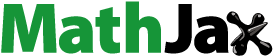
Abstract
The airflow behaviour and particle deposition before and after the maxillary expansion are evaluated using the computational fluid dynamics method. A pediatric patient was subjected to maxillary expansion and the CT scans of pre and post were recorded. The procured CT scans were developed into 3D models using Materialise MIMICS 21.0 (Materialise, Ann Arbor, MI). The analysis was performed using ANSYS FLUENT 2020 R2 with three different flow rates defined at the nostril inlet as 5, 10, and 15 LPM. The surface area and nasal volume increased by 5.1% and 16.5%, respectively, for the post-maxillary expansion case. The velocity profiles show a maximum value at the nasal valve regions followed by a decreased velocity in the mid-nasal region with an 85% lower velocity observed at the nasal valve for the post-maxillary expansion case. The nasal resistance and pressure drop decrease by 46% to 66% in post-maxillary expansion cases. The maximum WSS at the nasal valve region is observed which is reduced by around 47% for post-maxillary expansion cases. Particle deposition studies show a reduction in particle depositions in post cases indicating a greater number of inhaled particles reaching the lungs. The pressure drop and the nasal resistance values were reduced by more than 50% for post-maxillary expansion. The particle deposition studies show increased depositions at higher flow rates for both cases.
1. Introduction
The human nasal cavity is responsible for critical physiological functions. Apart from respiration which supports the very existence of life on earth, the nose carries out other functions such as the filtering of unwanted dust particles from reaching the lungs, thermal control, and humidification of the inspired air to the required conditions in addition to providing a sense of smell (Elad et al., Citation1993). Airway patency and dental malocclusions have always been studied under a plethora of scientific evidence. A constricted upper airway is significantly associated with a certain type of dental malocclusions and such patients fall under the high-risk category of developing obstructive breathing with a partially/completely collapsed upper airway, more commonly known as sleep-disordered breathing or obstructive sleep apnoea. One of the distinguishing features of a Class III malocclusion is maxillary hypoplasia with palatal constriction. Many authors have reported improvement in the upper airway characteristics following maxillary protraction via Dentofacial Orthopaedic growth modulation therapy. Protraction of the maxilla with simultaneous expansion using a face mask with Alt-RAMEC protocol has proven to be a successful treatment modality in Class III malocclusion with maxillary hypoplasia, in children within the age group of 9–12 years. There was a recorded increase in the length of the maxilla and mandible and the vertical height of the face. In addition to this, the maxillary forward position and the nasopharyngeal area saw a significant increase as a result of the treatment (Tuncer et al., Citation2009). A study conducted to evaluate the maxillary sinuses in growing subjects showed that sinuses develop early in females. The sinus grows particularly in the age groups of 9–14 years for males, whereas for females, it is from 6 to 11 years. Vertical development of the sinus was observed during the growth that resulted in an increased volume (Maspero et al., Citation2020). Researchers have also indicated that the increase in airway dimensions due to maxillary protraction may be minor in magnitude (Havakeshian et al., Citation2020). Yilmaz and Kucukkeles (Citation2014) evaluated airway measurements using CBCT after 9 weeks of Alt-RAMEC protocol in an airway study. They reported an enhancement in the upper airway and nasal width. Celikoglu and Buyukcavus (Citation2017) examined how different Alt-RAMEC protocols affected the airway after maxillary protraction and found that the effects of each protocol were similar. In 2018, a study (Büyükçavuş, Citation2019) used CBCT to investigate how the Alt-RAMEC protocol affected the airway. In this study, the RME protocol and the Alt-RAMEC protocol were compared in patients. They concluded that both groups’ increases in nasal and nasopharyngeal volume were comparable. Kaygisiz et al. (Citation2009) observed an improved airway due to face mask treatment which was retained up to 4 years post-treatment.
An adult approximately breathes about 10,000 to 20,000 L of air in a day which also brings enormous particulate matter like dust, smoke, and allergens along with various toxic and non-toxic aerosols (Guha, Citation2008). Occupational factors also lead to upper airway problems like sinusitis, nasal erosions, sinonasal cancers, and olfactory and vocal cord dysfunction (Zhao & Shusterman, Citation2012). The particle size largely determines the deposition patterns in the nasal cavity. Table shows the range of particles (Guha, Citation2008).
Table 1. Particle sizes (Guha, Citation2008)
Combustion of coal produces coal ash which is considered to be hazardous causing cancer and breathing difficulties like cough, breath shortness, respiratory infections, and allergies (Hagemeyer et al., Citation2019). Fly ash is a spherically shaped particulate matter with diameters ranging from 0.1 to 100 µm (Zierold & Odoh, Citation2020; Sarkar et al., Citation2005). The density of coal fly ash ranges from 1000 kg/m3 to 1780 kg/m3 (Bhatt et al., Citation2019).
CFD is widely used in predicting the airflow patterns in the complex nasal cavity. Additionally, particle deposition in the nasal cavity and also drug delivery-related applications have been using CFD for a very long time. Studies have been carried out by many researchers to understand the particle deposition and transport in the nasal cavity and also to understand the mechanisms and deposition patterns for the same (Dastan et al., Citation2014; Abouali et al., Citation2012; Bahmanzadeh et al., Citation2015; Zuber et al., Citation2020). Regional particle deposition is based on the significance of the nasal part under consideration and deposition requirements (Dong, Ma et al., Citation2018; Dong, Shang et al., Citation2018; Kiasadegh et al., Citation2020; Hazeri et al., Citation2021). The maxillary protraction procedure has reported subjective improvements in the breathing ability of children. There are no studies that help us evaluate the effect of this procedure on particle deposition patterns.
