfn and VMD software. The binding energy EB between SOPBI molecules and H+ ions was defined as:
(7)
(7)
In which ECPX, ESOPBI and EH are the energy of complexes, SOPBI molecules and H+ ions, respectively.
3. Results and discussions
The SOPBI polymers were synthesized by a solution condensation reaction, which had been widely studied and reported, as illustrated in Scheme 1 [Citation16, Citation17]. The characteristic absorptions from the specific groups of SOPBI polymers are identified and indicated in the FT-IR spectra as shown in . The broad absorption band at around 3300 cm−1 is ascribed to the stretching vibration of N-H in benzimidazole. The C = C and C = N stretching of the benzimidazole ring is observed at 1623 cm−1. The characteristic absorption of S = O bands in the SOPBI appears at 1165 cm−1 [Citation18]. The 1H-NMR spectra of prepared powders and polymers are shown in . Shifts from the 2-phenoxybenesulfinic acid and benzimidazole protons are observed within 7.2 to 8.5 ppm [Citation19]. The signal appearing at 13.03 ppm is ascribed to the N–H protons of SOPBI [Citation20]. These results indicate the successful preparation of sulfonated OPBI.
The SEM was used to observe the surface and cross-section morphology of SOPBI, membranes, as shown in . The membranes show uniformly dense surfaces, owing to the strong electrostatic interactions between zwitterionic groups(-SO3H and -NH-) [Citation21]. Furthermore, benefiting from strong intermolecular forces, the thickness of SOPBI membranes achieves 10 μm. The TEM images of SOPBI membranes stained with tungstate ions are displayed in . The observed worm-like structure is attributed to the hydrophilic–hydrophobic phase separation in prepared membranes. The darker regions are ascribed to the hydrophilic ion cluster domains and the brighter regions are regarded as the hydrophobic backbones [Citation22]. As expected that the SOPBI membranes exhibit obvious ionic cluster.
The area swelling ratio, water uptake, IEC, thickness and area resistance of SOPBI membranes doped in 3 M H2SO4 solution at 80 °C for 24 h was shown in . The ion exchange capacity (IEC) represents the concentration of ionic groups in the membrane. The IEC value of the SOPBI membranes is 1.6, indicating that the SOPBI membranes have strong ability to conductive ions. Interestingly, the H2SO4 doping level and swelling ratio of SOPBI membranes is 12% and 4%, respectively. That is mainly because of the strong hydrogen bond between sulfonic acid groups and the imidazole ring [Citation12, Citation23], Which reduces the distance between the polymer chains. The uncharged amine groups (i.e. −N(CH3)2 and − NH2) can act as a proton acceptor to form the ‘acid–base pair’ structure with the sulfonic group as the proton donor [Citation12].
The area swelling ratio, water uptake, IEC, thickness and area resistance of SOPBI membranes doped in 3 M H2SO4 solution at 80 °C for 24 h was shown in . The ion exchange capacity (IEC) represents the concentration of ionic groups in the membrane. The IEC value of the SOPBI membranes is 1.6, indicating that the SOPBI membranes have strong ability to conductive ions. Interestingly, the H2SO4 doping level and swelling ratio of SOPBI membranes is 12% and 4%, respectively. That is mainly because of the strong hydrogen bond between sulfonic acid groups and the imidazole ring [Citation12, Citation23], Which reduces the distance between the polymer chains. The uncharged amine groups (i.e. −N(CH3)2 and − NH2) can act as a proton acceptor to form the ‘acid–base pair’ structure with the sulfonic group as the proton donor [Citation12]. In addition, a low area resistance is achieved at RT in 3 M H2SO4 aqueous solution by the SOPBI membrane (0.6 Ω cm2). The results were attributed to the enhanced hydrophilia of membranes, and hydrogen bonding between H2SO4 and the basic functional groups imidazole, which increase the proton conductivity of membranes ().
