ABSTRACT
Global crop yield has been affected by a number of abiotic stresses. Heat, salinity, and drought stress are at the top of the list as serious environmental growth-limiting factors. To enhance crop productivity, molecular approaches have been used to determine the key regulators affecting stress-related phenomena. MYB transcription factors (TF) have been reported as one of the promising defensive proteins against the unfavorable conditions that plants must face. Different roles of MYB TFs have been suggested such as regulation of cellular growth and differentiation, hormonal signaling, mediating abiotic stress responses, etc. To gain significant insights, a comprehensive in-silico analysis of OsMYB TF was carried out in comparison with 21 dicot MYB TFs and 10 monocot MYB TFs. Their chromosomal location, gene structure, protein domain, and motifs were analyzed. The phylogenetic relationship was also studied, which resulted in the classification of proteins into four basic groups: groups A, B, C, and D. The protein motif analysis identified several conserved sequences responsible for cellular activities. The gene structure analysis suggested that proteins that were present in the same class, showed similar intron-exon structures. Promoter analysis revealed major cis-acting elements that were found to be responsible for hormonal signaling and initiating a response to abiotic stress and light-induced mechanisms. The transformation of OsMYB TF into tobacco was carried out using the Agrobacterium-mediated transformation method, to further analyze the expression level of a gene in different plant parts, under stress conditions. To summarize, the current studies shed light on the evolution and role of OsMYB TF in plants. Future investigations should focus on elucidating the functional roles of MYB transcription factors in abiotic stress tolerance through targeted genetic modification and CRISPR/Cas9-mediated genome editing. The application of omics approaches and systems biology will be indispensable in delineating the regulatory networks orchestrated by MYB TFs, facilitating the development of crop genotypes with enhanced resilience to environmental stressors. Rigorous field validation of these genetically engineered or edited crops is imperative to ascertain their utility in promoting sustainable agricultural practices.
1. Introduction
Regulation of gene expression is fundamental to biological phenomena such as development, differentiation, growth, and stress responses. Transcriptional regulation of genes is a widespread phenomenon among living organisms and is dependent on the binding of transcription factors (TF), a group of proteins acting as a cis-element at the promoter region of target genes and enhancer regions of nearby genes.Citation1 This binding then paves the way for the recruitment of other elements of transcription such as basal transcription factors and RNA polymerase. From the outlook of protein structure, transcriptional factors are made up of four parts: a transcriptional regulatory domain (TRD), a DNA-binding domain (DBD), a nuclear localization signal (NLS), and an oligomerization site.Citation2 The characteristics and structure of transcriptional factors depend upon these functional regions.Citation3 Plants respond to stress events by initiating stress-responsive gene expression which is regulated by a network of transcription factor families. In recent times several stress-induced transcription factors have been identified that participate in signal transduction pathways activating a whole array of genes under their control leading to plant adaptation to stress conditions.Citation4
MYB transcription factors are a diverse group of regulatory proteins that are found in eukaryotes. They constitute the huge family of transcriptional factors in plants and interact with other transcription factors as well. The basic role of MYB proteins is in the regulation of plant primary and secondary metabolism, cell growth and differentiation, nutrient deficiency signaling, trichome development, and environmental stress responses.Citation5,Citation6 These proteins are found abundantly in plant species, and the function of multiple MYB proteins has been thoroughly investigated utilizing genetic and molecular techniques. These regulatory proteins provide an excellent opportunity to enhance plant stress tolerance due to their potential to modify the expression of several downstream genes.Citation7
Advances in biotechnology have enabled the study of genes or the manipulation of desired pathways through genetic transformation. Transformation methods, including Agrobacterium-mediated transformation, biolistics, and CRISPR/Cas9 genome editing, enable precise genetic modifications to enhance stress tolerance. Concurrently, omics approaches such as genomics, transcriptomics, proteomics, metabolomics, and phenomics provide deep insights into the genetic, protein, metabolite, and phenotypic layers of stress response. These technologies collectively facilitate the identification and manipulation of key genes and pathways, leading to the development of crop varieties better equipped to withstand environmental stresses.
So far, research into the function of MYB proteins has been mostly carried out for the development of transgenic plants with increased resistance to drought, salinity, chilling, and heavy metal stress.Citation8 However recent studies byCitation9–11 presented that MYB proteins participate in heat stress-responsive gene expression leading to plant tolerance to high temperatures. Hence these transcription factors are excellent candidates for the development of thermotolerant crop plants.
However, an extensive literature review predicted that the role of OsMYB in a transgenic dicot plant and comparative analysis of OsMYB48 between monocots and dicots has not been studied at large, despite its significance in biological roles. Hence, the present study aims to carry out the transformation of the OsMYB gene in the wild tobacco plant and its genome-wide comparison using various in-silico approaches.
2. Materials and Methods
2.1. Computational Analysis
2.1.1. Sequence Retrieval
The genomic sequence and coding sequence (CDS) of 21 dicots and 10 selected monocot plants were retrieved from NCBI (https://www.ncbi.nlm.nih.gov/). The essential data such as the gene’s location on the chromosome and the strand position of the gene was retrieved from Ensembl Plants (https://plants.ensembl.org/index.html) database. The corresponding protein sequences were obtained from the UniProt database. The proteins were subjected to conserved domain search (CD-search) in NCBI, to check the similarity index of domains and superfamily.
2.1.2. Protein Domain Analysis
The MYB-DNA binding domains were identified by protein sequences that were subjected to analysis using an online tool SMART (http://smart.embl-heidelberg.de/) and were commanded to search for PFAM domains in the sequences. The results were then beautified using GeneDoc software.
