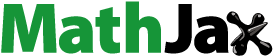
ABSTRACT
This study prepared ultralow electron-loss perovskites with spinel-like structures via a solid-state reaction. The (Mg0.95Co0.05)2(Ti0.95Sn0.05)O4 (MCTS) ceramic exhibited approximate values for ultrahigh quality factor, permittivity, and negative temperature coefficient (τf) of 310,000 GHz, 14.25, and −48.1 ppm/oC, respectively. To bring the τf closer to zero, a trace amount of (Ca0.95Sr0.05)(Ti0.97Sn0.03)O3 (CSTS) perovskite was added to MCTS during ceramic manufacturing. CSTS exhibited a permittivity of 92 and a large positive τf value of 810 ppm/°C. A 0.92MCTS–0.08CSTS hybrid ceramic sintered at 1325°C achieved remarkable microwave dielectric properties of εr ~18.42, Q×f ~ 237,000 GHz and τf ~3.72 ppm/°C. This mixed ceramic has significant potential for applications in manufacturing microwave devices.
1. Introduction
Zn2SnO4 has a spinel-type structure [Citation1–8], which is readily obtained via the solid synthesis of initial oxides or decomposition of appropriate double salts. It exhibits high thermal stability and is chemically and structurally characterized, having numerous microwave communication applications, such as patch antennas and wireless communication. However, dielectric substrates of microwave components are limited in their size, gain, and efficiency. When using microwave components, the following three dielectric properties of a material should be considered: low dielectric loss, permittivity, and temperature-independent resonant frequency, i.e. near-zero τf. Low-loss and high-performance ceramics have been extensively studied. For instance, the (1-x)[Li2Ti0.98Mg0.02O2.96F0.04 −1 wt%Nb2O5]-xLi3Mg2NbO6 ceramics with a near-zero τf have been reported [Citation9]. The phase composition, microstructure evolution, and microwave dielectric performance were determined as functions of x and the sintering temperature. Ultralow-loss Li3Mg3NbO7 ceramics with an orthorhombic rock-salt structure and optimized density (97.7%) have been prepared using a solid-state reaction method [Citation10]. The permittivity was mainly affected by the relative density and average ionic polarization, and the total quality factor and other microwave dielectric properties of perovskites were affected by losses, including conduction, radiation dielectrics, and surface waves. (ABO3), and spinel (A2BO4) are common structures in perovskite ceramics [Citation11,Citation12]; however, small amounts of additives have been mixed with ceramics to improve their microwave dielectric properties. In previous studies, two low-temperature sintering and high-performing microwave dielectric ceramics were prepared by adding small amounts of V2O5 and 0.6CuO–0.4B2O3 sintering additives to Li2Ti0.75(Mg1/3Nb2/3)0.25O3(LTMN0.25) [Citation13]. Furthermore, an improved (Mg0.95Co0.05)2TiO4 ceramic was prepared using a small amount of Co2+ instead of Mg2+ in a Mg2TiO4 perovskite [Citation14]. The quality factor Q × f of an (Mg0.95Co0.05)2TiO4 ceramic lies in the range 140,000–296,000 GHz; however this type of perovskite has a negative τf. Therefore, a (Ca1-xSrx)(Ti0.97Sn0.03)O3 perovskite material with a positive τf is selected for mixed-phase doping [Citation15]. Reportedly, the (Ca0.95Sr0.05)(Ti0.97Sn0.03)O3 (CSTS) ceramic has the following microwave dielectric properties: εr = 91.23, Q × f = 5200 GHz, and τf = 810 ppm/°C. Similarly, the microwave dielectric properties of (Mg0.95Co0.05)2(Ti0.95Sn0.05)O4 (MCTS) are as follows: εr = 14.25, Q × f = 310000 GHz, and τf = −48.1 pm/°C. To bring τf closer to zero, a hybrid ceramic, 0.92MCTS–0.08CSTS was formed by mixing CSTS and MCTS perovskites. This hybrid ceramic was used as the microwave ceramic substrate in an ISM band filter. Several analyses were performed using X-ray diffraction (XRD, Cu Kα at 40 kV and 40 mA, Siemens) method, scanning electron microscopy (SEM, Philips XL–40FEG), HP8757D network analyzer, and HP8350 sweep oscillator.
