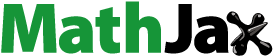
ABSTRACT
In this study, foamed ceramics were successfully prepared using cyanide tailings (CTs) with a high sulfur content as the main raw material. The effects of sintering temperature, holding time, and heating rate on the bulk density, water absorption, porosity, pore structure, and compressive strength of foamed ceramics were systematically investigated. The results showed that the elevated sintering temperature promoted the generation of liquid phases, resulting in an obvious enlargement of the pore size and a decrease in bulk density. In addition, a similar evolution trend could be found in the pore size and bulk density with the extension of the holding time, which was attributed to the degrees of silicon carbide reaction. Foamed ceramics with small pore sizes could be prepared at a heating rate that did not exceed 4°C/min. Then, the higher heating rates could bring abnormally large pores and be detrimental to the mechanical properties of the foamed ceramics, which were proven to be related to the decomposition of FeS2 introduced by CTs during the heating process. In conclusion, the foamed ceramics prepared with CTs in this study provide effective solid waste utilization and have good application prospects in wall composite one-piece insulation panels.
1. Introduction
Cyanide tailings (CTs) are hazardous solid waste residues produced during the production of gold using the cyanide gold extraction process [Citation1,Citation2]. With the increasing demand for gold and the decline in ore grade, the global production of CTs has been increasing yearly. However, most gold ores in China have complex compositions and lower gold contents, making metallurgical beneficiation difficult. It is estimated that more than 24.5 million tons of gold tailings are produced annually in China [Citation3]. Extraction by cyanide leaching is the main method for gold ore dressing and smelting worldwide. However, using highly toxic cyanide leads to a serious excess of CN– during gold extraction tailings, and long-term storage causes serious pollution of soil and groundwater, which may pose a serious threat to the surrounding environment and public health [Citation4]. Therefore, it is urgent to explore effective ways of resourceful and harmless use of CTs. Currently, the utilization of CTs mainly includes the recovery of valuable metals [Citation5–7], glass ceramic foams [Citation8,Citation9], construction materials [Citation10,Citation11], backfilling materials for mining areas [Citation12], and reclamation [Citation13]. Studies have shown that CTs contain large amounts of aluminosilicate minerals, which can be used to prepare architectural ceramics [Citation14]. For example, Wei et al. [Citation15] investigated the possibility of using gold mine tailings to produce sintered bricks and found the good sintering properties of gold mine tailings, but those of sintered bricks are significantly affected by the sintering parameters.
Besides, it is urgent to develop efficient detoxification and harmless utilization technologies of CTs. Studies have shown that cyanide in cyanide tailings can be effectively removed under high-temperature roasting, which is safe and harmless to handle [Citation16,Citation17]. Hai et al. [Citation18] improved the existing high-temperature incineration process for the cyanide removal of CTs from Liaoning Paishanlou Company. The results showed that the total cyanide content of CTs was reduced from 3.27 mg/L to below 0.01 mg/L after roasting at 250–300°C for 30–40 min, and the decomposition rate reached more than 99.69%. Roasting can destroy the coordination bond inside the heavy metal complex cyanide compound, and make it decompose into nontoxic substances such as ammonia (NH3), acetic acid (CH3COOH), and CO2. It indicates that the utilization of CTs in the ceramic-based material preparation not only contributes to the detoxification of CTs, but also realizes their harmless utilization.
In recent years, due to the rapid growth of construction areas in China, building energy consumption has risen to one-third of the total social energy consumption [Citation19]. At present, the most widely used insulation material for building walls is organic insulation material. Although it has good thermal and acoustic insulation properties, many shortcomings exist, such as easy combustion, a large deformation coefficient, and a short service life [Citation20]. Foamed ceramic is a ceramic-based porous insulation material that consists of a continuous solid-phase skeleton and closed pores [Citation21]. Foamed ceramic has attracted much attention in building thermal insulation owing to its excellent performance, such as light weight, high strength, heat insulation, flame retardance, and durability. Recently, researchers have successfully prepared foamed ceramics for construction insulation from solid wastes, such as fly ash [Citation22,Citation23], tile polishing waste [Citation24], and red mud [Citation25,Citation26], which not only eliminates industrial solid waste and effectively reduces environmental pollution, but also enhances the comprehensive performance of building insulation materials. Currently, foamed ceramics are commonly prepared by high-temperature foaming methods, and sintering parameters such as sintering temperature, heating rates, and holding time directly impact the properties of foamed ceramic samples [Citation27,Citation28]. Hui et al. [Citation20] prepared ceramic foams using extracted titanium slag and gold tailings as raw materials. They found that the increased sintering temperature led to the melting of more crystalline phases and the intensification of the TiC oxidation reaction, as well as an increase in the amount of liquid phase and gas pressure, resulting in a remarkable decrease in the bulk density and flexural strength of the samples. This result indicated that sintering parameters, such as sintering temperature, were important factors in determining pore formation and the properties of foamed ceramics.