2.1.3. Phylogenetic Analysis
A phylogenetic tree was constructed to analyze the phylogenetic bond between OsMYB with corresponding genes. Protein sequences were aligned using Clustal W with default parameters. The tree was constructed via Neighbor-joining (NJ) method, using the Poisson model and pairwise deletion, with a bootstrap value of 1000 with the Molecular Evolution Genetic Analysis 11 (MEGA 11) tool.Citation12
2.1.4. Multiple Sequence Alignment
To find the sequence similarity, proteins of all MYB genes with default parameters were aligned using ClustalW (https://www.genome.jp/tools-bin/clustalw/). The aligned proteins were then edited and colored in GeneDoc software based on their conserved regions.
2.1.5. Protein Motif Analysis
The conserved protein motifs responsible for different functions were identified using the Multiple Em for Motif Elicitation (MEME) server using default parameters.Citation13 The threshold value for finding motifs was set to 30 conserved motifs.
2.1.6. Promoter Analysis
The promoter sequence upstream 3000 bp of each gene was retrieved from NCBI and was subjected to evaluate the cis-regulatory elements by PlantCARE.Citation14
2.1.7. Gene Structure Analysis
The intron/exon sites of HSFs were investigated by aligning the CDS to its corresponding genomic DNA sequence using Gene Structure Display Server v2.0 (GSDS).Citation15 The Newick tree generated from the phylogenetic analysis was exported into the GSDS input page to organize genes according to their phylogenetic relationships. Furthermore, the introns were represented by a black line, exons with red boxes while upstream/downstream regions with gray boxes.
2.1.8. Physiochemical Properties
The physiochemical properties of proteins such as amino-acid length, molecular weight (Mw), and isoelectric point (pI), were determined using the ProtPram server. All the default parameters were set to mean values.Citation16
2.1.9. Subcellular Localization
The subcellular localization of targeted genes was evaluated through CELLO server v2.5 and UniProt.Citation17
2.2. Transformation
The vector harboring the OsMYB48 gene was already present in the laboratory and was used for transformation purposes. The T/A cloning vector Pcambia3300 contained the gene of interest along with Kanamycin as a selection marker.
2.2.1. Preparation of Electro-Competent Cells of DH5α
The strain of E. coli, DH5α was streaked on solid Luria Broth (LB) media using its glycerol stock. Streaked petri plates were incubated at 37°C, overnight. After obtaining discrete colonies, a single colony of DH5α was inoculated in 5 ml of LB broth and was kept at 37°C in an incubator shaker, overnight. To achieve the OD600 at 0.5–0.8, 45 ml LB broth was added to the starter culture and placed again in the incubator shaker at 37°C. The OD600 was checked with a spectrophotometer and the culture was further processed at an OD600 of 0.5–0.8. In 50 ml falcon tubes, the grown bacterial cells were pelleted out by centrifuging at 3000 rpm, at 4°C, for 15 minutes. After centrifugation, the supernatant was discarded, and the pellet was cleaned with 15% ice-cold glycerol and again centrifuged at 3000 rpm for 15 minutes. This washing technique was repeated again and again (at least three times) for the proper removal of excess glycerol and the bacterial pellet was re-suspended in 5 mL of 15% glycerol, and 50 µl aliquots were produced and kept at −80°C for later use. To test cell viability, 10 µl and 50 µl of competent cells were dispersed on the solid LB media plates after a 16-hour incubation at 37°C.
2.2.2. Electroporation of Transformed Plasmid into DH5α Competent Cells
For electroporation, 50 µl of electrocompetent cells (DH5α) were thawed on ice and shifted to a cold cuvette along with 2 µl of transformed DNA. The solution was mixed gently and kept on ice for approximately 10 minutes. In Porator, electroporation is carried out at a specified voltage (2.5 kV/0.2 cm). These electroporated cells were resuspended immediately in 1 ml of liquid LB media in Eppendorf tubes (autoclaved) and were incubated at 37°C for 1 hour at 200 rpm with continuous shaking. The transformed mixture was spread on LB agar media containing selection marker kanamycin (50 mg/L) and incubated at 37°C for 12 hours. Non-transformed electrocompetent cells of DH5α were also streaked on LB agar media with kanamycin, as a negative control.
2.2.3. Plasmid Isolation and PCR Confirmation
Plasmid from E. coli (DH5α) was extracted via protocols established by Sambrook and Russell, (2001)Citation18 with few modifications. After 16–20 hours, single colonies were hand-picked and inoculated in 2 ml liquid LB broth containing kanamycin (50 mg/L). The inoculated broth was kept for overnight incubation at 250 rpm, at 37°C with constant shaking. The culture was then transferred into 1.5 ml autoclaved Eppendorf tubes and was centrifuged at 12,000 rpm, at 4°C for 5 minutes. The pellet obtained was resuspended after discarding the supernatant by adding 150 µl of ice-cold Solution-I (Resuspension buffer) and 50 µl RNAase A and the solution was vortexed.
Solution-II (Lysis solution) (200 µl) was added to the solution, inverted 5–10 times for proper mixing, and placed on ice for a maximum of 10 minutes. Then 300 µl of Solution-III (Neutralization buffer) was added. The Eppendorf was then vortexed for 10 seconds to disperse the solution III through bacterial viscous lysate and stored the Eppendorf on ice for 5 minutes again.
The contents were centrifuged at 14,000 rpm at 4°C for 5 minutes. The supernatant was separated and shifted to a new Eppendorf tube and the pellet was discarded. The supernatant was then vortexed by adding an equal volume of Phenol: Chloroform (24:1) and centrifuged again for 5 minutes at 14,000 rpm. A clear supernatant was observed and carefully transferred to the fresh Eppendorf tube. By adding an equal percentage of isopropanol, the double-stranded DNA was precipitated. After keeping Eppendorf tubes at room temperature for 10 minutes, the mixture was centrifuged at the speed of 14,000 rpm for 5 minutes with a set temperature of 4°C. The supernatant was carefully decanted, and the DNA was washed with 70% ethanol that settled at the bottom in the form of a pellet. The pellet dried by keeping Eppendorf tubes inverted in the air on sterilized filter paper and was suspended in 100 µl TE buffer and stored at −20°C immediately for further use. The isolated plasmid from transformed DH5α was confirmed by running a polymerase chain reaction (PCR) with OsMYB48-specific primers.
