Abstract
An up-to-date overview of the recent history is given, aiming is to present a helpful working guide to the literature and at the same time introduce key systems and observational results, starting from the Sun and going toward the Galactic Center and parts of the Zona Galactica Incognita, and beyond. We start by presenting an observational view of the Milky Way’s disk plane (cartographic, dynamical, chemical cross-cut, magnetic). This included the four long spiral arms in the disk of the Milky Way galaxy, their geometry, components, velocity, their widths and internal layers as well as onion-like ordered offsets, the central galactic bars, arm tangents, arm pitch and arm shape, arm origins near the Galactic Center, and other possible players in the spiral arm, such as the magnetic field and the dark matter content. After, we present a basic analysis of some theoretical predictions from galactic arm formation: numerical simulations or analytical theories, and observations are checked against predictions from various numerical simulations and analytical (theoretical) models.
1. Introduction and scope – the galactic disk domain
Our galaxy has perhaps a trillion stars, orbiting around their common center in an ordered way. Knowing better our Galaxy observationally is our aim, as well as making basic physical explanations, and making preliminary comparisons to some predictions from numerical simulations or analytical models. Aside from irregular galaxies, interacting galaxies, and dwarf galaxies, the remainder consists of elliptical galaxies (about 1/3 of all galaxies) and spiral galaxies (about 2/3) – see [Citation1]. A good minority of spirals have a flocculent or asymmetric shape without long arms (30%), while the remaining spirals have a symmetric shape with either two long arms (10%) or else with four or six long arms (60%) – see [Citation2Citation3]. What about the Milky Way galaxy itself? Most astronomers answer that the Milky Way is approximately symmetric spiral with four long arms.
Here we concentrate mainly on the gaseous and stellar disk of our spiral galaxy the Milky Way, while briefly sketching the galaxy’s halo and nucleus. For a review of the integrated properties of the Milky Way, see [Citation4] although their Figure 11 with spiral arms dates back a decade ago to [Citation5]. For a grand review of the theories of spiral arm formation and evolution, the reader is referred to [Citation1Citation6].
To get going, we start geometrically (Section 2), employing galactic coordinates and logarithmic spiral arm equations, along with a distance scale, to bring pitch angle and interarm separation, leading to a discussion of techniques to deal adequately with some observational issues (say, how to appraise the foreground and the background and remove them, to leave only the spiral arm under study).
We then embark on the basic velocities involved for these arm features (Section 3), employing an assumed velocity dispersion, orbital circulation, and arm position in the velocity-longitude domain, leading to a discussion of techniques to deal adequately with a few observational issues (say, how to appraise dust extinction, being different at different galactic longitudes). Having both the geometry and the velocity distribution, one can enter the chemical domain (Section 4), employing different arm tracers as seen with telescopes, along different galactic longitudes and velocities, bringing statistics to deal with small error bars in measurements, leading to a discussion of techniques to deal adequately with a few observational issues (say, whether to give more weight to some chemical arm tracers, instead of the same weight).
Having reviewed the geometry, velocity and chemistry within a 5-kpc radius around the Sun, one proceeds to the interior of the Galaxy, namely within a 3-kpc radius of the Galactic Center (Section 5), starting with observational data about the start of each spiral arm there, and bringing the so-called ‘3-kpc-arm’ features in the fold, leading to a discussion of techniques to deal adequately with a few observational issues (say, the ‘blurred’ molecular ring seen by poor angular-resolution telescopes, regarded by some to be composed of separate molecular clouds as seen at specific galactic longitudes).
Some basic explanations for the spiral arms follow (Section 6), employing the predictions of numerical simulations and analytical theories, bringing in the angular rotations of galactic features (including the central bars), and comparing with observational data, leading to a discussion of techniques to deal with a few observational issues (say, the number of coexisting different density waves, if any). Comparing all theoretical predictions with all observations is outside the scope of this review.
We then look around for other, secondary features, that could perhaps be found later to have a bearing at some level on the spiral arms (Section 7), including a weak magnetic field in the galactic disk, or else a small quantity of dark matter in the spiral arms or interam, leading to a discussion of techniques to deal adequately with a few observational issues (say, the pitch angle of the stellar arm being the same or close to the pitch angle of the magnetic field in the arms).
2. Condensed survey of arm location, shape, pitch angle, interarm, distance
In this Section, the aim of this chapter is to provide the reader with an up-to-date global view of the galactic disk domain, how to trace the arms in a logarithmic fashion around the Galactic Center using observable parameters, where are the interarms and the Sun, and how to determine the distance to a galactic object.
Current maps of the Milky Way disk can differ somewhat, much like sixteenth Century maps of the Earth’s continents also differed, with unknown parts labeled ‘Terra Incognita’. Thus was defined a ‘Zona Galactica Incognita’ in the map of spiral arms for areas beyond the Galactic Center [Citation7].
2.1. Global view
The Galaxy has a central black hole, an elongated thick nuclear bulge bar, and two other thin exotic galactic bars (a 0.5-kpc-radius nuclear bar – see Table 2 in [Citation8]; a 4.2-kpc-radius long bar – see Table 3 in [Citation8]), and there is a huge disk around the galactic center (composed mainly of stars, dust, gas, mainly organized in spiral arms), and surrounded by a galactic halo. On a larger scale, the outer Milky Way seems to warp a little. Near l = 90° the mean disk is elevated to a galactic latitude b = +1° [Citation9] while in the opposite direction l = 270° the mean disk sinks to a latitude near b = −1°.
Observations of the Milky Way galaxy are tricky, as the Sun is near the middle of the galactic disk, so a view from Earth shows all the arms along the same narrow band on the sky (most arms seen at Earth are behind another arm).
The spiral structure can be determined observationally. A large number of localized observational efforts were made to observe the Milky Way in various segments of galactic longitudes. Many pieces of sky were scrutinized, one piece at a time, and their data were extracted and published. For just one example, see [Citation7].
Rather than geometric space, some have employed phase space. Some have separated the stars in the disk into phase spaces (spatially, kinematically, chemically), or into a group of older stars with high metallicity and high above the mid-plane, and a group of stars with low metallicity closer to the mid-plane [Citation10].
Observers seek to obtain measurable parameters of spiral arms. One goes to a telescope operating at a wavelength (λ) or a spectral frequency (f), and do measurements of the intensity (I), polarization state (q) of a chemical tracer (t) in an object located at a given galactic longitude (l), latitude (b) and radial velocity (v). If the object is toward a spiral arm seen tangentially, the object’s tracer (extended CO, say) would appear intense. In addition, foreground and background radiation would enter the measurements. Thus the measurements (I, f, q) of an object in arm Y (l, b, v) seen tangentially would also contain data from arm X in front along the same line of sight, as well as the interarm gas and the background disk emission.
2.1.1. Sun to Galactic Center distance
Early astronomers, up to and including Herschel in the late 1800s, thought that the Sun was at the center of our Galaxy. It took Shapley in the early twentieth Century to separate the sun’s position from the Galactic Center’s position, by about 20 kpc [Citation2]. Modern observations shows that the Sun is separated from the Galactic Center by about 8.0 kpc, give or take 0.2 kpc [Citation4Citation11Citation12]. This allows modern theories to properly present the Sun near but outside a spiral arm in the galaxy.
Table shows very recent attempts at measuring the distance of the Sun to the Galactic Center (GC), and the mean circular rotation velocity of stars near the Sun as they orbit around the GC. Each column in Table is described here: the first column refers to the distance between the Galactic Center and the Sun, in kiloparsecs. The second column refers to the kinematic circular orbital velocity of objects near the Sun around the Galactic Center, excluding peculiar velocities. The third column refers to the observational objects measured. The last column gives the appropriate references.
Table 1. Recent measures of global parameters of the Milky Way.Table Footnotea
2.1.2. Spiral arm shape
Among nearby spiral galaxies and in the Milky Way, many observed arm shapes are logarithmic. This is the spiral for which the radius R grows exponentially with the angle θ. Still, other shapes have been reported, such as an incomplete ring, an incomplete ellipse, a short line, or a complex polynomial.
The basic model of a Galaxy, with a spiral arm intensity I at galactic radius R and angle θ using a coordinate system based at the GC, and with ‘m’ different arms, a logarithmic ‘ln’ shape, an arm pitch angle ‘p’, follows from the equation [Citation13]:
(1)
(2)
and θo, A, and Ro are constants to be fitted, p > 0° with θ > 0° for an inward going arm (an arm spiraling toward a larger galactic radius ‘R’ as one proceeds to a greater angle θ, defined from the x-axis starting at the Galactic Center and going parallel to galactic longitude 90°).
Histograms for the 66 published pitch angle measurements before 2005 [Citation14] can be compared to histograms for 94 recent publications since 2015 [Citation15], and a higher peak and a narrower base can be found in the recent histogram, showing that a convergence is happening. Ditto for the published interarm measurement s, number of spiral arms m, and spiral arm shape; there is a convergence in that 12-year time period (central peak being about 3 times higher now, base width being about 3 times narrower now).
Figure shows a sketch of a fourfold-symmetry spiral arm model adopted by the author in a series of meta-studies, and this model is in approximate agreement with most recent models.
Figure 1. Sketch of the location of each spiral arm, seen at different galactic longitudes.
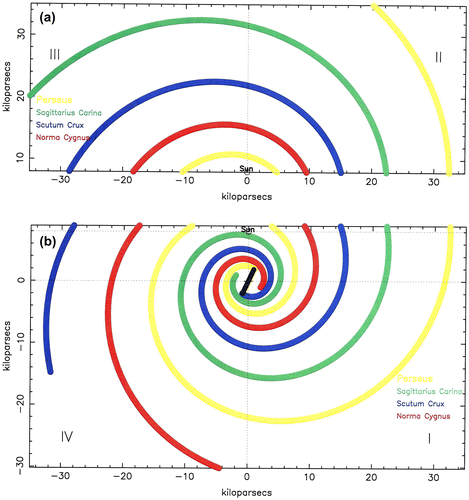
This figure gives a rough conceptual knowledge of where stellar arms are located in the Milky Way disk, separately for the outer galaxy (Figure (a)) and for the inner galaxy (Figure (b)).
Inputs are: m = 4 arms, pitch angle p = 13° inward with respect to a circle around the Galactic Center, logarithmic shape, and a distance of the Sun to the Galactic Center Rsun of 8.0 kpc. Also drawn is the short thick galactic bar, although the details are not yet agreed upon (2.1-kpc radius, at 25° from the line of sight – see Table 3 in [Citation8]). The interarm distance ‘s’, measured vertically across the Sun from the Sagittarius to the Perseus arm, is about 3 kpc.
For the spiral arms, a series of blocks, each with 15 to 20 publications, were collated and statistically analyzed. Then one needs a meta-analysis to mosaic them and to reconstruct a full view or model as seen from above – see [Citation7Citation14Citation13Citation16Citation17Citation18Citation19Citation20Citation8Citation15].
2.2. Arm pitch angle
Thus the intensity ‘I’ is maximum when γ = 0, and the arm separation ‘s’ between two adjacent arms (s = R2 – R1) at a fixed angle θ1 is [Citation13]:
(3)
The equations above define the Norma arm with θo = 0°, say. Owing to an imposed similarity conditions, each arm is offset from the preceding one by 90° in galactic longitude, so for the Perseus arm θo = −270° + θo (Norma), while θo = −180° + θo (Norma) for the Sagittarius arm, and for the Scutum arm θo = −90° + θo (Norma).
The use of Equation (3) with these values of m, s, and r1 (as the Sagittarius-GC distance) permits p to be obtained. Inside the Sun’s orbit, one can draw from the Sun a tangent to a spiral arm.