The mechanical properties of polymer membranes represent an indispensable factor for long-term durability in VRFB. As seen from , the SOPBI membrane shows a value of 22.1 MPa in tensile stress at break, after soaking in 3 M H2SO4 for 24 h at 80 °C, indicating that the intermolecular forces were enhanced by hydrogen bond of zwitterionic groups. Thus, the presence of a higher number of free ion-exchange groups (–SO3H) is beneficial for proton migration to satisfy the requirements of VRFB.
Figure 6. (a–d) Photos of diffusion cell at RT for 168h; (e)The concentration of the VO2+ ions in the receiving reservoir of the diffusion cell with membranes as a function of time.
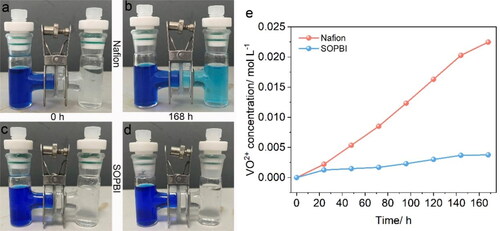
To investigate the effect of acid − base pair on ions selectivity of membrane, we tailored the vanadium-ion permeabilities of SOPBI and Nafion membranes. As displayed in , for the Nafion-based cell, the color of the VO2+ solution in the right diffusion cell gradually turns light blue after 168 h. Furthermore, as shown in , the vanadium-ion permeabilities of SOPBI (4.8 × 10−7 cm2 min−1) is apparently lower than that of the Nafion (12.8 × 10−7 cm2 min−1).
To investigate the proton conductive mechanisms and vanadium ion permeable of SOPBI membrane, the electrostatic potential (ESP) and binding energy were carried out. The molecular ESP of SOPBI units is exhibited in . The imidazole ring in SOPBI and sulfonic acid group owns more negative ESP values (more red regions), indicating that those groups more easily absorbed proton. Furthermore, as shown in the complexation ability of imidazole ring in SOPBI on H+ is −150.44 kcal mol−1, manifesting robust combinations between H+ and imidazole ring. After combined with H+, the imidazole ring not only can act as active site to conductive proton, but also restrict the vanadium-ion crossover.
Figure 7. (a) The molecular structure and (b) the electrostatic potential of SOPBI, (c) The molecular structures and (d) the binding energy of SOPBI-H1 and SOPBI-H2.
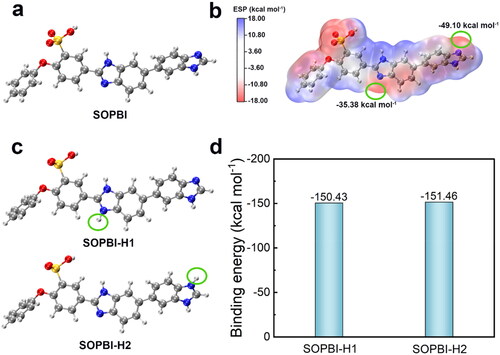
Finally, a vanadium flow cell was setup to investigate the cycling performance of the membrane (). shows the charge-discharge curves of the cell using Nafion and SOPBI membranes. Compared to Nafion, the SOPBI membrane enables a much lower overpotential at 100 mA cm−2, thereby yielding an extended discharge capacity that is 39 mAh larger than that with Nafion. With an increased current density, besides, the flow cell with SOPBI demonstrates a much higher Coulombic efficiency benefitting from the superior selectivity (). It is noted that at a high current density of 140 mA cm−2 the SOPBI membrane achieves a Coulombic efficiency of 98.7%, which is 3.5% higher than the Nafion membrane. Apart from that, the SOPBI membrane also proves to enable a much higher voltage efficiency than Nafion (), which is owing to a significantly enhanced conductivity of SOPBI. Overall, displays that the flow cell with SOPBI can realize an increased energy efficiency as compared with Nafion. Particularly, it is worth noting that at a high current density of 140 mA cm−2 SOPBI significantly outperforms Nafion in terms of energy efficiency (i.e. 7.7%). Last but not the least, the flow cell using SOPBI further delivers a stable charge-discharge cycling with an averaged 80% energy efficiency at 120 mA cm−2 for 200 cycles (), which again signifies the superiority of SOPBI for use in vanadium flow batteries.