In this work, foamed ceramics were prepared using CTs as the main raw material by a high-temperature foaming process. The influence of sintering temperature, holding time, and heating rate on the properties and pore structure of cyanide tailing foamed ceramics was systematically explored. In addition, the influence mechanism was investigated by analyzing the decomposition behavior of sulfur-containing minerals in CTs during the sintering process.
2. Experimental
2.1. Raw materials
The CTs used in this study were acquired from the gold refinery in Shandong Province, China. Analysis showed that the content of the total cyanide in CTs was 1710 mg/kg. Fly ash (FA) and feldspar (Fsp) were obtained from Henan Province and Hebei Province, China, respectively. The chemical compositions of these raw materials are listed in . The main chemical components of the CTs were SiO2 and Al2O3, as well as small amounts of Fe2O3, SO3, CaO, MgO, Na2O, and K2O. The mineral composition of CTs is displayed in . As seen in , the main mineral phases were quartz, pyrite, albite, anhydrite, and muscovite. Sodium carbonate (Na2CO3) and magnesium oxide (MgO) (AR, Sinopharm Chemical Reagent Co., Ltd., Shanghai, China) were added as fluxing agents. Silicon carbide powder (SiC) was selected as the foaming agent.
Table 1. The chemical composition of the raw materials (wt.%).
2.2. Sample preparation
First, the crushed CTs and other raw materials were dried at 105°C and passed through a 200-mesh screen. The powder mixture consisted of 40 wt.% CTs, 36 wt.% FA, 18 wt.% Fsp, 5 wt.% MgO, 1 wt.% Na2CO3, and 0.25 wt.% SiC prefabricated as the base formulation. The powder mixture was wet-mixed in a ceramic ball mill with a mass ratio of powder, ball, and water 1:4:0.8 for 7 h. After drying at 110°C in an electric oven, the slurry was dry-mixed for 1 h to obtain foamed ceramic powders. Then, the powders were shaped in an alumina cylindrical crucible by pressing at 10 MPa for 20 s. Finally, the obtained green bodies were heated at designed heating rates to the sintering temperature (1110–1170°C) and held for different times (20–80 min) in an electric furnace. The samples were cooled naturally to room temperature in the furnace and tested for their properties after drilling. The designed sintering parameters are listed in .
Table 2. The designed sintering parameters of samples.
2.3. Characterization
The chemical compositions of raw materials were determined by X-ray fluorescence spectroscopy (XRF, Thermo Fisher Scientific ARL Advant’X IntellipowerTM 3600, USA). The phase compositions of samples were detected by X-ray diffraction (XRD, Bruker D8 Advance, Germany). The content of cyanide in the CTs and the produced foamed ceramics were determined according to the HJ 745–2015 national environmental protection standard of China “Soil-Determination of Cyanide and Total Cyanide-Spectrometric Method”. The pore morphology of the samples was observed using a stereomicroscope (Beiyinhu ZS7045, China). The pore size distribution and average pore size were counted and calculated by the software (ImageView, Microdemo, China), and the pore size dispersion coefficient was calculated via EquationEquation (1)(1)
(1) and used to evaluate the uniformity of the pore size distribution of the material. The sintering and foaming behavior of green bodies under high temperatures were characterized by the self-designed high-temperature visualization and deformation analysis system. The amorphous phase content was identified using MDI Jade 6.0 software by simulation of the XRD patterns of the samples. Scanning electron microscopy (SEM, Nano SEM430, FEI, USA) was used to observe the microscopic morphology of the samples. Before characterization, samples were soaked in hydrofluoric acid solution (5 vol%) for 30 s to remove the glass phase on the surface. The gas release behavior of the samples during the heating process was observed by thermogravimetric mass spectrometry (TG-MS, Thermo plus EV2, Japan). The chemical valence state of the element Fe within the samples was detected by X-ray photoelectron spectroscopy (XPS, Thermo Scientific K-Alpha, USA). The pore size dispersion coefficient D was derived as follows:
where di is the pore size of each pore, is the average pore size, and n is the number of pores counted (n = 100).