Some arms can even show two arm tangents, one in Quadrant IV (lIV) and one in Quadrant I (lI), such as for the Carina–Sagittarius arm or for the Crux–Centaurus–Scutum arm, or for the Norma arm. It can be shown [Citation20Citation21] that the following equation holds:
(4)
This last equation yields p, when the two arm tangents to the same arm (in Quadrant I and Quadrant IV) are known. The last parenthesis at right in Equation (4) is expressed in angles (π is a half circle), all in radians. It is only a function of the observed quantities lI and lIV. This method employs observed data over a wide range in galactic longitudes, and can be used separately for each arm tracer appearing in both quadrants (CO, dust, HII, etc.).
Thus for the twin-tangents to the Carina (longitude l near 284°) – Sagittarius (l near 50°) arm, the mean pitch angle p is 14.0° ± 0.4° inward, while for the twin-tangents to the Crux–Centaurus (l near 310°) – Scutum (l near 31°) arm, the mean pitch angle p is 13.3° ± 0.5° inward [Citation20]. Moving to the twin-tangents to the Norma (l near 329°) – ‘start of Norma’ (l near 20°) arm, the mean pitch p is 13.7° ± 1.4° inward, using Equation (4) above and the data in [Citation22].
Because of the moderate pitch angle value, no arm can turn around the Galactic Center more than one half of a full circle before reaching the Sun’s orbit. A very small pitch angle would lead to very many turns of an arm around the Galactic Center, before reaching the Sun’s orbit [Citation19]. Sitting near the Sun, looking towards the GC (galactic quadrants IV and I), arms with a very small pitch angle will develop many turns around the GC before reaching the Sun, and thus will display many arm tangents, yet most predicted arm tangents are never observed, and the other predicted ones do not match the observed tangents (Table 3 in [Citation19]).
Some authors have expressed the view that a spiral pattern with a variable pitch angle is possible [Citation23].
2.3. The interarm ‘s’
The Sun is located in an interarm, between the Sagittarius arm and the Perseus arm. In the more recent period from 2002 to 2016, the observed ‘s’ value, calibrated with Rsun near 8.0 kpc, turns out to be near 3.1 kpc [Citation17Citation20].
2.4. Armlet, spur, bridge, branch
Near the Sun there is a local ‘armlet’ (or ‘spur’ or ‘branch’ or ‘bridge’). Many OB stars are found between l = 60° and l = 90°, within 2.2 kpc of the Sun [Citation24]. There may be such other spurs elsewhere in the Milky Way, as there are some similar armlets seen in other nearby spiral galaxies. Olano [Citation25] used the old (600 M years) stellar groups close to the Sun (their position and velocity) to explain the local ‘armlet’ through a model of an old supercloud (with a size near 600 pc) in orbit around the Milky Way, whose center collided with the Perseus spiral arm some 100 M years ago (his Figure 5), thus braking its speed (his Figure 6) and curving its orbital path (his Figure 12), now reaching the Sun’s area in the interarm, while disintegrating over time to form the younger Gould’s Belt and the local Orion armlet.
Near the Sun, different authors have drawn the local armlet at different orientation, position, and length (see [Citation26Citation27]). A completely new interpretation of the Orion armlet is given by Lépine et al. [Citation28].
Elsewhere in the Milky Way disk, one would expect some little armlets or branches aligned roughly parallel to the arms (at a small pitch), or else some little spurs or feathers aligned roughly perpendicular to the arms (at a large pitch).
Another interarm ‘spur’ (or ‘armlet’ or ‘bridge’) was found [Citation29], covering 7° in galactic longitude (l = 32° to 39°) and 30 km/s in radial velocity (from Vlsr = +60 to +90 km/s), located in between the Scutum arm and the Sagittarius arm.
Some small armlets are found alongside large arms in nearby spiral galaxies, unrelated to any grand design spiral.
2.5. Distance
A patch of a spiral arm containing an object (a star, say), can be measured from the Sun (galactic longitude l, distance r), or else also from the Galactic Center (θ, R).
Figure shows the Galactic quadrants (I to IV) around the Sun (small circle), with galactic longitudes l = 0° to 360° (shown as thick dots in a circle).
Figure 2. Sketch of the twin coordinate system employed.
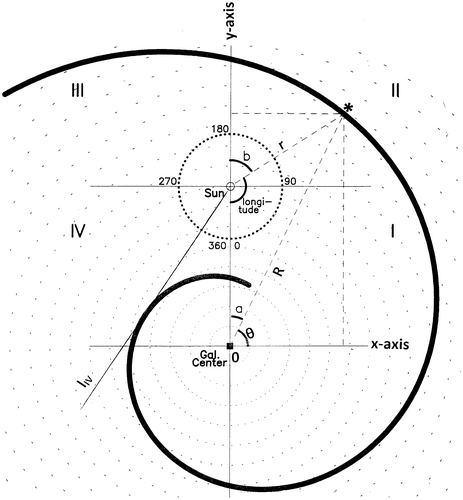
This figure gives the galactic longitude circle, galactic quadrant (I to IV) and radius r, as tied to the galactic coordinate system attached to the Galactic Center (x–y axis, radius R).
A spiral arm is shown, in a logarithmic shape, starting near the Galactic Center (square). An object (black star) on the spiral arm in Galactic quadrant II can be seen at longitude l and distance r from the Sun, with a radial velocity v (positive outward). Inside the sun’s orbit, a tangent to a spiral arm (lIV) has been drawn.
Distance determination is always done using some prior assumptions. Thus a kinematical model can be used to convert an observed radial velocity into a distance, assuming that the full measurement can be due to orbital motion around the Galactic Center. If a part of the radial velocity comes from another source, such as a supernova shell expansion or filament rotation, then the distance determination could be off. Different kinematical models exist, each using its own value of the circular orbital velocity at the solar orbit (between 200 and 250 km/s), thus adding a distance error of about 10% for a gas velocity of 225 km/s, say. Optical (UBVI) photometric calibration is reasonably known, using standard stars, color coefficients, zero-points data, DAOPHOT software; typical errors in distance can be 10%.
2.6. Summary
Several different methods have been devised to analyze the observations of a cloud, to perform a foreground and background subtraction, and to characterize only the data pertinent to that cloud. The background/foreground disk emission is fairly known; the different CO models gave slightly different output values from roughly the same observed data, especially when using different bin sizes in galactic longitudes, or different model data cutoff (63% removal, the amount of disk emission with galactic longitude – see [Citation30], or different complex disk emission model [Citation31].
Some prefer to create a three-dimensional symmetric model for the emission from the embedded disk gas, and to fit its parameters to the observed baseline intensity in a function of galactic longitude, then subtract this disk emission from the observed data, leaving the residual as the arm gas [Citation31]. Others use additional data such as the radial velocity to create a longitude-velocity plot, and to circumscribe the cloud there (within such permitted extent in velocity and permitted extent in longitude), then use that information to best fit that cloud in the intensity vs. longitude vs. distance diagram [Citation30]. Finally, a simple subtraction method is to use a second-order polynomial for the background / foreground disk emission on the plot of intensity vs. galactic longitude (which is subtracted), and followed using multiple Gaussians for the lines in the residual [Citation32]; this method assumed universal dust behavior (neglecting the arbitrariness in the amount of mid-infrared dust extinction as a function of different galactic longitude – see [Citation33]). Each method comes with a different number of assumptions (disk shape, velocity model, polynomial baseline, dust absorption, etc.), and with a different number of model variables to fit; the sum of the assumptions and model variables increases the final error bar.
3. Brief survey of arm kinematics and velocity
In this section, we provide the reader with a mid-scale (orbital) and a small-scale (turbulent) view of gas motions.
3.1. Small scale
Non-circular, localized gas motions can exist of the order of 10 km/s. These motions include interstellar shocks (from supernovae or encounters with a nonlinear density wave), expansion of superbubble shells, non-circular (elliptical) gas orbits around the Galactic Center, localized streaming motions from spiral density waves, infall of HI neutral gas from the halo, etc. [Citation34Citation35].
3.2. Mid scale
The stars and gas follow an orbit around the Galactic Center, obeying the gravitational attraction from the center of the Galaxy – much like the Earth and planets orbit the Sun. The gas and Sun will enter the slower moving arm (inner side), then exits the arm (outer side) some time later. Specifically, one assumes here that the Sun is interior to the corotation radius – a likely assumption.
Observations show a flat circular velocity (same at all radial distance from the Galactic Center). Adopting a flat circular velocity of 220–230 km/s above a minimum galactic radius of 2 kpc, one gets the basic equation for the radial velocity Vr of an object at galactic radius r and galactic longitude l, as seen from the Sun [Citation35Citation36]:
(5)
with (ω – ωo) being the difference in angular rotation rate at r and at the Sun.
Figure shows the radial velocity as a function of galactic longitude. The four-arm model in Figure has a counterpart in this velocity-longitude diagram. Thus the radial velocity derived from the model of Figure are shown in Figure .
Figure 3. Sketch of the radial velocity of each spiral arm, seen at different galactic longitudes (using a flat circular velocity of 220 km/s).
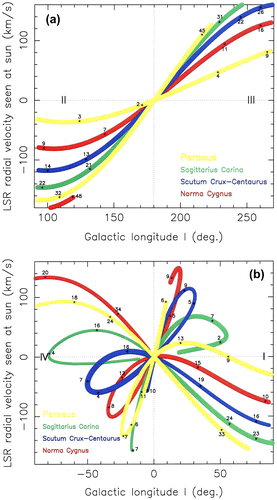
For a chosen portion of an arm (colored), this figure shows the radial velocity (vertical axis) of the gas in that arm as a function of the galactic longitude (horizontal axis) of the arm portion.
Thus with a spectroscopic instrument mounted on a telescope on Earth pointed at a specific galactic longitude (in Figure ), a gas or star’s observed radial velocity can be measured, and one can infer its specific spiral arm (in Figure ), and a distance from the Sun can be inferred for that arm at that longitude (in Figure ).
3.3. Comparisons with the observations
The four-arm model shows good agreement, in each Galactic Quadrant (GQ). Some of the comparisons below fall in a narrow range from the Galactic Center, and thus may not support any model employing circular orbits and giving near-zero radial velocities.
GQ I. Pandian & Goldsmith [Citation37] displayed the velocity of methanol masers in GQ I, and there is a good match in the four-arm model [Citation16]. Stark & Lee [Citation38] displayed the major 13CO J = 1-0 clouds for the Sagittarius arm (their C arm), the Scutum arm (their D arm), the Cygnus arm (their F arm), the Perseus arm (near l = 30° – their A arm; near l = 80° – their B arm), for which there is a good match to the model [Citation16]. Zucker et al. [Citation39] also found a good fit to this model [Citation16] with the 12CO data J = 1-0 in the longitude ranges l = 0°–8° (their Figure 29), l = 8°–16° (their Figure 26), l = 16°–24° (their Figure 23), l = 24°–30° (their Figure 19).
GQ II. Stark & Lee [Citation38] displayed the major 13CO J = 1-0 clouds for the Cygnus arm (their F arm), being a good match to the four-arm model [Citation16]. Dobbs et al. [Citation40] displayed the observed 12CO J = 1-0 clouds for the Perseus arm and the Cygnus arm, for which there is a good match to this four-arm model [Citation16].
GQ III. Yang et al. [Citation41] displayed 12CO J = 1-0 gas for the Perseus and Cygnus arm, for which there is a good match to this model [Citation16].
GQ IV. Brown et al. [Citation42] compared the HI absorption and radio recombination line velocities from 252 observed HII regions in GQ IV, and found most of them aligned with the four-arm model. Kothes and Dougherty [Citation43] shows the HI profile in GQ IV, and their Figure 1 does compare well with this model for the line of sight at l = 340°, with similar intersections with the spiral arm encountered [Citation16]. Also, their highest velocity HI gas (their Figure 3) is at this model velocity of −140 km/s. Finally, they observed that the HI gas peaks between −140 and −100 km/s due to a ‘pile-up in velocity space’, with gas distributed over a large distance interval due to seeing several arms in that longitude, as found in this model. Zucker et al. [Citation39] also found a good fit to this model [Citation16] with the 12CO data J = 1-0 in the Scutum arm, in the longitude ranges l = 330°–338° (their Figure 37), l = 353°–360° (their Figure 33).