2.2.4. Preparation of Agrobacterium (LBA4404) Electro-Competent Cells
The electrocompetent cells of Agrobacterium strain LBA4404 were prepared with the same methodology as used in the preparation of DH5 cells, except that the incubation temperature was 28°C and the timeframe was 24–48 hours, respectively. The competent cells were spread on LB media plates and were placed under incubation at 28°C for 24–48 hours, to check their viability.
2.2.5. Agrobacterium Transformation with Recombinant Vector
Electrocompetent cells of Agrobacterium (LBA4404) cells were electroporated with recombinant vector through electroporation, using the same methodology as used DH5α cells. For screening, a 50–100 µl transformed mixture was spread on LB media plates containing 50 mg/L of kanamycin and incubated for 48 hours at 28°C. Colony PCR was used to confirm the transformed colonies.
2.2.6. Agrobacterium-Mediated Transformation of Nicotiana tabacum
The protocol suggested by Horsch et al.,Citation19 was followed to transfer the tobacco plants with OsMYB48 using Agrobacterium, with few modifications.
2.2.7. Selection of Plant Material
To perform Agrobacterium-mediated transformation, the tobacco species, Nicotiana tabacum var. Virginia was selected as experimental material. Seeds of tobacco were kindly provided by the National Agriculture Research Centre (NARC), Islamabad, Pakistan.
2.2.8. Seed Sterilization
Seeds of tobacco plants were surface sterilized by repeated washing with double distilled water and 70% ethanol, followed by one-time washing with 30% hypochlorite solution. Lastly, the seeds were washed with double distilled water and placed on filter paper to dry.
2.2.9. Seed Germination and Plant Growth
For germination, seeds were dried and sterilized on filter paper (autoclaved) and placed on plates containing only MS media. Plates were placed then in a growth chamber under optimal conditions of 27°C temperature and a 16:8 light/dark cycle. Young seedlings were observed after two weeks. Plants when reached the 2–3 leaf stage, were shifted to culture bottles with simple MS media for shoot elongation and rooting.
2.2.10. Explant Preparation for Transformation
The leaves of a 1-month-old tobacco plant were cut into small leaf discs without the midrib ranging from 1–2 cm and were placed adaxially on solidified full-strength MS media for pre-culture. Nodal explants were also cut into small pieces and placed alongside leaf explants on simple MS media. Approximately 10–15 explants were sited on a single MS media, sealed the plates with parafilm, and incubated at a 16/8-hour photoperiod for 48 hours.
2.2.11. Infection and Co-Cultivation
Recombinant Agrobacterium’s strain LBA4404 was streaked on a solid LB media petri plate from its glycerol stock and was kept in the dark at 28°C for 24–48 hours. After obtaining colonies, a single colony was taken and grown in 25 ml liquid LB media containing 50 mg/L Kanamycin and acetosyringone (40 g/L) to increase the bacterial virulence by incubating at 28°C with constant shaking at 250rpm for 36–48 hours. When the OD600 of suspension reached between 0.5–0.8, the suspension was transferred to the 50 ml falcon tubes and was centrifuged at 3000 rpm for 10 minutes. The bacterial pellet was resuspended in the hormone-free MS liquid media (35 ml) and the supernatant was discarded carefully. Leaf explants were placed inside the falcon tube and the tube was swirled gently 2–3 times for better attachment of bacteria to explants. Explants were kept suspended in the solution for 10 minutes and then removed by using forceps and placed on hormone-free MS media petri plates. The petri dishes were sealed with parafilm, covered with aluminum foil, and kept in a growth chamber at 25°C for co-cultivation by providing a 16-hour light and 8-hour darkness cycle for two days.
2.2.12. Selection of Transformants
Following co-culture, the infected explants were transferred to the selection media i.e., MS-Hormonal (MSH) media containing cefotaxime 500 mg/L and kanamycin 50 mg/L. The media’s pH was set to 5.8 as per the growth requirement. After autoclaving, the selection media, cefotaxime, and kanamycin were added. Cefotaxime was utilized to prevent Agrobacterium’s overgrowth, while kanamycin was applied to select the transformants. The selected explant transformants were subcultured on the fresh selection media, every 14 days.
2.2.13. Regeneration and Shooting
After 20 to 25 days, the putatively transformed explants developed calli at the cut edges of the explants. After about 3–4 weeks, adventitious shoots began to appear. Regenerated shoots were afterward transferred to large jars for appropriate growth, using the same selection media and growing conditions as before.
2.2.14. Rooting of Transformed Plants
The shoots were excised from the explant when they developed two to three internodes and transferred to large ampules containing hormone-free solid MS medium for optimal rooting.
2.2.15. Confirmation of Transgenic Plants
First, transgenic plants were selected on selection media that contained Kanamycin (50 mg/L) and growth hormones, and plants that remained green and showed putative growth were later confirmed by PCR.
2.3. Molecular Analysis
2.3.1. Genomic DNA Isolation
For the confirmation of transgenic plants, both wild-type and transgenic plants’ total genomic DNA was isolated following the CTAB (Cetyl Trimethyl Ammonium Bromide) method proposed by Murray and Thompson.Citation20 Leaves were ground in a pestle and mortar by adding liquid nitrogen into fine powder. The powder was shifted to a 1.5 ml Eppendorf tube along with 500–700 µl of pre-warmed CTAB buffer. Samples were vortexed for proper mixing and were placed in a water bath for an hour. After that, an equal amount of phenol: chloroform: isoamyl alcohol (25:24:1) was added to the tube and centrifuged the solution at 14,000 rpm for 15 minutes. The supernatant was shifted to another sterile Eppendorf tube and discard the pellet. An equal amount of pre-chilled isopropanol was mixed in a supernatant to precipitate the genomic DNA and the tube was incubated overnight on ice. The contents were centrifuged at 14,000 rpm for 15 minutes to collect the pellet and discard the supernatant. The pellet obtained was washed with 70% ethanol, resuspended in the 30–50 µl TE buffer, and stored at −20°C for future use. To confirm the presence of DNA, the gel electrophoresis method was used.