The compressive strength of the samples drilled to ϕ 45 mm × 55 mm was measured by an electronic universal testing machine (MTS E44, China) with a 1 mm/min loading speed. Each data point represents the average value of three replicate samples. The bulk density, apparent porosity and true porosity of the samples were measured using the Archimedes drainage method, according to the PRC national standard (GB/T 2997–2015). The true density (ρ0) of the samples was measured by a fully automated gas displacement method true density analyzer (Quantachrome Autosorb Ultra Pyc 1200e, USA). The water absorption was determined by the dry weight and the saturated water weight of the sample. The relevant parameters were derived as follows:
where ρb, q, P, and wz represent the bulk density, apparent porosity, true porosity, and mass water absorption, respectively. m1 is the dry weight of the sample, m2 is the suspension weight of the sample in water, m3 is the weight of the sample saturated with water, and ρw is the density of water at the test temperature.
3. Results and discussion
3.1. The effects of sintering temperature on foamed ceramics
displays the deformation behavior of the green body of the sample during heating from 350°C to 1250°C at 4°C/min and the body height variation. As can be observed from the figure, the height of the green body remained relatively constant as the temperature increased from 350°C to 984°C. However, the green body gradually shrinks with temperatures higher than 1030°C until its height reaches the minimum value at 1110°C, with a shrinkage of 12.37%. As the temperature increases, the green body gradually melts and softens. Then, the green body gradually expands, and the decomposition of the foaming agent SiC forms foams under the action of CO2 gas. The height reaches a maximum at 1148°C, increasing by 63.15%, and remains relatively stable at temperatures lower than 1222°C. The morphology of the sample projection exhibits good roundness at around 1170°C, but large pores significantly appear at 1222°C. It follows that the initial foaming temperature of the mixture powder prepared by CTs is approximately 1100°C, and the instability and collapse temperature of the foams is about 1170°C. Therefore, the experiment was designed to prepare foamed ceramic samples at 1110–1170°C to investigate the effect of sintering temperature on the fabrication and properties of foamed ceramics.
As seen in , with the sintering temperature increase from 1110°C to 1170°C, the total porosity of the sample grows sharply from 41.39% to 92.15%, and the apparent porosity shows a decreasing trend. This indicates that the increase in the sintering temperature leads to the formation of many closed pores in the sample. Due to the rapid increase in pores, the bulk density of the sample rapidly decreases. Specifically, the bulk density of the sample fired at 1110°C is 1.571 g/cm3, which is close to the density of sintered ceramics. Currently, the sample is in a semi-sintered state because the liquid phase has not filled the stacked voids between the powder particles, the apparent porosity is as high as 5.65%, and the mass water absorption is 3.61 wt.%. With a further increase in the sintering temperature to 1155°C, the apparent porosity of the sample decreased to a minimum value of 1.61% under the effect of sintering. Still, the bulk density decreased to 0.24 g/cm3, which led to an increase in the mass water absorption to 5.89 wt.% instead of a decrease.
Figure 3. The physical properties of samples with different sintering temperatures: (a) apparent porosity and total porosity, (b) bulk density and water absorption.
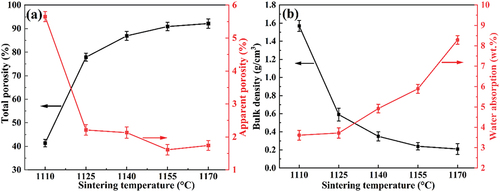
The pore morphology and pore size distribution of foamed ceramics prepared at different sintering temperatures are shown in . As can be seen from this figure, with increasing sintering temperature, the pore size and dispersion coefficient of the sample gradually increase, but the uniformity of the pores deteriorates. When the sintering temperature is 1110°C, many small pores with 0.18–0.42 mm diameters are formed inside the softening green body, and the average pore diameter is only 0.29 mm. As the sintering temperature increases, the amount of liquid phase in the green body gradually increases and the viscosity of the liquid phase decreases, reducing the growth resistance of the bubbles. Thus, the pore size and the roundness of the pores gradually increase, and the uniformity of the pores improves with a dispersion coefficient of 0.132 at 1140°C, as exhibited in . However, once the sintering temperature increased to 1170°C, the pores swelled obviously to 0.6–2.6 mm with an average pore size of 1.66 mm, and the dispersion coefficient increased to 0.269. The significant increase in pore size is attributed to the rapid coalescence of small pores with low viscosity in the body, which also brings connected pores and wrinkled pore walls, as shown in . This is the reason for the decrease in bulk density and the increase in water absorption. In addition, studies have revealed that the increased pore size and wrinkled pore wall significantly affect the mechanical properties of the sample. Therefore, as mentioned above, the sintering temperature of cyanide tailings foamed ceramics should not exceed 1170°C.
Figure 4. Pore morphology and pore size distribution of samples with different sintering temperatures: (a) 1110°C, (b) 1125°C, (c) 1140°C, (d) 1155°C, (e) 1170°C, (f) pore size distribution.