2. Experimental procedure
The (1-y)MCTS and yCSTS ceramics were prepared via a traditional solid-state reaction using CoO (>99.7%), TiO2 (>99.9%), CaO (>99.7%), MgCO3 (>99.7%), SrO (>99.7%), CaCO3 (>99.7%), and SnO2 (>99.7%) as raw materials. The decomposition reaction of MgCO3.xH2O within a temperature range of 300–700°C for 5 h can be represented as follows:
Based on this equivalent chemical composition, (1-y)MCTS and yCSTS powders were prepared based on the designed composition and mixed with zirconia balls and ethanol solution in a planetary grinder for 12 h. The prepared powders were dried and calcined in air within a temperature range of 1000–1100°C for 4 h before being mixed in the molar fraction of (1-y)MCTS and yCSTS and stirred for 12 h. Under a pressure of 20 MPa, the mixed powder containing 3 wt% and 10% polyvinyl alcohol (PVA 500, Showa, Japan) solution as an adhesive was pressed into oblate spheroid particles of diameter and thickness 1.1 and 0.5 cm, respectively. Thereafter, the pressed particles were sintered within a temperature range of 1250–1400°C for 4 h to form a (1-y)MCTS–yCSTS hybrid ceramic and cooled to room temperature in a furnace. The heating and cooling rates of heaters were set to 10 °C/min. Subsequently, XRD and SEM were used to analyze the crystal structure of the sintered ceramics and surface morphologies of the samples, respectively. The relative densities of the samples were estimated using the Archimedes method, whereas the microwave dielectric properties were measured using a network analyzer based on the Hakki – Coleman [Citation16] and Courtney [Citation17] methods. Furthermore, τf was estimated within a temperature range of 25–80°C as follows:
where f80 and f25 are the resonant frequencies at 80 and 25°C, respectively.
3. Results and discussion
The MCTS ceramic has a crystalline and spinel-like structure in the tetragonal P4_122 space group; however, it possesses two inequivalent (Mg0.95Co0.05)2 + sites. At the first site, an (Mg0.95Co0.05)2 + cation is bonded to six O2 − anions to form an (Mg0.95Co0.05)O₆ octahedra that shares corners with six equivalent (Mg0.95Co0.05)O₄ tetrahedral. The two edges of the octahedra are adjacent to equivalent (Mg0.95Co0.05)O₆ octahedra and other four edges are adjacent to equivalent (Ti0.97Sn0.03)O₆ octahedra. At the second site, an (Mg0.95Co0.05)2 + cation is bonded to four O2 − anions to form an (Mg0.95Co0.05)O₄ tetrahedra that share corners with six equivalent (Mg0.95Co0.05)O₆ octahedra or equivalent (Ti0.97Sn0.03)O₆ octahedra. Similarly, there exist two nonequivalent O2 sites. At the first site, O2 − is bonded to three (Mg0.95Co0.05)2 + cations and a (Ti0.97Sn0.03)4 + cation in a rectangular see-saw-like geometry. At the second site, O2 − is bonded to two Mg2 + cations and two equivalent Ti4 + cations in a distorted rectangular see-saw-like geometry. shows the crystal structure of the MCTS ceramic. shows the XRD patterns of the (1-y)MCTS–yCSTS ceramic sintered at 1325°C for 4 h. The type of secondary phase may depend on the ion diameter, dopant content, and sintering temperature. Secondary phases may influence the microstructure, chemical composition, and