2.3.2. PCR Confirmation
The isolated genomic DNA was subjected to PCR for the confirmation of transformation. The 25 µl of PCR mixture was prepared. Amplification was processed using a Gradient Multigene Thermal Cycler (Labnet). The amplification reaction had 35 cycles of denaturation at 95°C for 5 minutes, 54°C for 90 seconds, 72°C for 60 seconds, and 72°C for 20 minutes.
The primer sequences used for the amplification are given below.
OsMYB48 (Forward): 5ʹ ATCATGAGGACCAGCACCAA 3ʹ
OsMYB48 (Reverse): 5ʹ AGGTAGCGAATAATCCGAGCA 3ʹ
2.3.3. Gel Electrophoresis and Imaging
To visualize the results of PCR, run the PCR product on 1% agarose gel along with 1kb ladder DNA for the confirmation of the size of the band. It was later visualized in the Gel Documentation System under UV light.
3. Results
3.1. Computational Analysis
3.1.1. Identification of MYB Genes
In the present study, the gene and protein sequence of Oryza sativa japonica group’s OsMYB48 was blasted against multiple dicot and monocot plants in NCBI. Genomic sequence, coding sequence (CDS), and promoter sequence of genes showing the highest similarity to the sequence were retrieved from NCBI and Ensembl Plants. The protein sequences were obtained from the UniProt. The protein sequences obtained were subjected to a Conserved Domain Database (CDD) search in NCBI to find the conserved protein domains specific to the MYB transcription factor family. Each protein confirmed the presence of highly conserved MYB-DNA binding domains. Subsequently, 1 sequence each of 21 dicots (Arabidopsis thaliana, Arabidopsis lyrata, Brassica rapa, Brassica oleracea, Brassica napus, Camelina sativa, Capsicum annuum, Capsella rubella, Gossypium arboretum, Juglans regia, Medicago truncatula, Nicotiana sylvestris, Nicotiana attenuate, Nicotiana tomentosiformis, Nicotiana tabacum, Phalaenopsis equestris, Raphanus sativus, Salvia splendens, Solanum pennellii, Solanum lycopersicum, and Solanum tuberosum) and 10 monocots (Aegilops tauschii, Brachypodium distachyon, Dioscorea cayenensis, Musa acuminata, Oryza sativa japonica, Oryza brachyantha, Panicum virgatum, Sorghum bicolor, Triticum dicoccoides, and Zea mays) plant species were selected for analysis. The gene accession numbers of MYB genes along with their plant species have been presented in . The conserved domains of these proteins i.e., the SANT domain and MYB-DNA binding domains are presented in .
Table 1. The MYB genes with their identification numbers and respective plant species.
3.1.2. Multiple Sequence Alignment
To identify the conserved domain sequence within each repeat of the OsMYB48 along with other MYB proteins, multiple sequence alignment by Clustal W was performed. In the present study, the analysis performed by Du et al.Citation21 helped to identify the highly conserved MYB and SANT domains in the protein sequences (). The SANT domain was found to be located near the N-terminal of MYB proteins and consists of approximately 50 amino acids. The basic regions of MYB DNA-binding domains had approximately 105 amino-acid residues, with rare deletions and insertions. R2-R3 MYB DNA-binding domains overlapped and followed the SANT domain. Both R2 and R3-MYB domain sequences indicated the existence of three helixes of the HTH domain, which is known for highly specific binding.
Figure 2. Multiple sequence alignment of OsMYB48 with MYB proteins of monocots and dicots. The red line represents the conserved SANT domain. The blue line represents the repeats of the conserved domain of R2-MYB, and the green line represents the repeats of the R3-MYB conserved domain. Stars indicate the presence of conserved amino acid residues in the proteins.
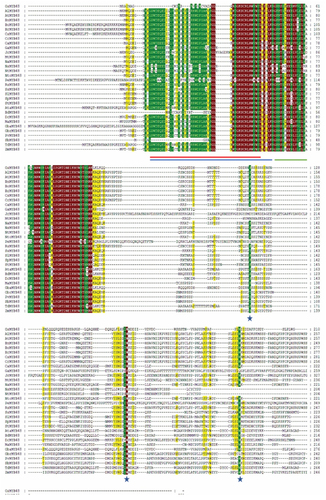
Consistent with earlier reports, which reported the presence of characteristic amino-acids in the R2-R3 MYB repeats of Arabidopsis, OsMYB and its homologs were also found to contain such as tryptophan (Trp) residues, which are a key player in sequence-specific DNA binding.Citation22 Other than that, highly conserved amino-acid residues were found in addition to the Trp residues (W). These residues included Glycine (G), Proline (P), Threonine (T), Glutamic acid (E), Glutamine (Q), Leucine (L), Valine (V), Arginine (R) Aspartic acid (D), Phenylalanine (F), Isoleucine (I), Lysine (K) and Serine (S) in R2-MYB repeats. Glycine, lysine, Serine, Leucine, Arginine, Proline, Methionine (M), Glutamic acid, Histidine (H), Tryptophan, Tyrosine (Y), and Asparagine (N) were observed to be highly conserved in R3-MYB repeats. In each repeat, between the 2nd and 3rd conserved Trp residues, the alignment predicted the distribution of significant conserved MYB domain residues. As a result, the MYB domain in the first half of each repeat was discovered to be less conserved, while the third helix of the HTH domain was revealed to be highly conserved across all proteins in each repeat. Overall, there seems to be a significant similarity between OsMYB and other MYB proteins.