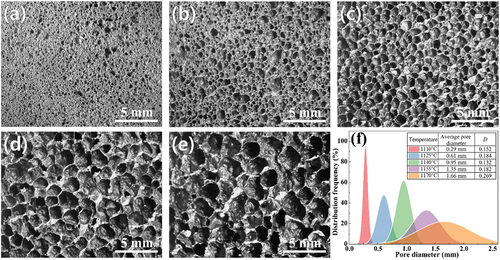
shows the phase composition of foamed ceramics sintered at different temperatures. As can be seen from the figure, the main crystalline phase inside the sample changes significantly as the sintering temperature increases. Specifically, samples fired at 1110°C are composed of a small amount of amorphous phase and a variety of crystalline phases, such as spinel (MgAl2O4), anorthite (CaAl2SiO8), albite (NaAlSi3O8), hematite (Fe2O3), and enstatite (Mg2Si2O6). With increasing sintering temperature, the diffraction peaks of the anorthite and albite phases, which both have a comparatively low melting point, gradually disappear and are replaced by a gradual increase in the diffraction peaks of the amorphous phases. The intensity of the diffraction peaks of spinel gradually increases. According to , only spinel crystalline diffraction peaks exist in the XRD pattern of the sample sintered at 1170°C. The simulation of XRD plots yielded 79.19%, 85.72%, 86.36%, 88.13%, and 92.15% amorphous phase content for 1110°C, 1125°C, 1140°C, 1155°C, and 1170°C samples, respectively. The melting of the lower melting point anorthite and albite phases produces a large liquid phase, forming a low-viscosity silicate melt, which not only reduces the resistance to bubble growth, leading to a significant increase in porosity and pore size, but also forms a large amount of amorphous glass phases during the cooling stage. In addition, cyanide is not detected in any of the samples sintered at 1110–1170°C, indicating that the cyanide is completely decomposed under the action of high temperature.
3.2. The effects of holding time on foamed ceramics
To investigate the effects of holding time on the properties of foamed ceramics, they were produced by holding at 1155°C for 20–80 min, and their bulk density, water absorption, total porosity, and apparent porosity were comparatively analyzed. As shown in , the total porosity of the sample increases from 1.02% to 2.20% with increasing holding time, prompting the bulk density to gradually decrease from 0.407 g/cm3 to 0.203 g/cm3. However, unlike the firing temperature, the apparent porosity increases with the extension of holding time. When the holding time exceeded 40 min, an obvious increase occurred in the apparent porosity, which led to an increase in the water absorption from 2.61 wt.% to 8.83 wt.%. The change in apparent porosity is related to the transformation of the pore structure. As shown in , the pore size of the sample gradually increases and the pore roundness decreases with increasing holding time. The evolution of the pore structure with the holding time should be attributed to the decomposition behavior of SiC in the foaming stage [Citation29]. Previous studies have revealed that the surface of SiC particles is covered with a layer of SiO2 oxide film before 1000°C, which hinders the decomposition of SiC. In the sintering and insulation stage, the SiO2 layer is gradually eroded by silicate melt formed in the body. Then, the CO2 gas generated from SiC decomposition is wrapped in the body, contributing to the rapid increase in pore size. The pore size increases slowly when the holding time exceeds 60 min, which indicates that SiC particles dispersed in the green body are almost completely decomposed at 1155°C for 60 min. However, due to the high surface energy of the foam system, the coalescence of pores will occur under excessive holding time. As shown in , many small holes appear in the pore wall as the holding time increases to 80 min, leading to a high apparent porosity. It follows that the holding time of foamed ceramics from CTs should not exceed 60 min when fired at 1155°C.
Figure 6. The physical properties of samples with different holding times: (a) total porosity and apparent porosity, (b) bulk density and water absorption.
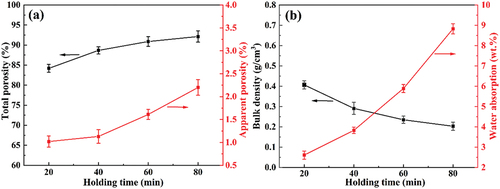
Figure 7. Pore morphology and SEM images of samples with different holding times: (a) 20 min, (b) and (e) 40 min, (c) 60 min, (d) and (f) 80 min.