mechanical and electrical properties of the ceramics. The appearance of (Mg0.95Co0.05)(Ti0.95Sn0.05)O3 as secondary phases may degrade their microwave properties compared to MCTS. The XRD patterns revealed the presence of (1-y)MCTS and yCSTS hybrid phases in these samples. The XRD patterns of (1-y)MCTS–yCSTS ceramics sintered at 1325°C were similar for various y values. In , the peaks indicate the primary and secondary crystal phases of the (1-y)MCTS–yCSTS and (Mg0.95Co0.05)(Ti0.95Sn0.05)O3 ceramics, respectively. Different structures caused the formation of mixed phases in the (1-y)MCTS–yCSTS ceramic systems. shows the XRD of the 0.92MCTS–0.08CSTS ceramics sintered at various temperatures. CSTS perovskite is an orthorhombic structure in the orthorhombic Pnma space group, and a (Ca0.95Sr0.05)2 + cation bonds to eight O2 − anions in an 8-coordinate geometry. Contrastingly, a (Ti0.97Sn0.03)4 + cation bonds with six O2 − anions to form a corner-share (Ti0.97Sn0.03)O₆ octahedron. The following two unequal O2 sites are formed: (i) O2 − bonded to two equivalent (Ca0.95Sr0.05)2 + cations and two equivalent (Ti0.97Sn0.03)4 + cations in a 4-coordinate geometry, (ii) O2 − bonded to three equivalent (Ca0.95Sr0.05)2 + and two equivalent (Ti0.97Sn0.03)4 + cations in a 5-coordinate geometry. shows the absence of angular displacement in the 0.92MCTS–0.08CSTS ceramic.
Figure 2. X-ray diffraction patterns of (1-y)(Mg0.95Co0.05)2(Ti0.97Sn0.03)O4 - y(Ca0.95Sr0.05)(Ti0.97Sn0.03)O3 ceramics sintered at 1325°C for 4 h.
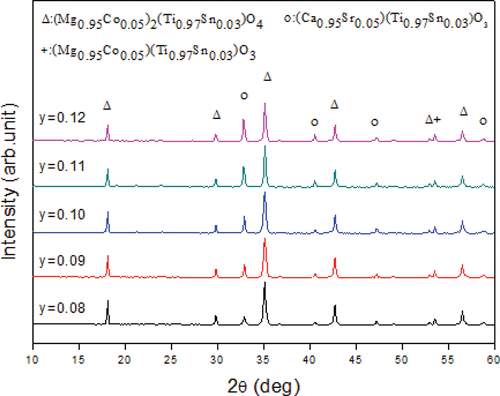
Figure 3. X-ray diffraction patterns of a 0.92(Mg0.95Co0.05)2(Ti0.97Sn0.03)O4-0.08(Ca0.95Sr0.05)(Ti0.97Sn0.03)O3 ceramic sintered at various temperatures.
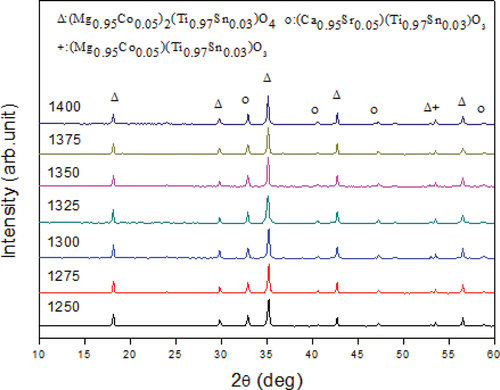
shows the SEM images of 0.92MCTS–0.08CSTS ceramics sintered at various temperatures. The increase in grain size is not significant when the sintering temperature ranges from 1250 to 1275°C. The uniform grains transform to non-uniform grains when the sintering temperature increases from 1375 to 1400°C. Furthermore, a decrease in pores at the grain boundaries can be observed as the sintering temperature increases from 1250 to 1300°C. An anoxic atmosphere is easily formed at such high temperatures, and the evaporation of SnO2, such as the reaction 2SnO2 (g) → 2SnO (g) + O2, may lead to a higher porosity [Citation18]. Pores at the grain boundaries decrease the ceramic density [Citation19]. The 0.92MCTS–0.08CSTS hybrid ceramics sintered at 1375°C were distinguished using the energy dispersive X-ray (EDX) analysis in combination with SEM (). shows the compositional data for regions A and B. The grain morphology of well-developed 0.92MCTS–0.08CSTS ceramics can be divided into the following two parts: MCTS of the large-grain B and CSTS of the small-grain A. The miscible forest materials of spinel-like and modified orthorhombic perovskite ceramics cannot form solid solutions. The EDS analysis in indicates that the crystal grain points A and B are analyzed as CSTS and MCTS, respectively, as shown in . The CSTS of grain A contains no traces of Mg and Co, and the MCTS of grain B contains no traces of Ca and Sr.