3.1.3. Protein Motif Analysis
To identify the conserved motifs/regions in OsMYB48 protein with comparison to other MYB proteins, MEME was employed. In total, 30 motifs were identified in both dicot and monocot plants (). The sequence of these motifs has been presented in . Motifs 1 and 2 have the most amino acid residues (50), followed by motif 11 (49), and motif 5 (42). Motifs 1 and 3 represented the R3-MYB domain and were found to be present in all proteins. Motif 2 represents the R2-MYB domain and was found in all proteins except OsMYB48, SbMYB, and BdMYB48. The sequence is similar to the first helix of R2 repeat, which has been found to be less conserved during multiple sequence alignment. Although motif 18 with 8 residues has been determined near the N-terminal of these proteins where lies the R2-MYB domain the sequence shows high similarity with the third helix of the R2-MYB domain. The presence of long-conserved residues in the aligned sequences can reveal the conserved topology of MYB proteins across species. Motif 4 with 21 residues was found in all proteins except OsMYB48, GaMYB48, NsMYB48, NtMYB48, AtaMYB59, BdMYB48, OsMYB59, and TdMYB. Motif 5 with 42 residues was only predicted in 9 sequences, all dicot plants. This region could be a consensus sequence in dicotyledonous MYB proteins. Other motifs were found in variations in all protein sequences and showed less similarity. OsMYB48 and OsMYB59 showed high similarity and shared similar motifs except motif 2 in OsMYB59. A total of 9 motifs were found in the OsMYB48 protein sequence, of which most of the motif’s function is yet to be determined.
3.1.4. Phylogenetic Analysis of MYB48 Proteins
A neighbor-joining phylogenetic tree incorporating both dicot and monocot plants was created to gain insight into the phylogeny and evolution of OsMYB48. MEGA 11 was used to create the phylogenetic tree, which was then refined with iTOL (). The analysis performed by Islam et al.Citation23 helped in tree annotation. Based on the tree topology, the created tree was divided into four basic groups: groups A, B, C, and D. Based on their evolutionary ties, these groups were further subdivided into sub-groups. In regard to gene structure and conserved functional domains, some genes from distinct groups may differ.
According to the phylogram, class A showed the maximum number of sub-classes, followed by class B, D, and C’s MYB proteins. The gene of interest OsMYB48 was classified into class B, based on its structural and physicochemical properties. The class A was divided into four sub-classes including A1 (BnMYB, BoMYB48, BrMYB48 and RsMYB48), A2 (CsMYB48, CrMYB48, AlMYB48, and AtMYB48), A3 (JrMYB48, and MtMYB48), and A4 (SsMYB48). The class B was divided into three sub-classes including B1 (OsMYB48, OsMYB59, AtaMYB59, TdMYB, and BdMYB48), B2 (NsMYB48, and NtMYB48), and B3 (GaMYB48, and PeMYB48). Class C was divided further into two sub-classes including C1 (SlMYB48, SpMYB48, and StMYB48), and C2 (NaMYB48, NtoMYB48, and CaMYB48). Class D was divided into three sub-classes including D1 (SbMYB, ZmMYB, PvMYB, and ObrMYB), D2 (DcMYB59), and D3 (MaMYB59).
According to the analysis, OsMYB48 grouped with other monocots, and dicot plants also grouped with other dicots. OsMYB48 showed a close relationship with OsMYB59 in the phylogram, depicting a close evolutionary relationship. However, it was observed that species from the same genus as Nicotiana, Arabidopsis, Brassica, and Solanum clustered into similar groups, which predicts the conservation of MYB proteins during evolutionary processes, both in monocots and dicots. OsMYB48’s clustering with other monocots suggests that it arises from the same lineage. However, the function of the gene cannot be predicted merely on tree topology. More molecular and genetic studies can explain the role of OsMYB48 during various conditions.
3.1.5. Gene Structure Analysis
GSDS software was used to evaluate the intron-exon arrangement of all of the targeted MYB proteins to investigate the structural link between OsMYB48 and its related monocotyledonous and dicotyledonous MYB proteins. MYB genes from the same class and subclass have nearly equivalent intron-exon patterns with respect to intron count, intron phase, exon length, and overall gene length (). The introns in class A ranged from 1 to 3. In OsMYB48, only one intron was found to be interrupting the coding sequence. Other members of sub-class B1 also showed one or no intron. The sub-class B2 members possessed two introns. While sub-class B3 showed a variation of introns, ranging from 2 to 5 introns. Both class C and D possessed two introns in each sequence. The GSDS analysis was in line with the phylogenetic relationships between OsMYB48 and other MYB proteins.
Figure 6. Gene structure of OsMYB48 along with various MYB genes of monocot and dicot plants. The intron is represented by the black line, coding sequence with a red box, while the untranslated region with a grey box. The length of the introns, exons, and untranslated sections is indicated by the scale bar at the bottom.
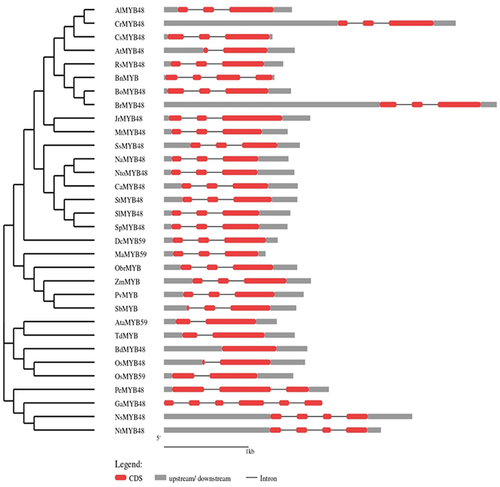
3.1.6. Physio-Chemical Properties of OsMyb48 and Relative MYB Proteins
The physio-chemical properties of OsMYB48 and other targeted proteins such as chromosomal location, amino-acid length, iso-electric point (pI), and molecular weight (Mw), were investigated using Expasy, NCBI, and Ensembl Plants (). OsMYB48 was found to be present on the forward strand of chromosome number 1. It has 210 amino-acid residues. Its isoelectric point value was calculated to be 6.29 and Mw was determined as 24,075.62 g/mol. The chromosomal location of a few genes was not available yet.