3.4. Discussion
There was an issue on the physical reality of the ‘fingers’ pointing at the Sun. In a supernova explosion, HI gas affected by the supernova will have a large front component and a large back component in radial velocity, which, if taken as orbital motion in a kinematic distance model, would be erroneously translated as HI gas at different distances along the line of sight, resulting in a ‘finger’ pointing at the Sun. At radio wavelengths, HI gas is measured in galactic longitude and radial velocity, and if a simple orbital velocity model is used to get its ‘kinematical’ distance, it will result in ‘features elongated along the line of sight’, like ‘fingers’ pointed at the Sun (HI in [Citation44], their Figure 4(a)). Others studied more complex velocity models to get a more realistic distance from the Sun, using velocity deviations or jumps. Without these jumps, they reconstructed numerically some ‘density fingers’ pointing to the Sun [Citation45Citation46].
At optical and near-infrared wavelengths, isochrone fits in the UBVRI method and variable reddening and absorption laws should differ for each galactic longitude [Citation33Citation47], due to variable dust density and dust size with increasing and decreasing galactic longitude, correlated with spiral arms. Using instead ‘universal’ dust laws (invariant with galactic longitude), then stars at the same distance, but at slightly different longitudes, could appear to go up or down in distance, along their specifically reddened line of sight at different galactic longitudes. They are seen as pseudo ‘chains of O-B starts elongated along the line of sight’ [Citation48], visible in their Figure 4 at galactic longitudes 78°,174°, 189°, and 290°, and in their Figure 6 at longitudes 134°, 189°, 290°, 342°. These are seen as a pseudo line of open star clusters, near l = 242° and near l = 258° [Citation49Citation50]. Other spiral galaxies have been observed, and spiral galaxies have never shown such ‘fingers’ pointing at a specific star in a specific location inside a galaxy.
Are there velocity jumps not due to distance offsets? Velusamy et al. [Citation51] used the kinematical orbital method, with the circular velocity of 220 km/s, to obtain the linear distances (their Table 1c) and linear offsets relative to the mid-arm (their Table 2a). They obtained maps in [CII] at λ = 158 μm with Herschel HIFI as restored to an effective beam of 80′′ and a resolution of 2 km/s. They first looked toward the tangents to the spiral arm of Norma (near 328° for the CO peak intensity) and toward the beginning of the Perseus arm (near 337° for the CO peak intensity). These longitudes represent the cold CO (1-0) peak intensity lanes [Citation52]. Thus toward the cold CO arm tangents, they found two [CII] peaks in radial velocity, separated by about 10 km/s (their Figures 7(b) and 8(b)). They attributed their two [CII] peaks to one from a warm medium (compressed), and one from a colder medium (molecular). They identified the compressed medium toward the mid-arm as the ‘inner edge’ of the spiral arm (their Figure 11), although the observed dust lanes are farther away in longitudes, at an ‘innermost arm edge’ [Citation52].
4. The arm width, composition, structures in parallel lanes (physical offsets)
When moving a telescope in galactic longitudes with a filter to observe a single tracer at a time (CO, dust, masers, etc.), one can find a peak intensity at a longitude where the arm is seen tangentially. The reader could then have a clear view of the onion-like separation of these arm tracers in galactic longitudes, as well as the reversal of these onion-like tracers as one crosses the Galactic Meridian (located at a galactic longitude of zero).
The aim here is to provide the observed mean offset in linear width of a tracer t with respect to tracer 12CO in a typical spiral arm, as well as individual offsets s in each individual arm. Few numerical or analytical theoretical predictions for the Milky Way conform to this offset reversal across the Galactic Meridian.
We can use different tracers to get the galactic longitude where an arm is seen tangentially.
4.1. Arm tangents
There is a recent published Catalog of tangents of arm tracers (arms seen tangentially, using different chemical tracers) culled from the literature, and various statistics can ensue [Citation22]. There are many wavelengths represented in this Catalog of tangents to the spiral arms, as seen in gamma-ray, optical, near and far infrared, submillimeter, and radio. The Catalog has a master table of the mean longitude for each arm and for each tracer (Table ), followed by individual tables (one table for each arm tracer) listing individual observational data for each arm [Citation22]. In its first publication, this Catalog had 43 entries (Table 3 in [Citation18]). In the most recent publication, it grew to 215 entries (Tables 3–10 in [Citation22]).
Uncertainties. For the 12CO tangent to the Crux–Centaurus arm, the mean of 309.5° has an r.m.s. of 1.0° and s.d.m. of 0.3° (Table 5 in [Citation22]). For the dust-870 -microns tangent to the Carina–Centaurus arm, the mean of 311.4° has an r.m.s. of 0.5° and a s.d.m. of 0.35° [Table 9 in [Citation22]). The separation (12CO vs. dust) is 1.9° and the combined s.d.m. is 0.46°. The dust tangent is always closest to the GC than the CO tangent. For the 12CO tangent to the Sagittarius arm, the mean of 50.5° has an r.m.s. of 0.9° and s.d.m. of 0.5° (Table 5 in [Citation22]). For the dust-870 -microns tangent to the Sagittarius arm, the mean of 49.1° has an r.m.s. of 0.2° and a s.d.m. of 0.1° [Table 9 in [Citation22]). The separation (12CO vs. dust) is 1.4° and the combined s.d.m. is 0.5°. The dust tangent is always closest to the GC than the CO tangent. Combining all the arms together, the angular separation (12CO vs. dust) is 3.2° with an r.m.s. of 0.68° and a s.d.m. of 0.3° (Table 1 in [Citation22]) giving a signal/noise ratio of 11, while the linear separation (12CO vs. dust) is 315 pc with an r.m.s. of 64 pc and a s.d.m. of 26 pc (Table 1 in [Citation22]) giving a signal/noise ratio of 12.
Using the dust tracer, the galactic longitude of each arm is always closer to the direction of the GC than for the CO tracer for that arm. This is true for ALL spiral arms (Table 1 in [Citation22]). Using the galactic quadrants, the hot dust is at a higher galactic longitude than the CO before (at left of) the Sun-Galactic Center line (in galactic quadrant IV), but the hot dust is at a smaller galactic longitude than the CO after (at right of) the Sun-Galactic Center line (in galactic quadrant I).
This ‘reversal in ordering’ across the Sun-GC line is predicted by the shocked physics of density waves. Thus the angular distance of a tracer (dust, say) from the arm center (CO) is positive toward the arm’s inner edge (toward the Galactic Center), and negative otherwise [Citation18Citation52]. This observed reversal, around the Sun-GC line, is the first concrete, necessary and sufficient proof for the basic galactic density wave model with shocks.
It is important to use statistics in order to get the best galactic longitude of an arm tangent, from a given tracer, hence the presence of separate tables with their means and errors. Basic statistics with median and means, weights and error bars, balance out positive vs. negative biases. Biases can arise notably from different model assumptions, differing procedures, differing averaging bin sizes, or different radial velocity range, or a smaller range in galactic longitudes (affected by the local emission from an object such as a supernova remnant), or different input data cutoffs.
To avoid overlapping when comparing different arms, it is important to ‘anchor’ all tracers to a common system; so one defines one of them (broad emission from 12CO 1-0) as the ‘zero’ of linear separation from the mid-arm. There are enough measurements in the CO catalog [Citation22] to pinpoint accurately where the peak CO is located.
The motivation for choosing the galactic longitude of the CO peak intensity (observed at a low-angular resolution of 8’) as the center of a spiral arm was explained [Citation18] thus: CO is prominent in intensity and well mixed with cold molecules, so a CO telescope with a broad angular view can easily find the tangent to a spiral arm having molecules, with a very small uncertainty in galactic longitude. This CO tracer is the low-excitation J = 1-0, low temperature (10 K), low-angular resolution (8 arc-min), narrow-line width (2 km/s), 115 GHz data from the Columbia survey, as integrated over a radial velocity range associated with a spiral arm. Some of the earliest works employed 12CO J = 1-0 as tracer to locate the arm tangent [Citation53Citation54Citation55].
In our Galaxy, another good arm tracer is 13CO J = 1-0. There is an absence of bright massive molecular clouds in the interarms (not observed in 13CO 1-0), as evidenced in Figures 12 and 13 in Roman-Duval et al. [Citation56]. The confinement of massive, bright molecular clouds to spiral arms implies that these clouds are formed in the arms, and have short lives (less than 10 M years), to prevent them from spreading in the interarms. Roman-Duval et al. [Citation57] defined a ‘diffuse’ CO component as CO gas detected in the 12CO J = 1-0 line but showing no emission in the 13CO J = 12–0 line (their Section 1, Figure 9 and Table 5c), spread over the disk, amounting to about 25% by mass of the total molecular gas mass.
Thus a first a statistical analysis gets the mean of galactic longitude values for the different entries for a tracer ‘t’ over each arm, and second a statistical analysis gets the mean offset in galactic longitude of the different means of tracer ‘t’ for all arms – this ‘linear sequence’ aims to bring the errors down.
Figure (a) shows the mean positions (from a catalog of arm tangents) for the mean CO tracer and the hot dust tracer, as a function of galactic longitude [Citation22]. Adding 360° to the negative galactic longitudes (at left) would give a positive longitude value. One can readily see that the hot dust tracer of an arm is closer to the Galactic center than the mean CO tracer. Note the tracer reversal across the galactic center: at right (l > 0°) the hot dust is at a smaller galactic longitude than the CO, while at left (l < 0°) the hot dust is at a greater galactic longitude than the CO.
Figure 4. Display of spiral arm tracers.
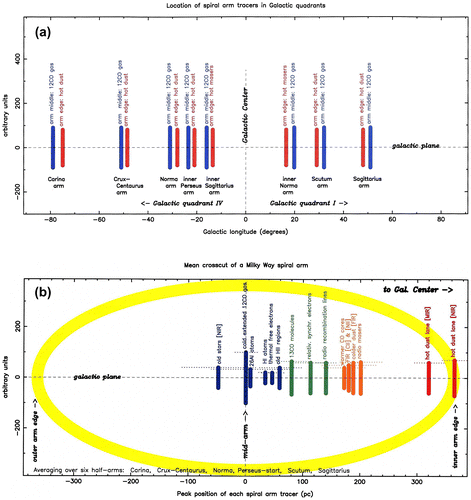
Table shows the statistics on the linear separations of each tracer, with 18 entries averaged over 6 half-arms. It confirms earlier efforts [Citation18Citation22Citation52]. In Table , the first four arms are in Galactic quadrant IV, and the hot dust is separated from CO by a positive value toward the GC; the last row shows its mean separation from CO to be between 200 and 400 pc toward the GC. In Table , the last two arms are in Galactic quadrant I, and the hot dust is separated from CO by a positive value toward the GC; the last row shows its mean separation from CO to be between 300 and 400 pc toward the GC. Here, we took account of the reversal of hot dust and CO between galactic quadrants IV and I.
Table 2. Linear separation (S) of each arm tracer from 12CO, in each spiral arm.Table Footnotea
In Table , the method to obtain the linear separation S is obtained simply using the angular separation in galactic longitude between the CO arm tangent and the other tracer arm tangent in Column 1, then by multiplying this angular separation by the distance between the Sun and the arm tangent. The mean separation S and its standard deviation of the mean (s.d.m.) are given in columns 8 and 9.