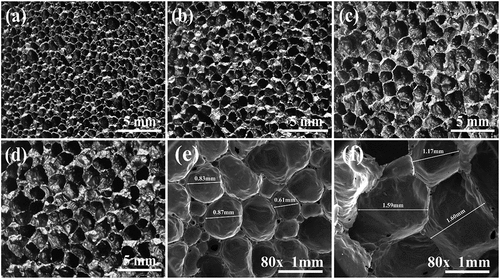
shows the XRD patterns of each sintered foamed ceramic at different holding times. As can be seen from the figure, all samples consist of spinel, anorthite, and albite. However, with the extension of the holding time, the diffraction intensity of the spinel phase gradually strengthens, while that of the anorthite phase gradually weakens. This indicates that different phases within the matrix continuously react with each other through solid-solid and solid-liquid reactions during the holding stage. However, the glass phase content of the blanks does not change significantly. The simulation of XRD plots yielded amorphous phase contents of 81.75%, 81.81%, 83.48%, and 83.43% for 20 min, 40 min, 60 min, and 80 min samples, respectively. This strongly indicates that the degree of SiC reaction at different holding times is the main reason for evaluating pore structure in the samples [Citation30]. With the extension of the holding time at the maximum temperature, the high-temperature liquid phases generated in the billet erode the SiC protective film more severely, increasing the degree of SiC reaction. As a result, the total porosity and pore size of the samples gradually increase, while the bulk density gradually decreases with the extension of the holding time.
3.3. The effect of heating rate on the foamed ceramics
The heating rate is a key processing parameter affecting the phase reaction in the green body [Citation31]. The change in the phase composition in the samples could further influence the pore structure and the properties of the foamed ceramics. According to the XRD pattern of the CTs (earlier depicted in ), they contain a large amount of pyrite, and the rate of FeS2 decomposition is related to the oxygen concentration and the reaction temperature. To further analyze the sintering process, the weight loss and thermal behavior of the green body were examined by TG-DTG-MS, where the MS curve tracked the volatilization of SO2 gas, as shown in . As can be seen from the figure, the thermogravimetry (TG) curve changed significantly with increasing temperature, and the total weight loss of the green body was 11.89%. From 25°C to approximately 300°C, a weight loss of about 1.47% can be observed on the TG curve that corresponds to an endothermic peak at approximately 215°C on the DTG curve, which could be attributed to the volatilization of free water and adsorbed water. As the firing temperature increased, an endothermic peak was generated at 340°C with a TG weight loss of 1.01%, possibly caused by the removal of bound water. From 400°C to approximately 600°C, a strong endothermic peak at 492°C is shown in the DTG curve with a TG weight loss of 2.21%, which may be caused by the decomposition of sulfur-containing minerals, such as iron sulfide, in the cyanide tailings. It is worth noting that the MS curve shows a pronounced peak at approximately 500°C and significant fluctuations above 800°C. This indicates that the sulfur-containing minerals in the green body decompose and release a large amount of SO2 gas at temperatures between 434°C and 552°C and above 806°C. The TG curve shows a maximum weight loss of 7.20% above 600°C, which may be caused by the decomposition of carbonates, as well as reactions or transitions within the mineral fraction. If SO2 gas is generated and discharged as the temperature exceeds 1096°C, the gas can be wrapped in the softened body as a foaming agent such as SiC. Therefore, the effect of the heating rate in the temperature range of 400°C to 600°C on the pore structure and properties of foamed ceramics needs to be investigated.
shows the longitudinal profile morphology and the average size of pores in each delamination of foamed ceramics prepared with different heating rates. As shown in , with increasing heating rate, the average pore size of the sample decreases and then increases, reaching a minimum of 0.88 mm when the heating rate is 4°C/min. In addition, as seen from the longitudinal profile morphology, the samples went from uniform pore size to the gradual phenomenon of smaller pore size at the bottom and larger pore size at the top. Finally, at a heating rate of 8°C/min, the deteriorated large pores appeared at the bottom. This could be related to the decomposition of FeS2 introduced by CTs. The decomposition of FeS2 is accompanied by a large amount of O2 consumption, leading to insufficient O2 concentration inside the bodies, especially in the bottom area, where it cannot fully contact the air. When the heating rates increase, the O2 in the air does not have enough time to penetrate the bottom of the body, limiting the decomposition of SiC during the foaming stage. Thus, the pore size at the bottom is smaller than that at the top, forming a graded pore structure in the samples.
Figure 10. Pore morphology and average pore size of samples with different heating rates: (a)1°C/min, (b) 2°C/min, (c) 4°C/min, (d) 8°C/min, (e) average pore.