Figure 4. SEM micrographs of 0.92(Mg0.95Co0.05)2(Ti0.97Sn0.03)O4 - 0.08(Ca0.95Sr0.05)(Ti0.97Sn0.03)O3 ceramics sintered at various temperatures.
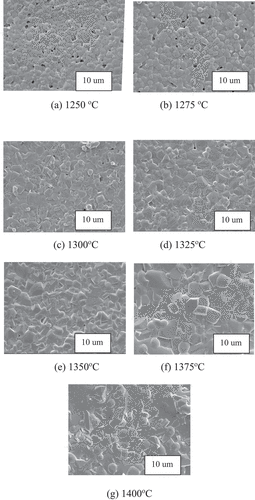
Figure 5. The marks of SEM for the 0.92(Mg0.95Co0.05)2(Ti0.97Sn0.03)O4 - 0.08(Ca0.95Sr0.05)(Ti0.97Sn0.03)O3 ceramics sinter at 1375°C.
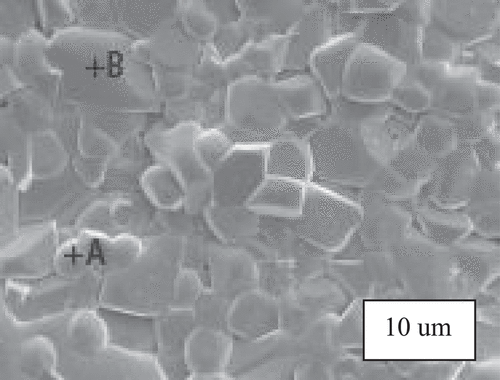
Table 1. EDX data of 0.92(Mg0.95Co0.05)2(Ti0.97Sn0.03)O4- 0.08(Ca0.95Sr0.05)(Ti0.97Sn0.03)O3 ceramics for areas A and B.
The Mg – Ti phase was present in the large-grain B. Compared to a pure MCTS ceramic, the CSTS – MCTS ceramic results in lower sintering temperatures owing to the smaller grain size of the CSTS perovskite, which aids in the densification of ceramics. shows the relative densities of (1-y)MCTS – yCSTS hybrid ceramics sintered at various temperatures for 4 h. As the sintering temperature increases up to 1325°C, the relative density increases to a maximum value of 98% (y = 0.08) and then decreases. shows the decrease in relative density owing to the abnormal growth of grains.
Figure 6. Relative density of (1-y)(Mg0.95Co0.05)2(Ti0.97Sn0.03)O4 - y(Ca0.95Sr0.05)(Ti0.97Sn0.03)O3 ceramics sintered at various temperatures.