Table 2. Physiochemical properties of selected MYB genes.
3.1.7. Cis-Acting Element Analysis
The cis-regulatory elements of OsMYB48 were identified by subjecting its promoter sequence (3000 bp upstream) to the promoter analysis in the PlantCARE database. Results predicted the presence of nine regulatory elements involved in light responsiveness; eight elements involved in abiotic stress responses; five core promoter elements; one tissue-specific element, and six elements conserved for MYB-binding and recognition activities (). Abiotic stress-responsive elements included the regulatory elements acting against hormonal responses of MeJA, Gibberellin, and abscisic acid and environmental stresses such as anaerobic induction, drought, and cold. The ratio of these elements in the promoter region has been presented in . All of these elements suggest a potential role of OsMYB48 in stimulating abiotic stress responses and in cell growth and differentiation mechanisms.
Table 3. Cis-acting elements are found in the promoter region of OsMYB48..
3.1.8. Sub-Cellular Localization
The sub-cellular location of MYB proteins was identified and predicted by UniProt and CELLO server. The location of OsMYB48 and all other proteins was predicted to be in the nucleus of the cell. This suggests that these proteins play a significant role in core regulation and similar biological processes.
3.2. Functional Analysis
3.2.1. Validation of Cloning of OsMyb48 by PCR
The T/A cloning vector containing the OsMYB gene was cloned into E. coli (DH5α) cells via electroporation method. The bacterial colonies obtained were cultured in LB media containing 50 mg/L kanamycin and incubated at 37°C, at 200 rpm shaking overnight to validate the cloned gene into E. coli. Isolated plasmid from bacterial culture was confirmed by PCR using gene-specific primers. On a 1% agarose gel, a product of 633 bp () was identified, confirming the cloning of the OsMYB48 gene.
3.2.2. Transformation of OsMyb48 into Agrobacterium Tumefaciens
The OsMYB48 expression vector was transformed into the Agrobacterium tumefaciens strain (LBA4404) via the electroporation technique. The Agrobacterium-mediated transformation results were established by colony PCR by using the OsMYB48 gene-specific primers which resulted in the desired amplification ().
3.2.3. Agrobacterium-Mediated Transformation of Tobacco with OsMyb48
1-month-old tobacco plant’s young leaves were grown on MS media and were subjected to Agrobacterium-mediated transformation under controlled growth conditions and hormonal concentrations with selection marker Kanamycin (50 mg/L). After two weeks, the nodal explants and some leaf explants showed direct regeneration of the plant (). Other explants started developing small bud-like calluses at their cut edges. Both regenerated plants and calluses were subjected to continuous shifting on fresh media every 2 weeks. In the form of small shootlets, the calli began to regenerate. After 4 weeks, the shootlets had grown to the point where they could be separated from callus and explant and shifted to large vessels for optimal growth. For rooting, regenerated shoots with at least two to three nodes were transferred to hormone-free MS media. After 15 days, rooting was observed. Later, these transgenic plants were shifted to the soil in small pots to obtain a mature plant under controlled conditions of 16:8 light/dark cycles at 25°C, in a greenhouse.
Figure 10. Regeneration of transformed tobacco from leaf and stem explants growing on selection media. (a) 2-month-old young tobacco plant, (b) explants placed on selection media after infection, (c, d) direct regeneration of new plants from stem explants, (e) calli emerging from cut edges of leaf explants, (f) small plants emerging from leaf explants, (g, h) 21 days old callus, showing shoot regeneration.
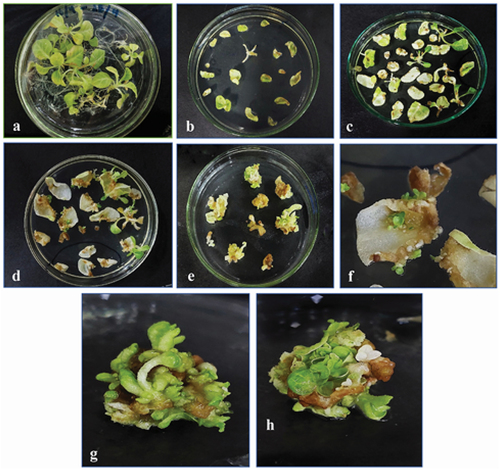
3.2.4. Validation of Transgenic Plants
To validate transgene insertion, PCR amplification was performed by means of isolated DNA from transgenic plants as a template. The OsMYBF and OsMYBR primers produced high-quality PCR products with a size of approximately 633 bp. The amplified products were then seen and documented on a 1% agarose gel ().
4. Discussion
MYB TF is a diverse and significant family of transcription factors that govern numerous defense responses to abiotic and biotic stressors, as well as hormone signaling.Citation24 The role of MYB TF in the regulation of cellular and metabolic processes in plants is studied at large and found to be promising.Citation25 Rice is a major cereal crop all over the world. Understanding the molecular mechanisms of plant MYB TF, regulation of MYB TF during environmental stresses, factors associated with enhanced resistance to abiotic stresses, identification of novel genes, and exploration of existing genetic resources are all important steps in ensuring food security in adverse environmental conditions.Citation26–29
In the present study, OsMYB TF from the rice was analyzed. The MYB TF is a highly conserved class of TF, thus its functions are also highly conserved among plants, including both monocots and dicots. In order to evaluate the evolution of OsMYB48 protein in rice and other plant species, a phylogenetic study was performed, and proteins were widely grouped into four groups. A relatively large evolutionary distance was observed between dicots and monocots, but a close relationship between genus Oryza, Aegliops, and Brachypodium is in line with botanical classification. An interesting phenomenon was observed in sub-class D1, in which a dicot PvMYB was classified with three monocot MYB proteins (). These results are in alignment with the previous study.Citation30 This further supports the results obtained in the present study and suggests a further expansion of R2-R3 MYB genes in monocots and dicots. It was also supported by the results obtained after MSA, in which R2-R3 MYB domains were found to be highly conserved among all MYB TFs.