The data for each arm tracer are taken from the publication as published in the refereed literature [Citation22], and include:
• | radio observations of HI atom at a wavelength of 21 cm, radio observations of NH3, 13 CO, 12CO molecules, radio observations of recombination lines at 1.4 GHz and of relativistic synchrotron emission at 408 MHz, radio observations of masers in starforming regions, and radio centrimetric observations of thermal free electron in the interstellar medium through pulsar dispersion measure at centimetric wavelengths, | ||||
• | Far Infrared observations of cold dust, | ||||
• | Mid-Infrared observations of [CII] and [NII] lines, | ||||
• | Near Infrared observations of stars and of hot dust, | ||||
• | Optical and radio observations of HII ionized regions, | ||||
• | Gamma-ray observations at 1.8 MeV of the 26Al (aluminum) atom. |
Figure (b) shows the onion-like disposition of the different arm tracers inside a spiral arm, with the Galactic Center being the onion’s center. The innermost onion sheet (red) represents the hot dust lane, due to the shock when the gas enters the inner edge of a spiral arm. The outermost onion sheet (blue) represents the cold, extended CO gas in the arm middle. The arm tangent is about 6 kpc from the Sun – see Table 1 in [Citation22], not nearby. Hence a 2.5° separation between CO and dust converts to 260 pc – see Table 1 in [Citation22]. Inversely, a 200 pc separation at 6 kpc converts to 0.033 radian or 1.9°.
The peak of the old HII regions is at about the same place as the peak of the large angular scale 12CO 1-0 gas emission, near the arm center. Visible old stars fill all the arms. Following Figure 2 in [Citation52], one divides the arm tracers into 4 zones:
• | A blue zone is drawn for arm tracers at or very near the arm center, or mid-arm, around 0 pc, which include the cold 12CO J = 1-0 molecular gas as observed with a big half-power beamwidth of 8’. It encompasses tracers who fall nearby, and have a linear separation (from 12CO) smaller than their error (1 s.d.m.). Examples are the peak of the HII region complexes, the peak of old stars seen in NIR, and the peak of thermal electron, etc. This zone contains older evolved stars and older visible HII regions. | ||||
• | A green zone is drawn for arm tracers further on, at around 100 pc away from the mid-arm, with arm tracers having a linear separation of that order, larger than their error (1 s.d.m.). Examples are the peak synchrotron radiation and radio recombination lines near H166α at 1.4 GHz. This zone might contain radio emission from young supernovae and young stars. | ||||
• | An orange zone is drawn for arm tracers farther out, around 200 pc from the mid-arm, with arm tracers having a linear separation of that order, larger than their error (1 s.d.m.). Examples are the colder FIR dust and the masers. This zone might contain very young star-forming regions. | ||||
• | A red zone is drawn for arm tracers way out, around 300 pc from the mid-arm, close to the arm’s edge facing the direction to the Galactic Center (inner arm edge). Examples are the hot NIR and MIR dust emission. This zone might contain extended dust shocked by the galactic density wave. |
The only theory that predicts such an offset for different tracers is the galactic density wave theory [Citation58Citation59]. The arm cross-cuts are made between the Sun and the GC, hence at typical radial galactic distance from the GC of about 5 kpc. Inside corotation, with the orbiting gas going faster than the spiral pattern, the density wave theory predicts some offsets as follow: the hot dust lane (inner edge), the gas maximum and HII regions (arm center). Not all offsets shown in Figure 4 have been predicted by the density wave theory.
4.2. Filaments
What is the exact location of the giant (100 pc) molecular filaments [GMF] – are they mostly in the interarm, or in the spiral arm ? If in the arm, are they in the dust lane, or do they fit the middle of the arm (spine)? Several narrow, long (50 pc to 230 pc) giant molecular filaments are known. Some authors place the long molecular filaments in the middle (center) of an arm. Thus in GQ IV, the 400-pc ‘Nessie’ GMF appears to be a ‘bone’ in the Scutum arm at 338° and −38 km/s (one filament in Figure 4 in [Citation60], although along that longitude the radial velocity changes very rapidly with distance from the Sun (20 km/s over 1 kpc), making the true distance unclear if some of the filament velocity is not entirely due to galactic rotation. Thus for a 10 km/s random velocity, there is a 0.5 kpc distance move, making Nessie an interarm object. Also, Zucker et al. [Citation39] found some filaments near the mid-arm structure. Here one must know exactly where is the inner arm edge and the mid-arm, as the two are about 300 pc apart (Figure 4).
Yet some authors place the filaments at the inner edge of an arm. Wang et al. [Citation61] located many cold filaments and found 1/4 of them to be close to an arm center. Ragan et al. [Citation62] found the kinematical distance in a plot of radial velocity vs. galactic longitude for many filaments to be in the interarm regions (seven filaments in their Figure 4). Abreu-Vicente et al. [Citation63] position about half of the filaments in the interarms (8 out of 17 in their Figure 1). For their GMF, their Table 2 and Section 3.1.1 gave different kinematic and extinction distances (about 3.4 kpc vs. about 4.9 kpc), or a difference about three times that of a typical arm width (≈ 0.6 kpc), making it difficult to say if a Giant Molecular Filament is located inside or outside an arm. Wang et al. [Citation61] defined the location of a spiral arm in the radial velocity vs. galactic longitude space (their Figures 4(a) and (b)) and claimed that 27% of their long (100pc) filaments delineated the center of spiral arms (within an arm width of 10 km/s), and that a further 20% were interarm features (or spurs). Only more observations, with a more precise distance determination, could tell us exactly where a giant molecular filament fits inside or outside a spiral arm.
Theory-wise, numerical simulations, including self-gravity and feedback, showed how easy it is to reproduce giant molecular filaments (40–250 pc in length) in the interarm regions through galactic shear, and these GMF appear to be situated either in the interarm or else in the process of joining an arm – see [Citation64].
4.3. Discussion
There is an issue about fitting locally and projecting globally – or how far can you extrapolate? All approaches use limited observations plus assumptions to derive a model. Many model fits to the spiral arms have only employed nearby tracers, such as optically visible open star clusters in the dusty galactic disk, hence within 3 kpc of the Sun’s location. Without observing an arm tracer at large distances from the Sun, the fitted model parameters have large error bars, and their projection over the whole galactic disk is challenging [Citation65Citation66].
There is an issue about the existence or not of two superior arms. Is Sagittarius major or minor? Are there some arms more intense than others? The radio observations of [Citation67] found the Sagittarius arm to be prominent, strongly detected and clearly defined, traced by massive star formation; they concluded that the Sagittarius arm is a major feature of the Galaxy, rather than a minor arm. The near-infrared range is biased by massive extinction of light by dust, so these data must be calibrated as a function of longitude [Citation68Citation69]. Some observers prefer the near-infrared range of wavelengths; they concluded that the Sagittarius arm is not a true arm, nor is the Norma arm a true arm [Citation70Citation71]. Once one does a proper dust correction, then other arms show up. Taking all wavelengths into account, the crosscut of alternating spiral arms shows all arm widths to be nearly equal [Citation18]. A sizable minority of nearby spiral galaxies possess a four-arm symmetry along with a bar, such as NGC 5970, NGC 0180, NGC 3346, NGC 6744.
There is an issue about the possible merging of the Perseus and Sagittarius arm. Some numerical models use only two spiral arms, along with bifurcations and mergings. Siebert et al. [Citation66] derived a Sagittarius arm merging with the Perseus arm, at a location some 3 kpc away from the Sun near galactic l = 90°. The reality is that most spiral galaxies show a flat pitch angle with galactic radius [Citation72], hence if all arms start near the GC then merging of two major arms further away is not possible.
5. Where is the location of the start of each of the four spiral arms, near the Galactic Center?
In this section, the goal of this chapter is to provide the reader with the observed start of the Perseus arm near the Galactic Center, as well as that of the Scutum arm down there, and the recently observed start of the Sagittarius arm, and that of the Norma arm.
5.1. The start of the four spiral arms
The observed ‘start of Perseus’ arm, or Perseus arm origin, is already well documented, between galactic longitudes −23° (= 337°) for the 12CO 1-0 peak intensity and −20° (= 340°) for the hot dust lane [Citation16Citation73Citation74].
Similarly, the observed beginning of the Scutum arm is well documented, between galactic longitudes +26° (dust lane and methanol masers) and + 33° for the 12CO 1-0 peak intensity [Citation31Citation52Citation73].
Concerning the other two spiral arms, an early attempt by Green et al. [Citation75] using methanol masers (but not 12CO) suggested a link of the arms to the ends of the long thin bar (as known then). Also, the ends of the long thin bar nowadays cross some spiral arms, without apparent arm disruption.
One can accurately model spiral arms (see basic equations in [Citation13]), using as input the best observations of arm tangents between the Sun and the Galactic Centre (GC), in a galactic radial range from 3 to 8 kpc, and also using the best observed spiral pitch angle value. The resulting output should be the best prediction for the start of these four arms near the GC (arm origins between 2 and 3 kpc). One finds that the tangent to the ‘start of the Sagittarius’ spiral arm (arm middle) is at l = −17° ± 0.5°, (= 343°) while the tangent to the ‘start of the Norma’ spiral arm (arm middle) is at l = +20° ± 0.5°. The origins of these four arms are found to be close to 2.2 kpc from the Galactic Center [Citation8].
Figure is a representation of the many additional features occurring near the Galactic Center [Citation8]. The central galactic bulge (diamond, oriented at 25° to the Sun-Galactic Center line), and the blurred molecular ring near 3.7 kpc are also sketched. The short outflows (orange) represent the small +3 kpc ‘arm’ (a misname short feature) and the small –3 kpc ‘arm’ (a misname short feature).
Figure 5. Sketch of the starts of the 4 spiral arms.
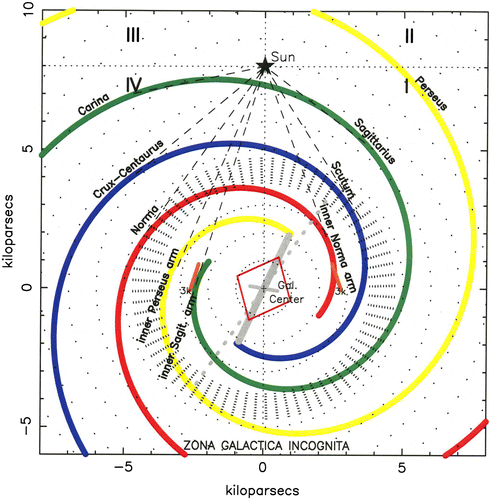
Recent observations of the Milky Way’s central area shows three possible bars there, somewhat overlapping in space (the bulge bar, the nuclear bar, and the thin long bar).
Much of this describes recent observations and models that are not fully agreed upon and that are sometimes in conflict with each other.
In this figure, as seen from the Sun, one first finds the tangent to each arm, near their origin where the arm turns and becomes tangent to the line of sight from the Sun, and then one looks for observations of chemical tracers for this arm (and their offsets). Observations of these chemical tracers showed that the tracer ordering ends exactly where the arm tangents are extrapolated from 12CO, giving an observed ‘confluence’ (between the tangent point and the ordered tracers: hot dust, masers, stars, etc.).
In this figure, the observations showed, very near the arm tangents to two spiral arms, filaments going at high speeds (a misnamed ‘3-kpc arms’ feature) which are potentially related to the spiral arms of Norma (at l = +20°) and of Sagittarius (at l = −17°). Misnamed ‘3-kpc arm’ features far from the Galactic Center (at l > +13° or at l < 347°) may be shocks associated with long spiral arms [Citation76].
5.2. Individual vs. global pitch angles
Table shows for the Milky Way that each arm has a similar pitch angle, if one excludes the innermost and outermost regions (longitude 337° arm, and Cygnus + 1 arm).
Table 3. Observed individual pitch angle (p, in degrees, negative inward), for each spiral arm in the Milky Way galaxy.Table Footnotea
The data in Table for each arm are taken from the literature, using either the parallax method, the kinematic method, the luminosity-distance method, or the tangential method using the arms on both side of the sun-galactic center line. Most of the data used come from the parallax method employing masers.
Comparing each arm to the next arm, the last row shows that the mean individual pitch angle is often the same, from arm to arm, within the relative observational standard errors (about 3°).
Elsewhere, in many nearby disk galaxies, the pitch angle appears roughly flat over 10 kpc, save for localized deviations with an amplitude near 20° [Citation72].