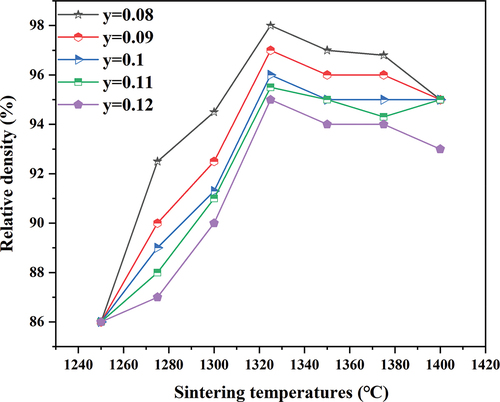
shows that the variations in permittivity and relative density are mutually consistent. When the sintering temperature increases to 1325°C, εr attains its maximum value before decreasing slightly. For the 0.88MCTS–0.12CSTS ceramic sintered from 1250 to 1325°C for 4 h, εr increased from 18.11 to a maximum of 21.77. presents the microwave dielectric properties of the (1-y)MCTS – yCSTS ceramics sintered at 1325°C for 4 h. As y increased from 0.08 to 0.12, the εr value of the (1-y)MCTS – yCSTS ceramic increased from 18.42 to a maximum of 21.77.
Figure 7. Dielectric constant curves of (1-y)(Mg0.95Co0.05)2(Ti0.97Sn0.03)O4- y(Ca0.95Sr0.05)(Ti0.97Sn0.03)O3 ceramics sintering at different temperatures for 4 hrs.
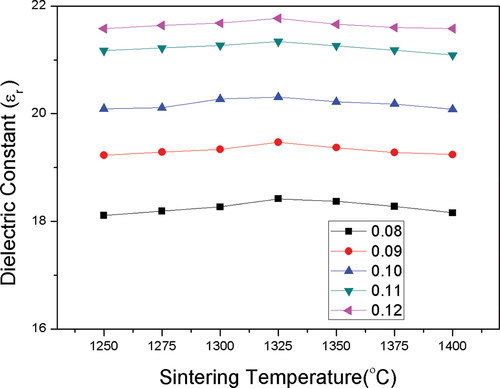
Table 2. Microwave dielectric properties of the (1-y)(Mg0.95Co0.05)2(Ti0.97Sn0.03)O4- y(Ca0.95Sr0.05)(Ti0.97Sn0.03)O3 ceramics sintered at 1325°C for 4 hrs.
The main causes of microwave dielectric losses include lattice vibration modes, porosity, density, secondary phases, impurities, and lattice defects. The factors minimizing the Q × f value can be categorized into internal and external losses. The former is caused by the interaction between polar phonon vibrations in crystals and microwave electric fields, whereas the latter includes ordered and disordered transitions, pore densities, grain sizes, oxygen vacancies, and impurity phases in the ceramics. The defect-free single crystal exhibited the highest intrinsic Q-value, which could be accurately described within the microwave frequency range using the classical damping oscillator model. Using the one-phonon absorption approximation, the model yielded the following approximate reciprocal relationship between Q × f and the dielectric:
where the frequency f should be approximately limited to the phonon engine frequency (1012 Hz) at room temperature for effective estimation. Reportedly, the extrapolation of EquationEq. (3)(3)
(3) can yield a satisfactory Q-value for well-machined ceramics at room temperature, ranging from microwave to megahertz frequencies that are 1–4 orders of magnitude lower than the optical phonon engine frequency. The experimental results yield the following relationship between Q × f and εr:
Therefore, the increase rate of εr in Q × f is fairly smooth compared to the Q × f values in EquationEq. (4)(4)
(4) , which can be attributed to external factors. Reportedly, porous dielectrics deteriorate the Q × f value, and the degree of their influence depends on the dielectric type.