Figure 8. (a) Schematic illustration of an all-vanadium redox flow batteries; (b) Charge-discharge curves of various membranes at a current density of 100 mA cm−2; CE (c), VE (d) and EE (e) of VRFB single cells assembled with: Nafion212 and SOPBI membranes at various current densities; (f) Cycling performance of the VRFB single cell with SOPBI at 120 mA cm−2.
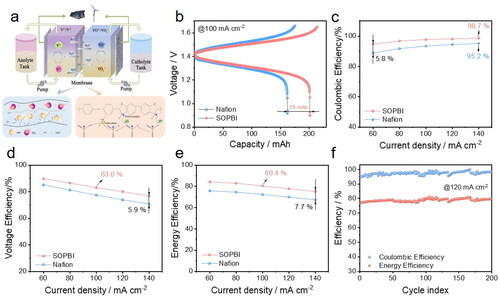
4. Conclusion
Novel polymers were successfully prepared by sulfonated Poly (4,4′-diphenylether-5,5′-bibenzimidazole) according to 1HNMR and FTIR spectra. The prepared membranes are rich in acid − base pair for proton transport. As a result, SOPBI membrane exhibited an area resistance of 0.6 Ω cm2 at RT. Moreover, the strong electrostatic interactions between zwitterionic groups benefit the membrane to exhibit less swelling with superior mechanical strength. A tensile stress at break of 22.1 MPa was achieved at ambient atmosphere. A VFB cell assembled with the SOPBI membrane demonstrates excellent performance with an energy efficiency of 77.7% at 140 mA cm−2 and achieves long-term stable cycling over 200 cycles at 120 mA cm−2. These results suggest that the SOPBI membrane is promising to be used as the membrane electrolyte in all-vanadium redox flow batteries.
Disclosure statement
No potential conflict of interest was reported by the authors.
Data availability statement (DAS)
The authors confirm that the data supporting the findings of this study are available within the article.
References
- Yuan Z, Li X, Duan Y, et al. Application and degradation mechanism of polyoxadiazole based membrane for vanadium flow batteries. J Membr Sci. 2015;488:1–11. doi: 10.1016/j.memsci.2015.04.019.
- Chae IS, Luo T, Moon GH, et al. Ultra-high proton/vanadium selectivity for hydrophobic polymer membranes with intrinsic nanopores for redox flow battery. Adv Energy Mater. 2016;6(16):1600517–1600523. doi: 10.1002/aenm.201600517.
- Jiang B, Wu L, Yu L, et al. A comparative study of nafion series membranes for vanadium redox flow batteries. J Membr Sci. 2016;510:18–26. doi: 10.1016/j.memsci.2016.03.007.
- Lu W, Yuan Z, Zhao Y, et al. High-performance porous uncharged membranes for vanadium flow battery applications created by tuning cohesive and swelling forces. Energy Environ Sci. 2016;9(7):2319–2325. doi: 10.1039/C6EE01371F.
- Zhao Y, Li M, Yuan Z, et al. Advanced charged sponge-like membrane with ultrahigh stability and selectivity for vanadium flow batteries. Adv Funct Materials. 2016;26(2):210–218. doi: 10.1002/adfm.201503390.
- Zhang Y, Wang H, Liu B, et al. An ultra-high ion selective hybrid proton exchange membrane incorporated with zwitterion-decorated graphene oxide for vanadium redox flow batteries. J Mater Chem A. 2019;7(20):12669–12680. doi: 10.1039/C9TA01891C.
- Xie C, Yan H, Song Y, et al. Catalyzing anode Cr2+/Cr3+ redox chemistry with bimetallic electrocatalyst for high-performance iron–chromium flow batteries. J Power Sources. 2023;564:232860. doi: 10.1016/j.jpowsour.2023.232860.
- Cheng Y, Zhang N, Wang Q, et al. A long-life hybrid zinc flow battery achieved by dual redox couples at cathode. Nano Energy. 2019;63:103822. doi: 10.1016/j.nanoen.2019.06.018.