5.3. Central bars
There is a thick bulge bar (about 2.1-kpc radius and at about 20° to the line-of-sight – see Table 3 in [Citation8]). The existence and nature of central bar(s) is an area of active work and debate. There are as well other ‘putative’ bars (one is a 4.2-kpc-radius bar at about 40° to the line-of-sight – see Table 3 in [Citation8]; and one is a 0.5-kpc-radius bar almost perpendicular to the line-of-sight – see Table 2 in [Citation8]).
Is the long bar a composite of segments, as it appears to cross four spiral arms (Figure ) without any observable effect? Some groups view the long bar as a composite: a bar and an S-shaped trail [Citation77], or else a physical link between the outer edges of the short bulge bar and those of the long bar, appearing as ‘leading ends’ [Citation78], while Monari et al. [Citation79] have argued for the long bar being a loosely wound spiral, not a straight structure.
5.4. Discussion
As mentioned in the Introduction, the molecular ring is a rather blurred composite of various segments, and is regarded by some as not a physical barrier to the arms – see the discussion in [Citation16]. With a higher angular resolution, the molecules were found in specific areas, not in a whole ring. Thus once one removes the origins of each of four arms in that radial range, then very little gas appears to be left there in velocity space [Citation80].
There is an issue about the location of the so-called misnamed ‘3-kpc arm’ features. They perhaps should be relabeled ‘3-kpc-streams’. Some have been assigned close to the well-known ‘start of the Perseus’ arm, while others have been assigned to the well-known tangents to the Scutum arm. Others found the so-called ‘3-kpc arm’ feature at various places, over a wide area near the Galactic Center between 12° < l < 24° and −12° < l < −21° [Citation8]. These ‘3-kpc-streams’ exist observationally, yet their ages and stability are unknown. They could be due to temporary turbulence, as sometimes predicted in some models [Citation76].
6. Some explanations for the arm’s orbital dynamics, formation, maintenance, and waves
In this section, we provide the reader with basic observables for angular rotation of various galactic features, and an attempt at basic explanations of the observed spiral arms through the numerical or analytical theories for spiral arms.
6.1. Angular rotation and orbits
Starting from galactic radius R and orbital velocity V, one can obtain angular rotation ω and orbital period P, as follows:
(6)
(7)
where 1 M years = 3.16 × 1013 s and: 1 pc = 3.09 × 1013 km. Note the approximate sign (≈), as this is not a strict equality.
Table shows the results. The first column indicates the feature whose distance (column 2) angular rotation (column 4), circular velocity (column 6) and orbital period (column 8) are given, along with their respective references. (columns 3, 5, 7, 9).
Table 4. Recent angular rotation values of some Milky Way galactic features.
The ‘spiral pattern’ rows refer to the ‘pattern speed of the spiral density wave’ at the Sun’s location (set at 8.0 kpc), while various published models [Citation81Citation82Citation83Citation84Citation85] found it an angular velocity value (column 4), from which equation 6 here yielded its orbital speed at this radius (velocity in column 6), while equation 7 yielded an orbital period (column 8). The word ‘circular’ is mathematical, as the model-dependent pattern orbit may not be circular. This places the Sun just inside the corotation radius.
The ‘bulge bar’ rows refer to the orbital speed at the end of the short thick bar (set at a radius of 2.1 kpc [Citation8]) straddling the stellar bulge, while various published models [Citation86Citation87] found it an angular velocity value (column 4), from which equation 6 here yielded its orbital speed at this radius (velocity in column 6), while equation 7 yielded an orbital period (column 8).
The word ‘circular’ is mathematical, as the model-dependent bar orbit may not be circular.
For the stars and gas near the Sun in the Local Standard of Rest (LSR), one can use the galactic distance of the Sun to the Galactic Center Rsun (8.0 kpc) and orbital velocity Vlsr to compute here ω and P for the stars and gas.
For the hot gaseous halo around the Milky Way (within 50 kpc) at a temperature near 2 × 106 K, one can use at a radius of 8.0 kpc the observed rotation velocity 183 ± 41 km/s around the Galactic Center [Citation88].
For the short boxy bulge bar at the Galactic Center, one can use the radius R = 2.1 kpc for the boxy bulge bar. One takes for ω the value predicted by the bar model.
Are there links between these ω values?
In the density wave theory, the LSR’s angular value near the Sun ought to be slightly larger than the value for the spiral pattern, in order to provide a shock upon entering an arm (before co-rotation). Orbital streamlines around the Galactic Center predict that the orbiting gas will slow down as they approach a spiral arm, and speed up afterwards when leaving the arm.
It is noted that the ω value for the hot halo gas is close to but slightly smaller than the ω value for the spiral pattern, although there is no obvious direct explanation. Hodges-Kluck et al. [Citation88] suggested that outflows from the disk into the halo may follow galactic rotation but eventually lag behind the gas in the disk. Expectations for the bulge bar vary. Englmaier and Gerhard [Citation36] and others argued that the angular rotation of the spiral pattern (≈ 25 km/s/kpc) ought to be similar to the angular rotation of the short boxy bulge bar (≈ 35 km/s/kpc), if the two features are attached. Bissantz et al. [Citation89] used smoothed-particles hydrodynamics (SPH) to follow gas flows orbiting around the Galactic Center. Their models indicated the need for different angular speeds, the one for the bar differing from one for the spiral pattern, and sufficiently different to avoid a dissolution of the arms.
6.2. Formation and maintenance and waves
How do arms form in the Milky Way? There are a plethora of theories and simulations to generate spiral arms. Their predictions should match a few observed arm parameters: linear separation of different arm tracers from 12CO (over 350 pc), all arm tracers to one side of 12CO, the number of arms (4), average pitch angle (13° inward), arm shape (logarithmic) and azimuthal arm spacing (equal), to first order.
No small improvements in distant-based (>30 kpc), tidal theories for the arm formation could generate this arm number (4), and the observed tracer offsets (red, orange, green, blue in Figure (b)). No small improvements in local-based (< 3 kpc), individual random perturbing instabilities could show this equal arm ordering and tracer offsets. Perhaps additions in galactic-based (≈ 10 kpc) wave theories and simulations might be made to explain the ordered offsets for different chemical tracers in four equidistant spiral arms.
6.2.1. Versions of a globally based theory and simulations
Theories having shocks can create offsets among arm tracers. The quasi-stationary density-wave theory can produce several arms, an orbital trajectory of the gas and stars going through spiral arms, and a linear separation between arm tracers as occasioned by a shocked gas and dust entering the arm from the inner edge, then some of it coalescing to form stars there (dust lane), progressing through the arm and exiting on the arm’s outer edge [Citation59]. In Figure , one can assume that the corotation radius is above 9 kpc. Beyond corotation, with the orbiting gas slower than the spiral pattern, the wave theory predicts a reversal of the tracer offsets with respect to the arm center.
In the non-linear density wave, the shock position and the gas density maximum (at mid-arm) are separated by about 12 Myrs [Citation59], or 306 pc for a gas velocity difference of 25 km/s [Citation1]; this separation between the shocked dust lane and the mid-arm is similar to the observed arm’s halfwidth of 360 pc [Citation52]. The theoretical model with four arms of [Citation90] has the gas density maximum (potential minimum, broad CO at mid-arm) separated from the shock lane (hot dust).
The density wave proposed that the peak of each arm tracer is between the inner edge of an arm and the mid-arm, excepting the old stars being distributed all over the arm. All arm tracers are to be found between these two boundaries, none outside; this is exactly what is observed in arm tangent catalogs [Citation52].
The density wave predicts that the inner arm edge with the dusty shock lane shall be at a higher galactic longitude than the mid-arm in Quadrant IV, but at a lower galactic longitude than the mid-arm in Quadrant I – as observed in arm-tangent catalogues [Citation52].
Renaud et al. [Citation91] used hydrodynamical simulations at a high resolution of 0.05pc, with stellar feedback, a bar, and many arms, finding a global molecular structure in the Milky Way disk. Their arms displayed a gradient of gas density and velocity across a spiral arm (their Figure 13), and they found 3 or 4 spiral arms close to the bar (their Figure 17). They also find many ‘beads on a string’ with regular spacing along the arm.
6.2.2. Versions of locally based theories
The transient-recurrent ‘dynamic spiral’ reconnection theory [Citation1] has arms breaking and reconnecting, and a high pitch angle between 20° and 40° [Citation1], unlike the mid 13° pitch as found generally in the Milky Way. Baba et al. [Citation92] proposed a dynamic spiral theory, in which the gas does not flow through the spiral arm (contrary to the density wave theory), but falls into the arm from both inner and outer sides (their Figure 5), and there is no offset between the gas density peak and the stellar spiral arm. One would need a shock on the inner side of the arm (but not on the outer arm side).
The stochastic, self-propagating star formation theory could produce a very irregular, flocculent arm pattern [Citation1], not the quasi-regular pattern seen in the Milky Way.
The swing-amplification theory could produce numerous flocculent arms irregularly located [Citation1], not quasi-regularly spaced as in the Milky Way.
6.2.3. Versions of bar-driven or tidally based theories
The bar-driven theory could induce two strong arms [Citation1], not four as found in the Milky Way. Perhaps each of two straddling galactic bars could perhaps produce its own set of twin arms.
The tidal theory involving a recent passage of the Sagittarius galaxy could induce two strong arms [Citation1], not four as found in the Milky Way. Perhaps the two Magellanic Clouds could produce two sets of twin arms.
6.3. Discussion
There is an issue about the number of different spiral density waves that could be co-existing. Most models assume two arms (m = 2 waves) or four arms (m = 4 waves). Griv et al. [Citation93] assumed a collection of spiral waves, including m = 1–4 arms [Citation93], leading to many arms. To deal with this collection, they employ a superposition of Fourier harmonics and azimuthal wave number m (= number of spiral arms for a given harmonic). To find which m is best, they employed a weight sum S of the velocity difference (observed minus modeled); S is also called later an ‘estimator’. Their best fit to the data employs an ‘estimator’ S that yield similar values (between 24.4 and 24.9 for open clusters in their Table ; between 4 and 5 for masers in their Table ), irrespective of having no spiral (m = 0) being fitted or of any m value being fitted (m = 1–4). They suggested ‘a number of discrete spiral modes of collective oscillations’ (their abstract). Figure 5 in [Citation93] showed their preferred m = 1 spiral mode. Their observed data are restricted to a short distance from the Sun. This small region encompasses a local ‘Orion’ spur in which the Sun is located, and they do not delete the data from this local spur, thus projecting its effects all around the whole Galaxy.
There is an issue about whether there are rings, and not spiral arms. Melnik et al. [Citation94] assumed that there are two rings in the Milky Way, with the Sun as set at a galactic radius of 7.5 kpc, an outer ring R2 above the Sun at a mean galactic radius of 8 kpc, and the other ring R1 below the Sun at a mean galactic radius of 6 kpc (their Figure 1). The rings would be elliptically shaped (their Section 3). Their model tangent, as seen from the Sun, for the ‘ascending’ segment of R1 (Carina segment) is at a galactic longitude of +302° (their Figures 2(b) and 4(a)). This is in between the observations of the arm tangent for the Carina arm near l = 283° and the tangent to the observed Crux–Centaurus arm near l = 310° [Citation52]. Their ring R1 is made up of an ‘ascending’ segment of pitch +6° (near the Carina arm, their Figures 2(b) and 4(a)) and a ‘descending’ segment of pitch −6° (near the Sagittarius arm – their Figures 2(b) and 4(a)), giving a mean ring pitch of 0°. But the observations show the Carina–Sagittarius arm to be a continuous spiral with the same pitch of −13° [Citation20].