For ceramics possessing low Q × f values of the order 103 GHz, the effect of porosity on Q can be described as , where Qo is the intrinsic quality factor measured by microwave reflectance spectroscopy and P is the porosity. However, for ceramics possessing high Q × f values of the order 105–106 GHz, such as polycrystalline Al2O3 ceramics, even a small amount of porosity can significantly reduce the Q value according to the following equation:
where Qo is the full-density dielectric quality factor 1.565 × 10−5, is a constant 6.3 × 10−3, and P is the porosity. shows the Q × f values of (1-y)MCTS–yCSTS ceramics sintered at different temperatures. These values increased till the sintering temperature attained a maxima of 1325°C and then decreased with increasing sintering temperature. A maximum Q × f value of 237,211 GHz was obtained for the 0.92MCTS–0.08CSTS ceramic sintered at 1325°C. The degradation of Q × f values can be attributed to abnormal grain growth at excessively high sintering temperatures, as shown in . Furthermore, the Q × f value decreases with increasing CSTS content, i.e. the y value increases. This is because the Q × f value of a CSTS ceramic is lower than that of an MCTS ceramic and less than 5,000 GHz.
Figure 8. Q×f values of (1-y)(Mg0.95Co0.05)2(Ti0.97Sn0.03)O4 - y(Ca0.95Sr0.05)(Ti0.97Sn0.03)O3 ceramics sintered at various temperatures for 4 h.
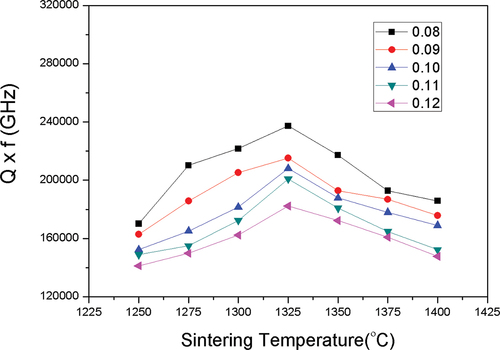
Reportedly, microwave dielectric loss is affected by the sum of the intrinsic and extrinsic losses. The former is caused by lattice vibration modes, crystal structure, and dielectric polarization, whereas the latter is caused by secondary phases, grain boundaries, oxygen vacancies, densification, and porosity. The relationship between the microstructure of a material and sintering temperature affects the properties of phase formation.
Liquid-phase sintering with glass additives is an inexpensive and effective method for reducing the sintering temperature. At lower sintering temperatures, the product content was low and the grain size was relatively small, indicating no phase formation. When the temperature increased to 1375°C, the size of each grain remained the same, i.e. a phase was formed. Additionally, the microstructure attained maximum densification, rendering obtaining the optimal Q × f value difficult.
As the sintering temperature continued to increase, the Q × f value decreased owing to excessive grain growth. As the CSTS content increased, the overall Q × f value decreased owing to the low Q × f value of the CSTS. The occurrence of the secondary phase (Mg0.95Co0.05)(Ti0.97Sn0.03)O3 reduced the Q × f value below that of (Mg0.95Co0.05)2(Ti0.97Sn0.03)O4.
shows the τf values of (1-y)MCTS–yCSTS ceramics sintered at various temperatures. The τf value was affected by material composition, additives, and second phase. Because the τf values of MCTS and CSTS were −47.5 and + 810 ppm/°C, respectively, the increase in CSTS content led to a more positive τf value. Hence, a zero τf value can be achieved by adjusting the CSTS content in the (1-y)MCTS–yCSTS ceramic. When the 0.92MCTS–0.08CSTS ceramic was sintered within a temperature range of 1250–1400°C for 4 h, the τf value was approximately 3.79 ppm/oC, which is close to zero, indicating good temperature stability. When the y value increased from 0.08 to 0.12, the τf value of the (1-y)MCTS–yCSTS ceramic varied from 3.79 to 22.22 ppm/°C, as shown in .
Figure 9. τf values of (1-y)(Mg0.95Co0.05)2(Ti0.97Sn0.03)O4 - y(Ca0.95Sr0.05)(Ti0.97Sn0.03)O3 system sintering at different temperatures for 4 h.