- Fornari RP, Mesta M, Hjelm J, et al. Molecular engineering strategies for symmetric aqueous organic redox flow batteries. ACS Materials Lett. 2020;2(3):239–246. doi: 10.1021/acsmaterialslett.0c00028.
- Ye J, Cheng Y, Sun L, et al. A green SPEEK/lignin composite membrane with high ion selectivity for vanadium redox flow battery. J Membr Sci. 2019;572:110–118. doi: 10.1016/j.memsci.2018.11.009.
- Ahn Y, Kim D. Ultra-low vanadium ion permeable electrolyte membrane for vanadium redox flow battery by pore filling of PTFE substrate. Energy Storage Mater. 2020;31:105–114. doi: 10.1016/j.ensm.2020.06.035.
- Zhang H, Yan X, Gao L, et al. Novel triple tertiary amine polymer-based hydrogen bond network inducing highly efficient proton-Conducting channels of amphoteric membranes for high-performance vanadium redox flow battery. ACS Appl Mater Interfaces. 2019;11(5):5003–5014. doi: 10.1021/acsami.8b18617.
- Shi M, Dai Q, Li F, et al. Membranes with well-defined selective layer regulated by controlled solvent diffusion for high power density flow battery. Adv Energy Mater. 2020;10(34). doi: 10.1002/aenm.202001382.
- Di M, Hu L, Gao L, et al. Covalent organic framework (COF) constructed proton permselective membranes for acid supporting redox flow batteries. Chemical Engineering Journal. 2020;399:125833. doi: 10.1016/j.cej.2020.125833.
- Mo F, Chen Z, Liang G, et al. Zwitterionic sulfobetaine hydrogel electrolyte building separated positive/negative ion migration channels for aqueous Zn-MnO2 batteries with superior rate capabilities. Adv Energy Mater. 2020;10(16):2000035–2000047. doi: 10.1002/aenm.202000035.
- Wan L, Xu Z, Wang P, et al. H2SO4-doped polybenzimidazole membranes for hydrogen production with acid-alkaline amphoteric water electrolysis. J Membr Sci. 2021;618:118642. doi: 10.1016/j.memsci.2020.118642.
- Najibah M, Tsoy E, Khalid H, et al. PBI nanofiber mat-reinforced anion exchange membranes with covalently linked interfaces for use in water electrolysers. J Membr Sci. 2021;640:119832. doi: 10.1016/j.memsci.2021.119832.
- Yang S, Ahn Y, Kim D. Poly(arylene ether ketone) proton exchange membranes grafted with long aliphatic pendant sulfonated groups for vanadium redox flow batteries. J Mater Chem A. 2017;5(5):2261–2270. doi: 10.1039/C6TA07456A.
- Maity S, Jana T. Polybenzimidazole block copolymers for fuel cell: synthesis and studies of block length effects on nanophase separation, mechanical properties, and proton conductivity of PEM. ACS Appl Mater Interfaces. 2014;6(9):6851–6864. doi: 10.1021/am500668c.
- Yang J, Li Q, Lars N, et al. Crosslinked hexafluoropropylidene polybenzimidazole membranes with chloromethyl polysulfone for fuel cell applications. Adv Energy Mater. 2013;3(5):622–630. doi: 10.1002/aenm.201200710.
- Lv B, Yin H, Shao Z, et al. Novel polybenzimidazole/graphitic carbon nitride nanosheets composite membrane for the application of acid-alkaline amphoteric water electrolysis. J Energy Chem. 2022;64:607–614. doi: 10.1016/j.jechem.2021.05.009.
- Zhu M, Zhang X, Wang Y, et al. Novel anion exchange membranes based on quaternized diblock copolystyrene containing a fluorinated hydrophobic block. J Membr Sci. 2018;554:264–273. doi: 10.1016/j.memsci.2018.01.055.
- Anahidzade N, Abdolmaleki A, Dinari M, et al. Metal-organic framework anchored sulfonated poly(ether sulfone) as a high temperature proton exchange membrane for fuel cells. J Membr Sci. 2018;565:281–292. doi: 10.1016/j.memsci.2018.08.037.