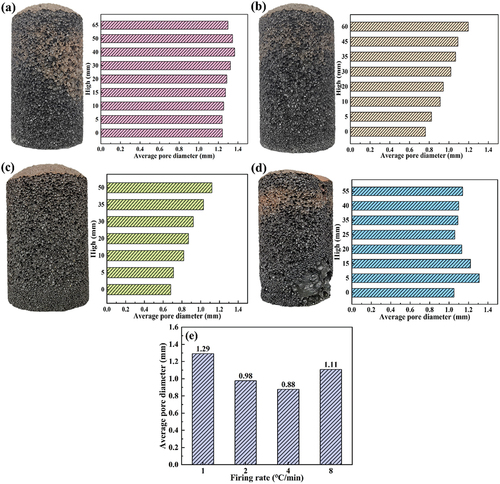
However, when the heating rate increases to 8°C/min, the concentrated decomposition of sulfur-containing minerals leads to a severe shortage of oxygen at the bottom, resulting in the transition of the Fe ion valence state. shows the Fe 2P XPS spectra of the bottom and top foamed ceramics made at an 8°C/min heating rate. The results show that the percentage of Fe2+/Fe3+ in the bottom sample is significantly greater than in the top sample. As a modifier of the aluminum-silicon melt network, the entry of Fe2+ into the melt will drastically reduce the melt viscosity [Citation32,Citation33]. This leads to the rapid expansion of gas bubbles to form large colossal pores, resulting in the deterioration of the pore structure of the sample and reduced performance. Meanwhile, this conclusion can be verified from the microstructure of the pores wall at the bottom and top of foamed ceramics, which were made at an 8°C/min heating rate, as shown in . It can be seen that the pore size in the bottom of the sample is significantly larger than that in the top of the sample. Besides, the grains distributed in the wall of large pores are significantly larger than those in the small pore wall, but the number of grains distributed in the large pore wall is less. This indicates that the more liquid phase generated at high temperature, the lower the viscosity of the melts system. Pores in the lower melts are easy to coalesce with each other, resulting in the formation of deteriorated large pores in the bottom sample, which is consistent with the conclusion above.
Figure 11. The Fe2P XPS spectra of samples at 8°C/min: (a) bottom of the sample, (b) top of the sample.
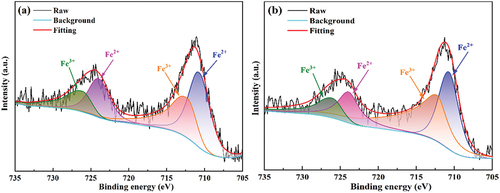
Figure 12. SEM images of the samples at 8°C/min: (a-c) top of the samples, (d-f) bottom of the samples.
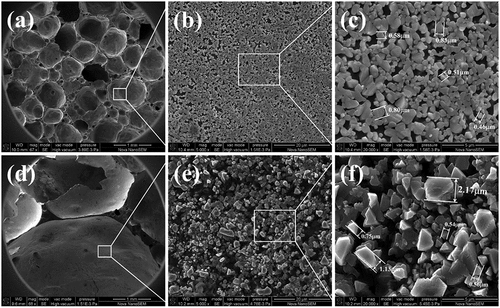
The change in pore structure with sintering rate also affects the physical properties of the sample. shows the bulk density, water absorption, apparent porosity, total porosity, and compressive strength of foamed ceramics fired at different heating rates. As shown in , when the heating rate increases from 1°C/min to 4°C/min, the total porosity of the sample decreases from 92.88% to 90.89%, which makes the bulk density increase to the maximum value of 0.235 g/cm3. Similarly, the apparent porosity decreases from 2.63% to 1.61% with increased heating rates, which gradually decreases the water absorption. The decrease in porosity and the average pore size, as well as the improvement in pore wall integrity, contribute to the improved mechanical properties of foamed ceramics [Citation34]. As seen from , the compressive strength of the samples increases significantly from 0.85 MPa to 2.71 MPa as the heating rate increases from 1°C/min to 4°C/min. However, once the heating rate increased to 8°C/min, the water absorption and apparent porosity increased due to the appearance of colossal pore structures in the samples. At the same time, the presence of the deteriorated large pores also reduces the compressive strength of the sample from its maximum value of 2.71 MPa to 1.45 MPa. Therefore, the heating rate in the 400–600°C temperature range should not exceed 8°C/min when preparing foamed ceramics with CTs.
4. Conclusions
In this study, foamed ceramic materials were successfully prepared by a high-temperature foaming process using cyanide tailings with high sulfur content as the main raw material and silicon carbide as the blowing agent. The effects of sintering temperature, holding time, and heating rate on the physical properties and pore structure of foamed ceramics were investigated. The following main conclusions were drawn:
The bulk density gradually decreases and the pore size gradually increases as the sintering temperature increases, which is mainly attributed to the melting of the feldspar phase with a low melting point and the increase in the high-temperature liquid phase, resulting in a significant increase in the total porosity of the foamed ceramics. At a sintering temperature of 1170°C, the pores of the sample undergo coalescence and the pore diameter range increases to 0.6 – 2.6 mm.