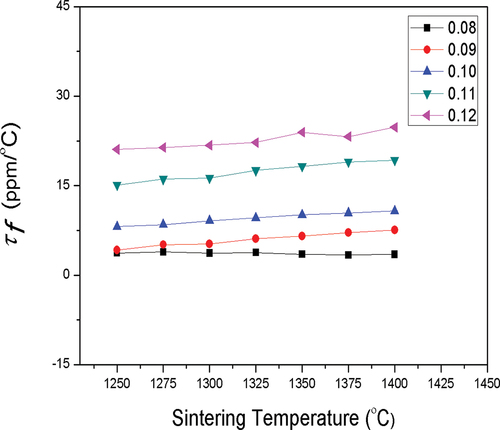
shows the physical layout of the designed filter with a center frequency of 2.4 GHz, and lists the simulation results. Compared with FR4 and alumina, using the 0.92MCTS–0.08CSTS ceramic as the filter reduced the size and insertion loss to 0.37 dB. This design method can facilitate electromagnetic simulators (HFSS) to accomplish filter design and determine the physical size of the filter.
Figure 10. Layout of a band-pass filter with a (1-y)(Mg0.95Co0.05)2(Ti0.97Sn0.03)O4 - y(Ca0.95Sr0.05)(Ti0.97Sn0.03)O3 ceramic substrate.
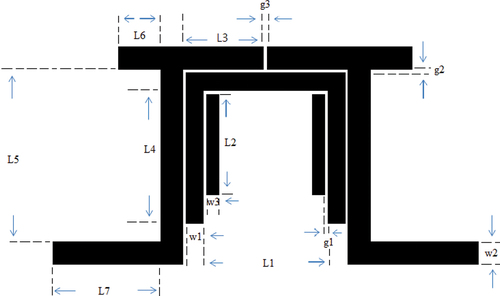
Table 3. Simulation results of the Band-Pass Filters Using Different Dielectrics.
4. Conclusions
This study incorporated CSTS into MCTS to form the (1-y)MCTS–yCSTS hybrid ceramic with an adjustable τf value and dielectric varying with y. The primary and secondary crystal phases were the (1-y)MCTS–yCSTS and (Mg0.95Co0.05)(Ti0.95Sn0.05)O3 ceramics, respectively. At a sintering temperature of 1325°C, the 0.92MCTS–0.08CSTS ceramics exhibited excellent microwave dielectric properties with the following parameters: permittivity ~ 18.42; Q × f ~ 237,211 GHz; τf ~3.79 ppm/°C. Therefore, the 0.92MCTS–0.08CSTS ceramic is suitable for fabricating microwave devices. The proposed dielectric combination has a negligible dielectric loss, and its dielectric and temperature characteristics render it promising for practical applications in microwave and next-generation communication systems.
Author contributions
YP: Preparation of materials, methodology, and software. Preparation of materials. Data curation and writing – original draft preparation. Writing – reviewing and editing. Software, theoretical calculation, and validation.
Disclosure statement
No potential conflict of interest was reported by the author(s).
Data availability statement
The data supporting the findings of this study are openly available to the reviewers.