The conserved protein domains and motif analysis results predicted the fundamental role in the functionality of OsMYB48 TF. Gene structure analysis, however, revealed variations among species, which may predict different downstream regulatory genes that take part in multiple signaling pathways. However, species with an approximately similar number of introns grouped together in a phylogenetic tree, supporting the results of this study. It was found that Brachypodium has zero introns in its gene structure, but japonica rice showed one intron in its gene structure (). The function of OsMYB48 can be determined by the location and characteristics of the cis-elements located in the gene promoter sequence. OsMYB48 is found to be mainly involved in light responses and the regulation of abiotic stress, as shown in . To date, there are only a few studies on the regulatory analysis of OsMYB48 TF. Light induces massive reprogramming of the plant transcriptomes and modulates signaling pathways involved in the increase or decrease of gene expression.
Previous and present studies have shown great similarities in amino acid sequence and gene structure between OsMYB48 and its relative closest homologue, OsMYB59.Citation31 OsMYB48’s promoter analysis revealed the cis-regulatory elements that are sensitive toward abscisic acid, gibberellin, and methyl jasmonate. It suggests their role in hormonal signaling. The fact that OsMYB48 and other MYB proteins’ subcellular location was predicted to be the nucleus of the cell, suggests their significant role in biological processes. Agrobacterium-mediated transformation of gene insertion in a plant is the most reliable and stable process. It was followed for OsMYB48’s transformation in a tobacco plant, under controlled conditions. Hormonal and antibiotic concentrations were increased or decreased to select the transformed plant and improve the transgenic plant’s growth under the light and dark cycle of growth. In a nutshell, OsMYB48 showed a promising role in major cellular processes and defense mechanisms, and its transformation in a model plant could pave the way for its expression analysis in other plants, under various environmental conditions.
Translating the findings from MYB transcription factor research into practical agricultural applications faces several challenges, including the complexity of plant stress responses, potential off-target effects of genome editing, and the regulatory hurdles associated with transgenic crops. Future research should prioritize the development of precise genome editing techniques to minimize off-target effects, comprehensive environmental and ecological impact assessments to address regulatory concerns, and the exploration of non-transgenic genome editing to circumvent the challenges associated with transgenic approaches. Additionally, interdisciplinary collaborations integrating plant physiology, molecular biology, and agronomy are essential to ensure the successful application of these findings in enhancing crop resilience to abiotic stresses.
Author Contributions
YA generated the idea. Experimental facilities and supervision were provided by TM. The experimental study was performed by YA. YA and JI wrote the manuscript. JI, SN, SH, SF; and SG helped with software, manuscript editing, and revision. SF, KAA, AAM, and TM reviewed and edited the manuscript and provided them with funds. All authors agreed to the published version of the manuscript.
Acknowledgments
The authors would like to extend their sincere appreciation to the Researchers Supporting Project Number (RSP2023R369), King Saud University, Riyadh, Saudi Arabia.
Disclosure Statement
No potential conflict of interest was reported by the author(s).
Data Availability Statement
All the raw data of this research can be obtained from the corresponding authors upon reasonable request.
Additional information
Funding
References
- Meshi T, Iwabuchi M. Plant transcription factors. Plant Cell Physiol. 1995;36:1405–20.
- Liu LJ, Zhang YC, Li QH, Sang Y, Mao J, Lian HL, Yang HQ. COP1-mediated ubiquitination of CONSTANS is implicated in cryptochrome regulation of flowering in arabidopsis. Plant Cell. 2008;20(2):292–306. doi:10.1105/tpc.107.057281.
- Du HAI, Wang YB, Xie YI, Liang ZHE, Jiang SJ, Zhang SS, Huang Y-B, Tang Y-X. Genome-wide identification and evolutionary and expression analyses of MYB-related genes in land plants. DNA Res. 2013;20(5):437–48. doi:10.1093/dnares/dst021.
- Wang H, Wang H, Shao H, Tang X. Recent advances in utilizing transcription factors to improve plant abiotic stress tolerance by transgenic technology. Front Plant Sci. 2016;7:67. doi:10.3389/fpls.2016.00067.
- Dubos C, Stracke R, Grotewold E, Weisshaar B, Martin C, Lepiniec L. MYB transcription factors in ara-bidopsis. Trends Plant Sci. 2010;15(10):573–81. doi:10.1016/j.tplants.2010.06.005.
- Ambawat S, Sharma P, Yadav NR, Yadav RC. MYB transcription factor genes as regulators for plant re-sponses: an overview. Physiol Mol Biol Plants. 2013;19(3):307–21. doi:10.1007/s12298-013-0179-1.
- Roy S. Function of MYB domain transcription factors in abiotic stress and epigenetic control of stress response in plant genome. Plant Signal Behav. 2016;11(1):e1117723. doi:10.1080/15592324.2015.1117723.
- Erpen L, Devi HS, Grosser JW, Dutt M. Potential use of the DREB/ERF, MYB, NAC and WRKY transcription factors to improve abiotic and biotic stress in transgenic plants. Plant Cell Tiss Organ Cult. 2018;132(1):1–25. doi:10.1007/s11240-017-1320-6.
- El-Kereamy A, Bi YM, Ranathunge K, Beatty PH, Good AG, Rothstein SJ. The rice R2R3-MYB transcription factor OsMYB55 is involved in the tolerance to high temperature and modulates amino acid metabolism. PloS One. 2012;7(12):e52030. doi:10.1371/journal.pone.0052030.