Some numerical and analytical models use an original two-arm model, and with some complex dynamics, they end up with a four-arm gas response after a short while (after 300 Myears; beyond 3 kpc galactic radius). In a two-arm structure close to the Galactic Center, each inner arm would develop a fork-like bifurcation producing two gaseous arms beyond. For these paired arms, one arm has a large pitch angle (near 15°) and the other arm has a small pitch angle (near 7°) as in Figure 7 in Pérez-Villegas et al. [Citation95]. Thus when traveling on an orbit at a galactic radius of 8 kpc around the Galactic Center, one would meet successively four gaseous arms, alternating in pitch angle: one with a large pitch, then a small pitch, a large pitch, and a small pitch. This predicted alternation in pitch angle (along a circular orbit) is not seen observationally for the Milky Way – see Table . Also, their azimuthal angular separation, along the circular orbit, between the four arms would also alternate, from small (≈40° between the 2 arms of a single pair), to large (≈140°), to small (≈40° between the 2 arms of the other pair), to large (≈140°). Finally, the predicted large and small angular spacings (along a circular orbit) between adjacent arms are not seen in the Milky Way, as the longitude tangents change monotonically in longitude – see Figure 4(a) here.
A Bayesian computer routine was proposed to model spiral arms, fitted to water and methanol masers. Their Carina arm has a computed arm tangent at longitude 302° [Citation96], contradicting published observations in a range from longitude 281° (CO) to 285° (dust) – see [Citation18]; this discrepancy is significant (17° at 5 kpc from the Sun is 1 450 pc). Also, their arm tangent to Norma is seen at galactic longitude l = 023°, contradicting published observations of the arm tangents to the Norma between l = 16° (masers) and 20° (CO) – see [Citation22]; this discrepancy is significant (4° at 6.5 kpc from the Sun is 454 pc). Finally, maser data can also be found in numerous small ‘spurs’ or ‘armlets’, and if not taken out they could be falsely taken as a part of long spiral arms (as defined with a dozen of other tracers).
7. Other potential players, in the galactic disk
In this section, the aim of this chapter is to provide a summary of some of the remaining galactic players that could affect the galactic disk, yet have not been observed so far to be at a high significance level. These remaining players may be independent, or may be linked; other players may yet to be identified in the future.
7.1. Magnetic field map and relations to the arms
Magnetic fields in the Milky Way’s disk were recently reviewed [Citation97Citation98Citation99Citation100Citation101]. Basically, there are large radial regions where the magnetic field direction is going clockwise (between 2 and 5 kpc from the Galactic Center; and between 7 and 12 kpc from the Galactic Center), or counterclockwise (between 5 and 7 kpc from the Galactic Center) – see [Citation9].
The electro-magnetic waves passing through the interstellar medium of our Galaxy are affected by the magneto-ionic medium in the galactic disk, as the wave’s polarized electric vector is rotated by the Faraday Rotation. Magnetic fields are deduced after the fitting of a Faraday Rotation Measure (RM) to the observed wavelength-dependent angles of the electric vectors of a source’s linear polarization emission.
Finding and eliminating an ambiguity of 180° in the position angle of the electric vector of polarization is important. The Faraday Rotation Measure Synthesis technique is fraught with this problem. Thus, some results [Citation102Citation103] on nearby spiral galaxies have claimed that a galaxy can have three galactic disks, each one on top of the other – a middle one full of stars and gas, and two pseudo-disks without stars and located on each side of the middle one; later it was shown that this is possibly due to the non-removal of the 180° – ambiguity mentioned above [Citation104Citation105].
7.1.1. Magnetic field reversal
Figure shows a representation of the general magnetic field lines and magnetic field directions within the disk of the Milky Way, as obtained with observed pulsar RM [Citation106]. The global disk magnetic field is essentially clockwise (blue arrows), except for a small zone where the magnetic field has reversed (red arrows), and has a small inward pitch angle.
Figure 6. Sketch of the magnetic field vectors.
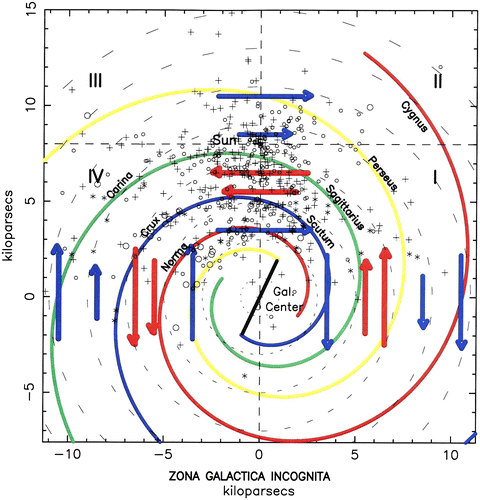
There is a small magnetic inclination inward (a small pitch angle, inward from the circle).
Van Eck et al. [Citation100] used the Faraday rotation measures of extragalactic point sources (quasars or elliptical galaxies) to infer in our interstellar medium an AxiSymmetric spiral magnetic field, with a single reversal zone (their Figure 11). Their model is very similar to that in [Citation106], differing only very near the Galactic bar where RM data are few.
The recent review of the Milky Way’s disk magnetic field by [Citation97], is also very close to that in [Citation106].
Smoothed-particle magneto-hydrodynamics simulations with an imposed spiral potential can reproduce a magnetic reversal at a radius of around 4 to 6 kpc in the Milky Way [Citation107].
Is the magnetic field attached to each arm? Some early models of the magnetic field direction alleged that the magnetic field was directed counterclockwise inside each spiral arm, and clockwise outside each spiral arm (in the interarm) – see [Citation108Citation109]. With four arms, one should see eight magnetic field reversals – the reality is that one does not observe so many magnetic reversals in other spiral galaxies, and neither in the Milky Way.
7.1.2. Magnetic field pitch
Kronberg & Newton-McGee [Citation110] found that the pitch angle of the magnetic field lines (outside spiral arms) is close to −5.5° ± 1°, thus a bit different than the pitch angle of gas, molecules and dust inside spiral arms at −13.1°, as found observationally in many arm tracers.
In nearby spiral galaxies, van Eck et al. [Citation111] found a small difference between the pitch angle of the magnetic field and the pitch angle of the stellar arm, with the magnetic pitch angle being more open (larger) than that of the stellar arm, by about 5°–10° (their Section 5.2).
The early issue about a magnetic control of a spiral arm has gone away. Some early models employed a strong magnetic field, enough to control the arm. In this case, the pitch angle of the magnetic field would be the same as the pitch angle of the stellar arm.
The basic density wave theory does predict a small difference in pitch angle between the interarm magnetic field direction and arm direction [Citation59]. Assuming a four-arm model in the galactic disk, the orbital path of gas and magnetic field around the Galactic Center becomes a continuous series of four segmented spirals [Citation9]. When the orbit of a gas and dust patch enters a spiral arm, the gas is shocked and the dust is heated, and the magnetic field line is reoriented.
7.2. Dark matter map and relations to the arms
Is there a dark matter disk? Since most mergers of nearby dwarf galaxies with the Milky Way end up in the Milky Way disk, some dark matter and newly accreted stars from these dwarfs should be found also in the Milky Way’s disk. Bregman [Citation112] argued for a model where unobservable baryons are in the halo, because either they were heated and expelled from the disk by supernovae or galactic superwinds, or they were heated early in the halo and thus resisted the infall on the disk and were later pushed in the intracluster medium.
McKee et al. [Citation113] reanalyzed the observed data in the solar neighborhood (stars, gas and baryons) to find the local density of all matter at about 0.10 ± 0.01 Msun/pc3; this value comprises a dark matter component at about 0.013 ± 0.003 Msun/pc3 (about 13% of all matter), but there is no indication of a vertical structure (in the plane or homogeneous) or of an horizontal structure (in an arm or an interarm). Figure 1 in Xia et al. [Citation114] showed that the median local dark matter density for six measurements between 2013 and 2016 to be 0.014 ± 0.004 Msun/pc3 (about 14% of all matter). Independently, using the Sloan Digital Sky Survey with about 10 000 stars, Read [Citation115] finds a rather weak ‘dark matter disc’, amounting to about 10% of the total mass in the local volume (8 < Rsun < 9 kpc; 0.5 kpc < z < 0.5 kpc). McGaugh [Citation116] found a solar neighborhood local dark matter density of 0.009 Msun/pc3 (her Equation (9)), or about 9% of the total matter density. Using the Gaia-ESO Spectroscopic Survey with 4 600 stars, the observed absence of accreted stars in the disk suggests an absence near the Sun of dark matter in the disk (the ‘dark disk’ of [Citation117]).
It is noteworthy that the mean dark matter density from the above values (from 9 to 14%) is not higher than a three-sigma result.
8. Conclusion, summary, and outlook
In summary, a review of the basic observed spiral arms was made (Figures ). In the latest maps of the Milky Way galaxy, each spiral arm has separated into slightly different parallel lanes (onion like), each with different chemical components (arm tracers). The presence of offsets among the arm tracers in the Milky Way (Figures 4(a) and (b)) confirms each arm as a true arm, and thus disfavors any two-arm model. A summary of what’s close to the Galactic Center, encompassing the start of the spiral arms, is given (Figure ). A recent model magnetic field picture is shown (Figure ).
In Section 2, the reader has been provided with a global view of the galactic disk domain, summarizing numerous papers with respect to the locations of spiral arms, their shape, pitch, separation, how to subtract foreground and background emissions in order to get the data for a given spiral arm. The Sun is located in a ‘spur’ in an interarm, between the Sagittarius and the Perseus arm. Another ‘spur’ has been observed in an interarm, around longitude 35° and radial velocity near +75 km/s, somewhere between the Scutum arm and the Sagittarius arm.
In Section 3, the reader has been provided with a radial velocity view of the gas in each spiral arm, located along the galactic disk. Older, published cartographic maps of the spiral arms showing weird ‘fingers of God’ pointing to the Sun were shown through models to be velocity jumps (not linked to orbital velocity) masquerading as pseudo physical jumps (fingers).
In Section 4, arm tangents and arm widths, with the mean galactic longitude at which each arm tracer, chemical or otherwise, are summarized. Always, for each spiral arm, the arm tangent for the hot dust (inner arm edge) is closer to the Galactic Meridian (at galactic longitude 0°) than the arm tangent for the 12CO tracer (middle arm position). This shows the Galactic Meridian to be some kind of ‘axis of reversal’ with respect to arm tracers.
In Section 5, the reader is provided with a view of the galactic interior, the inner area within about 3 kpc of the Galactic Center [Citation131]. A discussion there touched on the existence of an inner molecular ring, near a galactic radius of 3.5 kpc, and on the existence of a long thin physical bar crossing some spiral arms, as well as the ‘3-kpc-arm’ features that could be physical streams (not arms).
In Section 6, the reader is appraised of angular velocities and potential matches between observational data and model predicted features and numerical simulations, including shock locations, tracer offsets, HII peak. Some numerical simulations are compared to the overall observational data.
In Section 7, some other potential players in the galactic disk were briefly reviewed. The observed weak strength of the global disk magnetic field, and the observed weakness of the dark matter in the galactic disk, make them both minor players in maintaining the spiral arms. The pitch angle of the magnetic field in the arm is observed to be slightly different than that of the stellar arms, as predicted by theories involving magnetic fields.
Table lists recent observational efforts and results to find the exact distance from the Sun to the GC, and the circular velocity of the local stars around the GC.
Table , listing the observed linear separation of each specific arm tracer from the 12CO tracer, in each spiral arms, indicates a notable substructure inside an arm. With the discovery of substructure in spiral arms, reversing across the Galactic Meridian, the Milky Way is pointing us to a specific formation model for its spiral arms, with a very specialized onion-like ordering.
Table details the pitch angle of each spiral arm, as observed by different methods. Each method has some assumptions, and covers a different portion of each arm. Hence statistics can be used to obtain a more global picture.
Table , listing the angular rotation of some of the important Milky Way features, indicates some possible links. Unfortunately, the error bars are currently not small enough to eliminate many theories on these topics.
Still to be determined are the locations and relations between the 100-pc giant molecular filaments and the nearby spiral arms. Are they near the inner arm edge or in the interarm as predicted by some theories, or do they form the ‘backbone’ at the center of a spiral arm? Their exact distances from the Sun are not well known enough, but would have to be more precise than a half-arm width (340 pc).