References
- Park K, Seong JK, Kwon Y, et al. Influence of SnO2 addition on the thermoelectric properties of Zn1−xSnxO (0.01≤x≤0.05). Mater Res Bull. 2008;43(1):54–61. doi: 10.1016/j.materresbull.2007.02.018
- Ge NN, Liu CM, Cheng Y, et al. First-principles calculations for elastic and electronic properties of ZnSnO3 under pressure. Physica B. 2011;406(4):742–748. doi: 10.1016/j.physb.2010.11.046
- Li B, Luo L, Xiao T, et al. Zn2SnO4–SnO2 heterojunction nanocomposites for dye-sensitized solar cells. J Alloys Comp. 2011;509(5):2186–2191. doi: 10.1016/j.jallcom.2010.10.184
- Wang C, Xu B. Synthesis and optical absorption property of the Zn2TixSn1−xO4 (0≤x≤1) solid solutions. J Solid State Chem. 2004;177(10):3448–3453. doi: 10.1016/j.jssc.2004.05.024
- Kim HT, Kim Y, Valant M, et al. Titanium incorporation in Zn2TiO4 spinel ceramics. J Am Ceram Soc. 2001;84(5):1081–1086. doi: 10.1111/j.1151-2916.2001.tb00793.x
- Pal N, Paul M, Bhaumik A. New mesoporous perovskite ZnTiO3 and its excellent catalytic activity in liquid phase organic transformations. Appl Catal A Gen. 2011;393(1–2):153–160. doi: 10.1016/j.apcata.2010.11.037
- Chang YS, Chang YH, Chen IG, et al. Synthesis and characterization of zinc titanate doped with magnesium. Solid State Commun. 2003;128(5):203–208. doi: 10.1016/S0038-1098(03)00527-1
- Chaouchi A, Marinel S, Aliouat M, et al. Low temperature sintering of ZnTiO3/TiO2 based dielectric with controlled temperature coeffificient. J Eur Ceram Soc. 2007;27(7):2561–2566. doi: 10.1016/j.jeurceramsoc.2006.09.015
- Wang D, Li L, Du M. Ultra-low dielectric loss lithium-based, temperature stable microwave dielectric ceramics. Ceram Int. 2022 Jan;48(1):1394–1401. doi: 10.1016/j.ceramint.2021.09.225
- Cai C, Ma J, Xie J, et al. A novel Li3Mg3NbO7 microwave dielectric ceramic with ultra-low loss. Ceram Int. 2023 Aug 1;49(15):25495–25503. doi: 10.1016/j.ceramint.2023.05.090
- Zaman A, Uddin S, Mehboob N, et al. Structural investigation and improvement of microwave dielectric properties in Ca(HfxTi1-x)O3 ceramics. Phys Scr. 2021 Feb;96(2):025701. doi: 10.1088/1402-4896/abce74
- Goud JP, Kumar A, Alkathy MS, et al. Thickness dependence of microwave dielectric tunability in Ba0.5Sr0.5TiO3 thin films deposited by pulsed laser deposition. Ceram Int. 2023 Jan 1;49(1):1188–1194. doi: 10.1016/j.ceramint.2022.09.095
- Guo H-H, Zhou D, Du C, et al. Temperature stable Li2Ti0.75(Mg1/3Nb2/3)0.25O3-based microwave dielectric ceramics with low sintering temperature and ultra-low dielectric loss for dielectric resonator antenna applications. J Mater Chem. 2020;8(14):4690–4700. doi: 10.1039/D0TC00326C
- Belous A, Ovchar O, Durilin D, et al. High-Q microwave dielectric materials based on the spinel Mg2TiO 4. J Am Ceram Soc. 2006;89(11):3441–3445. doi: 10.1111/j.1551-2916.2006.01271.x
- Huang CL, Chen JY. High‐Q microwave dielectrics in the (Mg1−xCox)2TiO4 ceramics. J Am Ceram Soc. 2009;92:379–383.
- Hsu C-H, Chang C-H. Microwave dielectric properties of (Ca0.8Sr0.2)(SnxTi1−x)O3 ceramics. Mater Sci Eng B. 2013;178:354–357.
- Hakki BW, Coleman PD. A dielectric resonator method of measuring inductive capacities in the millimeter range. IRE Trans Microw Theory Tech. 1960;8(4):402–410. doi: 10.1109/TMTT.1960.1124749
- Courtney WE. Analysis and evaluation of a method of measuring the complex permittivity and permeability microwave insulators. IEEE Trans Microw Theory Tech. 1970;18(8):476–485. doi: 10.1109/TMTT.1970.1127271
- Liu XC. Synthesis, phase structure and dielectric property of the ZnO–MgO– SnO2 ceramics. J Alloys Comp. 2013;558:131–135. doi: 10.1016/j.jallcom.2013.01.021