The total porosity and pore size gradually increase, and the bulk density gradually decreases with the extension of the holding time, which is mainly attributed to more severe erosion of the SiC protective film by the high-temperature silicate liquid phase in the green body. Thus, more SiC blowing agents play a foaming role in the high-temperature molten green body. At a holding time of 80 min, many holes appear in the pore wall of the sample, increasing apparent porosity and water absorption.
Within a temperature range of 400 – 600°C, different heating rates have a significant effect on the physical properties of foamed ceramics, which is mainly attributed to the decomposition of sulfur-containing minerals, such as FeS2, in cyanide tailings and the release of a large amount of SO2 gas in this temperature range. The faster heating rate results in the difficult oxidation of SiC at the bottom of the green body during the foaming stage due to the lack of oxygen concentration, so the bottom of the sample forms a smaller pore size. The reducing atmosphere is generated at the bottom of the green body even at a heating rate of 8°C/min, resulting in the presence of Fe ions in the form of Fe2+. This transformation drastically reduces the melt viscosity, and the deteriorated large pores appear at the sample bottom, leading to a significant reduction in compressive strength due to the deterioration of the pore structure.
Disclosure statement
No potential conflict of interest was reported by the author(s).
Additional information
Funding
References
- Dong K, Xie F, Wang W, et al. The detoxification and utilization of cyanide tailings: a critical review. J Clean Prod. 2021;302:126946. doi: 10.1016/j.jclepro.2021.126946
- Kyle JH, Breuer PL, Bunney KG, et al. Review of trace toxic elements (Pb, Cd, Hg, As, Sb, Bi, Se, Te) and their deportment in gold processing: Part II: Deportment in gold ore processing by cyanidation. Hydrometallurgy. 2012;111-112:10–21. doi: 10.1016/j.hydromet.2011.09.005
- Chen L, Li Q, Jiang T. Comprehensive utilization of tailings in quartz vein-hosted gold deposits. Minerals. 2022;12(12):1481. doi: 10.3390/min12121481
- Barcelos DA, Pontes FVM, Da Silva FANG, et al. Gold mining tailing: environmental availability of metals and human health risk assessment. J Hazard Mater. 2020;397:122721. doi: 10.1016/j.jhazmat.2020.122721
- Zhang Y, Li H, Yu X. Fe extraction from high-silicon and aluminum cyanide tailings by pretreatment of water leaching before magnetic separation. Trans Nonferrous Met Soc China. 2013;23(4):1165–1173. doi: 10.1016/S1003-6326(13)62579-0
- Dai X, Simons A, Breuer P. A review of copper cyanide recovery technologies for the cyanidation of copper containing gold ores. Miner Eng. 2012;25(1):1–13. doi: 10.1016/j.mineng.2011.10.002
- Long H, Li H, Pei J, et al. Cleaner recovery of multiple valuable metals from cyanide tailings via chlorination roasting. Sep Sci Technol. 2021;56(12):2113–2123. doi: 10.1080/01496395.2020.1812650
- Liu T, Lin C, Liu J, et al. Phase evolution, pore morphology and microstructure of glass ceramic foams derived from tailings wastes. Ceram Int. 2018;44(12):14393–14400. doi: 10.1016/j.ceramint.2018.05.049
- Liu T, Lin C, Liu P, et al. Preparation and characterization of partially vitrified ceramic material. J Non-Cryst Solids. 2019;505:92–101. doi: 10.1016/j.jnoncrysol.2018.10.019
- Dong K, Xie F, Wang W, et al. Calcination of calcium sulphoaluminate cement using pyrite-rich cyanide tailings. Crystals. 2020;10(11):971. doi: 10.3390/cryst10110971
- Roy S, Adhikari GR, Gupta RN. Use of gold mill tailings in making bricks: a feasibility study. Waste Manage Res. 2007;25(5):475–482. doi: 10.1177/0734242X07076944
- Mashifana T, Sithole T. Clean production of sustainable backfill material from waste gold tailings and slag. J Clean Prod. 2021;308:127357. doi: 10.1016/j.jclepro.2021.127357
- Gcasamba SP, Ramasenya K, Ekolu S, et al. A laboratory investigation on the performance of South African acid producing gold mine tailings and its possible use in mine reclamation. J Environ Sci Health. 2019;54(13):1293–1301. doi: 10.1080/10934529.2019.1642694