Acknowledgments
The figure production made use of the PGPLOT software at the NRC Canada’s Herzberg A&A in Victoria, BC. I thank two anonymous referees for useful, careful, and historical suggestions.
Disclosure statement
No potential conflict of interest was reported by the author.
Funding
This work was supported by TARE-2017-J98W.
References
- Dobbs C, Baba J. Dawes review 4: spiral structures in disc galaxies. Publ Astron Soc Aust. 2014;31: article id 35.
- Elmegreen DM, Elmegreen BG. Flocculent and grand design spiral structure in field, binary and group galaxies. MNRAS. 1982;201:1021–1034.10.1093/mnras/201.4.1021
- Elmegreen GG, Elmegreen DM. Galaxies, spiral structure. IPAC. 1989. Available from: http://ned.ipac.caltech.edu/level5/ESSAYS/Elmgreen/elmgreen.html
- Bland-Hawthorn J, Gerhard O. The Galaxy in context: structural, kinematic, and integrated properties. Ann Rev Astron Astrophys. 2016;54:529–596.10.1146/annurev-astro-081915-023441
- Momany Y, Zaggia S, Gilmore G, et al. Outer structure of the Galactic warp and flare: explaining the Canis Major over-density. Astron Astrophys. 2006;451:515–538.10.1051/0004-6361:20054081
- Shu FH. Six decades of spiral density wave theory. Ann Rev Astron Astrophys. 2016;54:667–724.10.1146/annurev-astro-081915-023426
- Vallee JP. Metastudy of the spiral structure of Our home galaxy. Astrophys J. 2002;566:261–266.10.1086/apj.2002.566.issue-1
- Vallée JP. The start of the Sagittarius spiral arm (Sagittarius origin) and the start of the Norma spiral arm (Norma origin) – model-computed and observed arm tangents at galactic longitudes –20° < l < +23°. Astron J. 2016;151: article id 55.
- Vallée JP. Magnetic fields in the galactic Universe, as observed in supershells, galaxies, intergalactic and cosmic realms. New Astron Rev. 2011;55:91–154.10.1016/j.newar.2011.01.002
- Hawkins K, Jofré P, Masseron T, et al. Using chemical tagging to redefine the interface of the Galactic disc and halo. MNRAS. 2015;453:758–774.10.1093/mnras/stv1586
- Genzel R, Pichon C, Eckart A, et al. Stellar dynamics in the Galactic Centre: proper motions and anisotropy. MNRAS. 2000;317:348–374.10.1046/j.1365-8711.2000.03582.x
- Vallée JP. Recent advances in the determinations of some Galactic constants in the MilkyWay. Astrophys Space Sci. 2017;362: article id 79.
- Vallee JP. The Milky Way’s spiral arms traced by magnetic fields, dust, gas, and stars. Astrophys J. 1995;454:119–124.10.1086/176470
- Vallée JP. The spiral arms and interarm separation of the Milky Way: an updated statistical study. Astron J. 2005;130:569–575.10.1086/431744
- Vallée JP. Constraining the pitch angle of the Galactic spiral arms in the Milky Way. New Astron. Rev. 2017;79: in press.
- Vallée JP. New velocimetry and revised cartography of the spiral arms in the milky way – a consistent symbiosis. Astron J. 2008;135:1301–1310.10.1088/0004-6256/135/4/1301
- Vallée JP. A synthesis of fundamental parameters of spiral arms, based on recent observations in the Milky Way. Int J Astron Astrophys. 2013;3:20–28.10.4236/ijaa.2013.31003
- Vallée JP. The spiral arms of the Milky Way: the relative location of each different arm tracer, within a typical spiral arm width. Astron J. 2014;148: article id 5.
- Vallee JP. On a persistent large discrepancy in some parameters of the spiral arms in the Milky Way – a statistical and modelling analysis. MNRAS. 2014;442:2993–2998.10.1093/mnras/stu1068
- Vallee JP. Different studies of the global pitch angle of the Milky Way’s spiral arms. MNRAS. 2015;450:4277–4284.10.1093/mnras/stv862
- Drimmel R. Evidence for a 2-armed spiral in the Milky Way. Astron Astrophys. 2000;358:L13–L16.
- Vallée JP. A substructure inside spiral arms, and a mirror image across the Galactic Meridian. Astrophys J. 2016;821: article id 53.
- Reid MJ, Menten KM, Brunthaler A, et al. Trigonometric parallaxes of high mass star forming regions: the structure and kinematics of the Milky Way. Astrophys J. 2014;783: article id 130.
- Uyaniker B, Furst E, Reich W, et al. The Cygnus superbubble revisited. Astron Astrophys. 2001;371:675–697.
- Olano CA. The hypothesis of the local supercloud and the nearby moving groups of stars. MNRAS. 2016;458:4354–4367.10.1093/mnras/stw538
- Hou LG, Han JL. The observed spiral structure of the Milky Way. Astron Astrophys. 2014;569: article id 125.
- Bobylev VV. The Gould belt, the de Vaucouleurs-Dolidze belt, and the Orion arm. Astron Lett. 2014;40:783–792.10.1134/S1063773714120020
- Lépine JRD, Michtchenko TA, Barros DA, et al. The dynamical origin of the local arm and the sun’s trapped orbit. Astrophys J. 2017;843: article id 48.
- Rigby AJ, Moore TJ, Plume R, et al. CHIMPS: the 13CO/C18O (J = 3-2) heterodyne inner Milky Way plane survey. MNRAS. 2016;456:2885–2899.10.1093/mnras/stv2808
- García P, Bronfman L, Nyman L-A, et al. Giant molecular clouds and massive star formation in the southern Milky Way. Astrophys J Suppl Ser. 2014;212: article id 2.
- Bronfman L. Molecular clouds and young massive stars in the galactic disk. Astrophys Space Sci Lib. 1992;180:131–154.10.1007/978-94-011-2813-1
- Hou LG, Han JL. Offset between stellar spiral arms and gas arms of the Milky Way. MNRAS. 2015;454:626–636.10.1093/mnras/stv1904
- Gao J, Jiang BW, Li A. Mid-infrared extinction and its variation with galactic longitude. Astrophys J. 2009;707:89–102.10.1088/0004-637X/707/1/89
- Foster T, MacWilliams J. Modeling the neutral hydrogen interstellar medium: a better kinematic distance tool. Astrophys J. 2006;644:214–228.10.1086/apj.2006.644.issue-1
- Russeil D, Adami C, Georgelin Y. Revised distances of Northern HII regions. Astron Astrophys. 2007;470:161–171.10.1051/0004-6361:20066051
- Englmaier P, Gerhard O. Gas dynamics and large-scale morphology of the Milky Way galaxy. MNRAS. 1999;304:512–534.10.1046/j.1365-8711.1999.02280.x
- Pandian JD, Goldsmith PE. The Arecibo methanol maser galactic plane survey. II. Statistical and multiwavelength counterpart analysis. Astrophys J. 2007;669:435–445.10.1086/509305
- Stark AA, Lee Y. Giant molecular clouds are more concentrated toward spiral arms than smaller clouds. Astrophys J. 2006;641:L113–L116.10.1086/504036
- Zucker C, Battersby C, Goodman A. The skeleton of the Milky Way. Astrophys J. 2015;815: article id 23.
- Dobbs CL, Bonnell IA, Pringle JE. The formation of molecular clouds in spiral galaxies. MNRAS. 2006;371:1663–1674.10.1111/j.1365-2966.2006.10794.x
- Yang J, Jiang Z, Wang M, et al. A large-scale molecular line survey for cold IRAS sources in the galaxy. I. The CO (J = 1-0) data. Astrophys J Suppl Ser. 2002;141:157–185.10.1086/apjs.2002.141.issue-1
- Brown C, Dickey JM, Dawson JR, et al. A complete atlas of HI absorption toward HII regions in the southern galactic plane survey (SGPS I). Astrophys J Suppl Ser. 2014;211: article id 29.
- Kothes R, Dougherty SM. The distance and neutral environment of the massive stellar cluster Westerlund 1. Astron Astrophys. 2007;468:993–1000.
- Levine ES, Blitz L, Heiles C. The spiral structure of the outer Milky Way in hydrogen. Science. 2006;312:1773–1777.10.1126/science.1128455
- Baba J, Asaki Y, Makino J, et al. The origin of large peculiar motions of star-forming regions and spiral structures of our galaxy. Astrophys J. 2009;706:471–481.10.1088/0004-637X/706/1/471
- Pohl M, Englmaier P, Bissantz N. Three-dimensional distribution of molecular gas in the barred Milky Way. Astrophys J. 2008;677:283–291.10.1086/523317
- Li A, Wang S, Gao J, et al. Dust in the local group. In: Freeman KC, Elmegreen BG, Block DL, et al, editors. Lessons from the local group. New York (NY): Springer; 2015. p. 85–105.
- Bobylev VV, Bajkova AT. Determination of the galactic rotation curve from OB stars. Astron Lett. 2015;41:473–488.10.1134/S1063773715080010
- Vázques RA, Moitinho A, Carraro G, et al. Open clusters in the thirs galactic quadrant. III. Alleged binary clusters. Astron Astrophys. 2010;511: article id 38.
- Vazquez RA, May J, Carraro G, et al. Spiral structure in the outer galactic disk. I. The third galactic quadrant. Astrophys J. 2008;672:930–939.10.1086/523308
- Velusamy T, Langer WD, Goldsmith PF, et al. Internal structure of spiral arms traced with [CII]: unraveling the WIM, HI, and molecular emission lanes. Astron Astrophys. 2015;578: article id 135.
- Vallée JP. Catalog of observed tangents to the spiral arms in the Milky Way Galaxy. Astrophys J Suppl Ser. 2014;215: article id 1.
- Grabelsky DA, Cohen RS, Bronfman L, et al. Molecular clouds in the Carina arm – large scale properties of molecular gas and comparison with HI. Astrophys J. 1987;315:122–141.10.1086/165118
- Bronfman L, Cohen RS, Alvarez H, et al. A CO survey of the southern Milky Way – the mean radial distribution of molecular clouds within the solar circle. Astrophys J. 1988;324:248–266.10.1086/165892
- Alvarez H, May J, Bronfman L. The rotation of the Galaxy within the solar circle. Astrophys J. 1990;348:495–502.10.1086/168258
- Roman-Duval J, Jackson JM, Heyer M, et al. Kinematic distances to molecular clouds identified in the Galactic Ring Survey. Astrophys J. 2009;699:1153–1170.10.1088/0004-637X/699/2/1153
- Roman-Duval J, Heyer M, Brunt C, et al. Distribution and mass of diffuse and dense CO Gas in the Milky Way. Astrophys J. 2016;818: article id 144.
- Lin CC, Shu FH. On the spiral structure of disk galaxies. Astrophys J. 1964;140:646–655.10.1086/147955
- Roberts WW. Theoretical aspects of galactic research. Vistas Astron. 1975;19:91–109.10.1016/0083-6656(75)90008-2
- Goodman AA, Alves J, Beaumont CN, et al. The bones of the Milky Way. Astrophys J. 2014;797: article id 53.
- Wang K, Testi L, Burkert A, et al. A census of large scale (>10pc), velocity coherent, dense filaments in the Northern galactic plane: automated identification using minimum spanning tree. Astrophys J Suppl Ser. 2016;226: article id 9.
- Ragan SE, Henning T, Tackenberg J, et al. Giant molecular filaments in the Milky Way. Astron Astrophys. 2014;568: article id 73.
- Abreu-Vicente J, Ragan S, Kainulainen J, et al. Giant molecular filaments in the Milky Way II: the fourth Galactic quadrant. Astron Astrophys. 2016;590: article id 131.
- Duarte-Cabral A, Dobbs CL. What can simulated molecular clouds tell us about real molecular clouds? MNRAS. 2016;458:3667–3683.10.1093/mnras/stw469
- Kharchenko NV, Piskunov AE, Schilbach E, et al. Global survey of star clusters in the Milky Way. Astron Astrophys. 2012;543: article id 156.