- Meng X, Wang JR, Wang GD, Liang XQ, Li XD, Meng QW. An R2R3-MYB gene, LeAN2, positively regulated the thermo-tolerance in transgenic tomato. J Plant Physiol. 2015;175:1–8. doi:10.1016/j.jplph.2014.09.018.
- Zhao Y, Cheng X, Liu X, Wu H, Bi H, Xu H. The wheat MYB transcription factor TaMYB31 is involved in drought stress responses in arabidopsis. Front Plant Sci. 2018;9:1426. doi:10.3389/fpls.2018.01426.
- Kumar S, Stecher G, Li M, Knyaz C, Tamura K, Battistuzzi FU. MEGA X: molecular evolutionary genetics analysis across computing platforms. Mol Biol Evol. 2018;35(6):1547. doi:10.1093/molbev/msy096.
- Bailey TL, Boden M, Buske FA, Frith M, Grant CE, Clementi L, Ren J, Li WW, Noble WS. MEME SUITE: tools for motif discovery and searching. Nucleic Acids Res. 2009;37(suppl_2):W202–08. doi:10.1093/nar/gkp335.
- Lescot M, Déhais P, Thijs G, Marchal K, Moreau Y, Van de Peer Y, Rombauts S. PlantCARE, a database of plant cis-acting regulatory elements and a portal to tools for in silico analysis of promoter sequences. Nucleic Acids Res. 2002;30(1):325–27. doi:10.1093/nar/30.1.325.
- Hu B, Jin J, Guo AY, Zhang H, Luo J, Gao G. GSDS 2.0: an upgraded gene feature visualization server. Bioinformatics. 2015;31(8):1296–97. doi:10.1093/bioinformatics/btu817.
- Gasteiger E, Hoogland C, Gattiker A, Wilkins MR, Appel RD, Bairoch A. Protein identification and analysis tools on the ExPASy server. The proteomics protocols handbook. Humana press; 2005. p. 571–607.
- Yu CS, Chen YC, Lu CH, Hwang JK. Prediction of protein subcellular localization. Proteins Struct Funct Bioinf. 2006;64(3):643–51. doi:10.1002/prot.21018.
- Russell DW, Sambrook J. Molecular cloning: a laboratory manual (Vol. 1, p. 112). Cold Spring Harbor, NY: Cold Spring Harbor Laboratory; 2001.
- Horsch RB, Fry JE, Hoffmann NL, Wallroth M, Eichholtz D, Rogers SG, Fraley RT. A simple and general method for transferring genes into plants. Science. 1985;227(4691):1229–31. doi:10.1126/science.227.4691.1229.
- Murray MG, Thompson W. Rapid isolation of high molecular weight plant DNA. Nucleic Acids Res. 1980;8(19):4321–26. doi:10.1093/nar/8.19.4321.
- Du H, Yang SS, Liang Z, Feng BR, Liu L, Huang YB, Tang YX. Genome-wide analysis of the MYB transcription factor superfamily in soybean. BMC Plant Biol. 2012;12(1):1–22. doi:10.1186/1471-2229-12-106.
- Stracke R, Werber M, Weisshaar B. The R2R3-MYB gene family in Arabidopsis thaliana. Curr Opin Plant Biol. 2001;4(5):447–56. doi:10.1016/S1369-5266(00)00199-0.
- Islam M, Hasan M, Hoque H, Jewel NA, Bhuiyan M, Hossain F, Prodhan SH. Characterization of transcription factor MYB59 and expression profiling in response to low K+ and NO3− in indica rice (Oryza sativa L.). J Genet Eng Biotechnol. 2021;19(1):1–14. doi:10.1186/s43141-021-00248-6.
- Smita S, Katiyar A, Chinnusamy V, Pandey DM, Bansal KC. Transcriptional regulatory network analysis of MYB transcription factor family genes in rice. Front Plant Sci. 2015;6:1157. doi:10.3389/fpls.2015.01157.
- Li Z, Peng R, Tian Y, Han H, Xu J, Yao Q. Genome-wide identification and analysis of the MYB transcription factor superfamily in Solanum lycopersicum. Plant Cell Physiol. 2016;57(8):1657–77. doi:10.1093/pcp/pcw091.
- Ahmad Y, Haider S, Iqbal J, Abbasi BA, Yaseen T, Mahmood T. The mechanisms of genome editing technologies in crop plants. In: Principles and practices of OMICS and genome editing for crop improvement. Cham: Springer International Publishing; 2022. pp. 295–313.
- Haider S, Raza A, Iqbal J, Shaukat M, Mahmood T. Analyzing the regulatory role of heat shock transcription factors in plant heat stress tolerance: a brief appraisal. Mol Biol Rep. 2022;49(6):5771–85. doi:10.1007/s11033-022-07190-x.
- Haider S, Iqbal J, Naseer S, Yaseen T, Shaukat M, Bibi H, Mahmood T. Molecular mechanisms of plant tolerance to heat stress: current landscape and future perspectives. Plant Cell Rep. 2021;40(12):1–25. doi:10.1007/s00299-021-02696-3.
- Haider S, Iqbal J, Naseer S, Shaukat M, Abbasi BA, Yaseen T, Mahmood T. Unfolding molecular switches in plant heat stress resistance: a comprehensive review. Plant Cell Rep. 2021;41(3):1–24. doi:10.1007/s00299-021-02754-w.
- Katiyar A, Smita S, Lenka SK, Rajwanshi R, Chinnusamy V, Bansal KC. Genome-wide classification and expression analysis of MYB transcription factor families in rice and arabidopsis. BMC Genomics. 2012;13(1):1–19. doi:10.1186/1471-2164-13-544.
- Yanhui C, Xiaoyuan Y, Kun H, Meihua L, Jigang L, Zhaofeng G, Li-Jia Q. The MYB transcription factor superfamily of Arabidopsis: expression analysis and phylogenetic comparison with the rice MYB family. Plant Mol Biol. 2006;60(1):107–24. doi:10.1007/s11103-005-2910-y.