- Duan X, Meng F, Li Z, et al. Utilization of gold tailings for the construction of foamed ceramics used in external insulation buildings. Int J Appl Ceram Technol. 2022;19(4):2249–2258. doi: 10.1111/ijac.14018
- Wei Z, Zhao J, Wang W, et al. Utilizing gold mine tailings to produce sintered bricks. Constr Build Mater. 2021;282:122655. doi: 10.1016/j.conbuildmat.2021.122655
- Zhao B, Zhao L, Gao P, et al. Cyanide detoxification and iron mineral recycle of cyanide tailings by the sustainable utilization technology of oxidation-reduction roasting: process optimization and mechanism study. J Environ Chem Eng. 2023;11(3):109861. doi: 10.1016/j.jece.2023.109861
- Fu P, Li Z, Feng J, et al. Recovery of gold and iron from cyanide tailings with a combined direct reduction roasting and leaching process. Metals. 2018;8(7):561. doi: 10.3390/met8070561
- Hai L, Fang X, Zhao X, et al. Experimental analysis on cyanide removal of gold tailings under medium-temperature roasting. Sci Rep. 2023;13(1):3831. doi: 10.1038/s41598-023-28842-3
- Li L, Sun W, Hu W, et al. Impact of natural and social environmental factors on building energy consumption: based on bibliometrics. J Build Eng. 2021;37:102136. doi: 10.1016/j.jobe.2020.102136
- Hui T, Sun H, Peng T, et al. Recycling of extracted titanium slag and gold tailings for preparation of self-glazed ceramic foams. Ceram Int. 2022;48(16):23415–23427. doi: 10.1016/j.ceramint.2022.04.333
- Ge X, Zhou M, Wang H, et al. Effects of flux components on the properties and pore structure of ceramic foams produced from coal bottom ash. Ceram Int. 2019;45(9):12528–12534. doi: 10.1016/j.ceramint.2019.03.190
- Luo Y, Zheng S, Ma S, et al. Preparation of sintered foamed ceramics derived entirely from coal fly ash. Constr Build Mater. 2018;163:529–538. doi: 10.1016/j.conbuildmat.2017.12.102
- Cengizler H, Koç M, O Ş. Production of ceramic glass foam of low thermal conductivity by a simple method entirely from fly ash. Ceram Int. 2021;47(20):28460–28470. doi: 10.1016/j.ceramint.2021.06.265
- Wang H, Chen Z, Liu L, et al. Synthesis of a foam ceramic based on ceramic tile polishing waste using SiC as foaming agent. Ceram Int. 2018;44(9):10078–10086. doi: 10.1016/j.ceramint.2018.02.211
- Xia F, Cui S, Pu X. Performance study of foam ceramics prepared by direct foaming method using red mud and K-feldspar washed waste. Ceram Int. 2022;48(4):5197–5203. doi: 10.1016/j.ceramint.2021.11.059
- Chen X, Lu A, Qu G. Preparation and characterization of foam ceramics from red mud and fly ash using sodium silicate as foaming agent. Ceram Int. 2013;39(2):1923–1929. doi: 10.1016/j.ceramint.2012.08.042
- Zhou W, Zhang Z, Li N, et al. The preparation of mullite foamed ceramics reinforced by in-situ SiC whiskers and their reinforcement mechanism. Ceram Int. 2022;48(10):14224–14230. doi: 10.1016/j.ceramint.2022.01.310
- Martínez-Martínez S, Pérez-Villarejo L, Garzón E, et al. Influence of firing temperature on the ceramic properties of illite-chlorite-calcitic clays. Ceram Int. 2023;49(14):24541–24557. doi: 10.1016/j.ceramint.2022.11.077
- Liang B, Zhang M, Li H, et al. Preparation of ceramic foams from ceramic tile polishing waste and fly ash without added foaming agent. Ceram Int. 2021;47(16):23338–23349. doi: 10.1016/j.ceramint.2021.05.047
- Xiong H, Shui A, Shan Q, et al. The cause of foaming in polishing porcelain stoneware tile residues during sintering: interface corrosion of silicate-SiC. J Eur Ceram Soc. 2022;42(8):3660–3673. doi: 10.1016/j.jeurceramsoc.2022.02.060
- Fu F, Hu N, Ye Y, et al. Production of lightweight foam ceramics by adjusting sintering time and heating rate. Constr Build Mater. 2023;394:132063. doi: 10.1016/j.conbuildmat.2023.132063
- Wang J, Guo Q, Wei J, et al. Understanding the influence of iron on fluidity and crystallization characteristics of synthetic coal slags. Fuel Process Technol. 2020;209:106532. doi: 10.1016/j.fuproc.2020.106532
- Zhang L, Song X, Wei J, et al. Simulation and experimental study on the effect of iron on the structure and flow properties of coal ash slag. Chem Eng Sci. 2023;273:118642. doi: 10.1016/j.ces.2023.118642
- Dong Y, Guo W, Jiang C, et al. Using CaO as a modifier agent to optimize the pore structure of foamed ceramics from granite scrap. Ceram Int. 2023;49(9):13443–13451. doi: 10.1016/j.ceramint.2022.12.219