- Siebert A, Famaey B, Binney J, et al. The properties of the local spiral arms from RAVE data: two-dimensional density wave approach. MNRAS. 2012;425:2335–2342.10.1111/j.1365-2966.2012.21638.x
- Urquhart JS, Figura CC, Moore TJ, et al. The RMS survey: galactic distribution of massive star formation. MNRAS. 2014;437:1791–1807.10.1093/mnras/stt2006
- Steiman-Cameron TY. Twin masks of spiral structure? A local perspective. In: Block DL, Freeman KC, Puerari I, editors. Galaxies and their masks. New York (NY): Springer; 2010. p. 45–58.10.1007/978-1-4419-7317-7
- Steiman-Cameron TY, Wolfire M, Hollenbach D. COBE and the galactic interstellar medium: geometry of the spiral arms from fir cooling lines. Astrophys J. 2010;722:1460–1473.10.1088/0004-637X/722/2/1460
- Benjamin RA. The spiral structure of the Galaxy: something old, something new. Astron Soc Pac Conf Ser. 2008;387:375–380.
- Benjamin RA. Spiral arm tangencies in the Milky Way. IAU Symp. 2009;254:319–322.
- Honig ZN, Reid MJ. Characteristics of spiral arms in late-type galaxies. Astrophys J. 2015;800: article id 53.
- Bloemen JB, Deul ER, Thaddeus P. Decomposition of the FIR Milky Way observed by IRAS. Astron Astrophys. 1990;233:437–455.
- Bronfman L. Massive star formation in the southern Milky Way. Astrophys Space Sci. 2008;313:81–85.
- Green JA, Caswell JL, McClure-Griffiths NM, et al. Major structures of the inner Galaxy delineated by 6.7-GHz methanol masers. Astrophys J. 2011;733: article id 27.
- Vallée JP. On the many ‘3-kiloparsec arms’ – shocked wave and nuclear rotation. Astrophys Space Sci. 2017;362: article id 84.
- Wegg C, Gerhard O, Portail M. The structure of the Milky Way’s bar outside the bulge. MNRAS. 2015;450:4050–4069.10.1093/mnras/stv745
- Martinez-Valpuesta I, Gerhard O. Unifying a boxy bulge and planar long bar in the Milky Way. Astrophys J. 2011;734:L20–L23.10.1088/2041-8205/734/1/L20
- Monari G, Famaey B, Siebert A, et al. Staying away from the bar: the local dynamical signature of slow and fast bars in the Milky Way. MNRAS. 2017;465:1443–1453.10.1093/mnras/stw2807
- Dobbs CL, Burkert AS. The myth of the molecular ring. MNRAS. 2012;421:2940–2946.10.1111/mnr.2012.421.issue-4
- Dambis AK, Berdnikov LN, Efremov YN, et al. Classical Cepheids and the spiral structure of the milky way. Astron Lett. 2015;41:489–500.10.1134/S1063773715090017
- Gerhard O. Pattern speed in the Milky Way. Mem Soc Astron Ital. 2011;18:185–188.
- Li Z, Gerhard O, Shen J, et al. Gas dynamics in the Milky Way: a low parttern speed model. Astrophys J. 2016;824: article id 13.
- Junqueira TC, Chiappini C, Lepine JR, et al. A new method for estimating the pattern speed of spiral structure in the Milky Way. MNRAS. 2015;449:2336–2344.10.1093/mnras/stv464
- Koda J, Scoville N, Heyer M. Evolution of molecular and atomic gas phases in the Milky Way. Astrophys J. 2016;823: article id 76.
- Rodriguez-Fernandez NJ, Combes F. Gas flow models in the Milky Way embedded bars. Astron Astrophys. 2008;489:115–133.
- Qin Y, Shen J, Li Z-Y, et al. Kinematics of the X-shaped Milky Way bulge: expectations from a self-consistet N-body model. Astrophys J. 2015;808: article id 75.
- Hodges-Kluck EJ, Miller MJ, Bregman J. The rotation of the hot gas around the Milky Way. Astrophys J. 2016;822: article id 21.
- Bissantz N, Englmaier P, Gerhard O. Gas dynamics in the Milky Way: second pattern speed and large-scale morphology. MNRAS. 2003;340:949–968.10.1046/j.1365-8711.2003.06358.x
- Gittins DM, Clarke CJ. Constraining corotation from shocks in tightly wound spiral galaxies. MNRAS. 2004;349:909–921.10.1111/mnr.2004.349.issue-3
- Renaud F, Bournaud F, Emsellem E, et al. A sub-parsec resolution simulation of the Milky Way: global structure of the interstellar medium and properties of molecular clouds. MNRAS. 2013;436:1836–1851.10.1093/mnras/stt1698
- Baba J, Morokuma-Matsui K, Miyamoto Y, et al. Gas velocity patterns in simulated galaxies: observational diagnostics of spiral structure theories. MNRAS. 2016;460:2472–2481.10.1093/mnras/stw987
- Griv E, Kharchenko N, Piskunov A, et al. Spectrum of Lin-Shu-type density waves in the Galaxy: a number of discrete spiral modes of collective oscillations? MNRAS. 2015;453:1981–1989.10.1093/mnras/stv1636
- Melnik AM, Rautiainen P, Glushkova EV, et al. Evidence of the galactic outer ring R1 R2’ from young open clusters and OB-associations. Astrophys Space Sci. 2016;361: article id 60.
- Perez-Villegas A, Gomez GC, Pichardo B. The galactic branches as a possible evidence for transient spiral arms. MNRAS. 2015;451:2922–2932.10.1093/mnras/stv1157
- Ragan SE, Moore TJ, Eden DJ, et al. The prevalence of star formation as a function of Galactocentric radius. MNRAS. 2016;462:3123–3129.10.1093/mnras/stw1870
- Beck R. Magnetic fields in spiral galaxies. Astron Astrophys Rev. 2016;24: article id 4.
- Haverkorn M. Magnetic fields in the Milky Way. Astrophys Space Sci Lib. 2015;407:483–506.
- Heiles C, Haverkorn M. Magnetic fields in the multiphase interstellar medium. Space Sci Rev. 2012;166:293–305.10.1007/s11214-012-9866-4
- Van Eck CL, Brown JC, Stil JM, et al. Modeling the mangetic field in the Galactic disk using new rotation measure observations from the Very Large Array. Astrophys J. 2011;728: article id 97.
- Vallée JP. Magnetic Milky Way. Eur Astron Soc Publ Ser. 2012;56:81–86.
- Braun R, Heald G, Beck R. The Westerbork sings survey. III. Global magnetic field topology. Astron Astrophys. 2010;514: article id 42.
- Heald G, Braun R, Edmonds R. The Westerbork sings survey. II. Polarization, Faraday rotation, and magnetic fields. Astron Astrophys. 2009;509:409–435.
- Vallée JP. Creating a ‘Faraday Ghost’ inside the rotation measure synthesis technique, through a wide observational ‘Gap’ in wavelength coverage. Int J Astron Astrophys. 2013;3:99–107.10.4236/ijaa.2013.32011
- Mao SA, Ott J, Zweibel E. Wide-band Jansky Very Large Array polarization observations of M51. NRAO VLA Sky Survey Workshop. 2014. Available from: http://science.nrao.edu/science/surveys/vlass/Mao_M51_VLASSworkshopJan2014_Poster.pdf
- Vallée JP. An improved magnetic map of the Milky Way, with the circularly orbiting gas and magnetic field lines crossing the dusty stellar spiral arms. Astrophys J. 2008;681:303–310.10.1086/529162
- Dobbs CL, Price DJ, Pettitt AR, et al. Magnetic field evolution and reversals in spiral galaxies. MNRAS. 2016;461:4482–4495.10.1093/mnras/stw1625
- Han JL, Manchester RN, Lyne AG, et al. Pulsar rotation measures and the large-scale structure of the Galactic magnetic field. Astrophys J. 2006;642:868–881.10.1086/apj.2006.642.issue-2
- Han JL. Pulsars as excellent probes for the magnetic structure in our Milky Way. IAU Symp. 2013;291:223–228.
- Kronberg PP, Newton-McGee kJ. Remarkable symmetries in the Milky Way disc's magnetic field. Publ Astron Soc Aust. 2011;28:171–176.10.1071/AS10045
- Van Eck CL, Brown JC, Shukurov A, et al. Magnetic fields in a sample of nearby spiral galaxies. Astrophys J. 2015;799: article id 35.
- Bregman JN. The disk-halo connection and where has all the gas gone? EAS Publ Ser. 2012;56:5–17.10.1051/eas/1256002
- McKee CF, Parravano A, Hollenbach DJ. Stars, gas, and dark matter in the solar neighbourhood. Astrophys J. 2015;814: article id 13.
- Xia Q, Liu C, Mao S, et al. Determining the local dark matter density with LAMOST data. MNRAS. 2016;458:3839–3850.10.1093/mnras/stw565
- Read JI. The local dark matter density. J Phys G: Nucl Part Phys. 2014;41: article id 063101.
- McGaugh SS. The surface density profile of the galactic disk from the terminal velocity curve. Astrophys J. 2016;816: article id 42.
- Ruchti GR, Read JI, Feltzing S, et al. The Gaia-ESO Survey: a quiescent Milky Way with no significant dark/stellar accreted disc. MNRAS. 2015;450:2874–2887.10.1093/mnras/stv807
- Gillessen S, Plewa PM, Eisenhauer F, et al. An update on monitoring stellar orbits in the Galactic Center. Astrophys J. 2017;837: article id 30.
- McMillan PJ. The mass distribution and gravitational potential of the Milky Way. MNRAS. 2017;465:76–94.10.1093/mnras/stw2759
- Branham RL. The distance to the Galactic Center determined by G, K, and M stars. Astrophys Space Sci. 2017;362: article id 29.
- Bobylev VV. Kinematics of the galaxy from Cepheids with proper motions from the Gaia DR1 catalogue. Astron Lett. 2017;43:152–158.10.1134/S106377371703001X
- Bobylev VV, Bajkova AT. Kinematics of the galaxy from OB stars with proper motions from the Gaia DR1 catalogue. Astron Lett. 2017;43:159–166.10.1134/S1063773717030021
- Krishnan V, Ellingsen SP, Reid MJ, et al. First parallax measurements toward. A 6.7 GHz methanol maser with the Australian long baseline array – distance to G 339.884-1.259. Astrophys J. 2015;805: article id 129.
- Du X, Xu Y, Yang J, et al. Outer arm in the 2nd galactic quadrant: structure. Astrophys J Suppl Ser. 2016;224: article id 7.
- Nakanishi H, Sofue Y. Three-dimensional distribution of the ISM in the Milky Way galaxy III The total neutral gas disk. Publ Astron Soc Jpn. 2016;68: article id 5.
- Krishnan V, Ellingsen SP, Reid M, et al. Parallaxes of 6.7-GHz methanol masers towards the G 305.2 high-mass star formation region. MNRAS. 2017;465:1095–1105.10.1093/mnras/stw2850
- Sakai N, Nakanishi H, Matsuo M, et al. Outer rotation curve of the Galaxy with VERA III: astrometry of IRAS 07427-2400 and test of the density-wave theory. PASJ. 2015;67: article id 69.
- Sun Y, Xu Y, Yang J, et al. A possible extension of the Scutum-Centaurus arm into the outer second quadrant. Astrophys J Lett. 2015;798:L27–L32.
- Hachisuka K, Choi YK, Reid M, et al. Parallaxes of star-forming regions in the outer spiral arm of the Milky Way. Astrophys J. 2015;800: article id 2.
- Nagayama T, Omodaka T, Nakagawa A, et al. Astrometry of Galactic star-forming region Onsala 1 with VERA: estimation of angular velocity of galactic rotation at the Sun. Publ Astron Soc Jpn. 2011;63:23–30.10.1093/pasj/63.1.23
- Vallée JP. The Norma spiral arm: large-scale pitch angle. Astrophys Space Sci. 2017;362: article id 173.