Studies have indicated a significant reduction in airway resistance due to rapid maxillary protractions in children (Iwasaki et al., Citation2014). Ghoneima et al. (Citation2015) in their CFD study described a reduction in nasal resistance along with pressure, velocity, and turbulent kinetic energy. They also found an increase in nasal cavity volume and nasopharynx volume as a result of the maxillary expansion. CFD airflow studies were carried out on 15 children who had undergone rapid maxillary expansion carried out due to a deviated septum resulting in a 50% reduction in wall shear stress (Chen et al., Citation2022). It is noted that there are many studies carried out to understand the airway dimensional changes and corresponding evaluation of flow parameters like the pressure, velocity, and wall shear stress as a result of rapid maxillary expansion, but there are no studies reported on the estimation of the particle deposition patterns. This work aims to fill this gap thereby adding novelty to this study. The current study investigates the microparticle deposition in the pediatric nasal cavity before and after a maxillary protraction protocol using CFD analysis. A comparison of the velocity, pressure, and particle deposition patterns is done with a normal and patent nasal cavity of a 12-year-old male. Steady-state simulation is carried out at 5, 10, and 15 LPM. This paper also reports the changes in airway dimensions, airflow patterns, and nasal resistance due to maxillary expansion in a patient-specific model.
2. Materials and methods
2.1. Details of patient
The Institutional Ethical Committee clearance was obtained for this study (Protocol Reference Number: 19,031). The study was carried out in the Department of Orthodontics and Dentofacial Orthopaedics, Manipal College of Dental Sciences, and the patient was a healthy subject with Class III Malocclusion and the treatment was done using Maxillary Protraction Appliances. An incisor end-to-end relationship, a Class III molar relationship, and a Wits evaluation of −1 mm all pointed to the person having a Class III malocclusion in their mixed or permanent dentitions.
The patient was treated with bonded RME appliance consisting of a hyrax screw (Leone Orthodontic Products, Sesto Fiorentino, Italy), acrylic cap, and metal framework with hooks for engaging extraoral elastics as shown in Figure . The activation protocol of the Hyrax screw in the AltRaMEC group was 1 mm per day (2 turns twice a day, 0.5 mm × 2 = 1 mm) for a week; for the succeeding week, the screw was closed at the same rate as the expansion schedule. A weekly check of the patient was done to check for the screw opening or closing. After this, the patients were asked to wear a Petit-type Face-mask for maxillary protraction for a minimum of 14 hours per day. The protraction in the Alt-RaMEC sample was considered completed when a full-cusp Class II molar relationship was achieved to account for relapse (Liou & Tsai, Citation2005). The total treatment time was 12± 2 months (average). Two sets of CBCT images were acquired using Planmeca ProMax 3D Mid CBCT (Planmeca, Helsinki, Finland), one before initializing the treatment (T0) and one after completion of treatment (T1).
2.2. Nasal cavity computational models
The works of Zubair et al. (Zubair, Abdullah et al., Citation2013; Zubair, Citation2012) provide details of the modelling and meshing of a nasal cavity. CT scans of patients are obtained for pre- and post-surgery cases and their details are provided in Table . The slice increment of the scanned image is 0.6 mm good enough to capture the intricate nasal cavity geometry as indicated by some of the researchers (Bailie et al., Citation2006).
Table 2. Patient specifics
In Table , P01A and P01B correspond to the same patient who had undergone an RME protocol and the P02 corresponds to the patient with a normal nasal cavity without any breathing-related difficulties. P02 was admitted for conditions of headache which required the scans to be taken and this study has utilized the nasal cavity information from the scans.
MIMICS (Materialise, Ann Arbor, MI) image processing software is utilised for CT image viewing and segmentation. The nasal cavity model is built by manually segmenting slice by slice using threshold values ranging from −1024 HU to −444 HU. The 3D model is then exported to 3-MATIC (Materialise, Ann Arbor, MI) for better visualization, and then further saved in .STL format to be exported to CATIA V6 where the final nasal cavity is generated and saved in .stp format. This is then exported to FLUENT (ANSYS2022 R1) for airflow and particle deposition simulations.
2.3. Flow field governing equations
The equations that determine the airflow include the continuity and momentum equations which essentially are the mathematical forms of conservation laws of physics, namely the mass and momentum. EquationEquations (1)(1)
(1) and (Equation2
(2)
(2) ) below are the continuity and momentum conservation equations
In the above equations, “u”, “ρ”, “v”, and “p” refer to the velocity of air, fluid density, kinematic viscosity, and pressure of the fluid, respectively.
Broadly, the nasal airflow can be classified as either laminar or turbulent in nature which is due to the Reynolds number which is defined as the ratio of inertial to viscous forces in the flow. Hahn et al. (Citation1993) studied the nasal airflow for a range of flow rates and observed disturbed laminar flow for flow rates of around 12 LPM. A half-nasal model flow analysis by Doorly et al. (Citation2008) showed a largely undisturbed laminar flow in much of the nasal cavity, though instability was observed in the nasal cavity at 12 LPM. Kleinstreuer and Zhang (Citation2003) studied the airflow in an oral cavity for different steady-state inspiration flows ranging from 15 LPM to 60 LPM and found that the flow becomes turbulent above 15 LPM and that the particle motions are influenced by the flow fluctuations at 60 LPM. It was also found that in comparison to turbulent dispersion alone, particle deposition is influenced by particle size and inhalation flow rates. Table provides researchers who have used laminar and turbulent models for different flow rates.
Table 3. Viscous models used for the different flow rates
2.4. Particle deposition equations
In the present study, the microparticles are tracked using the Lagrangian particle tracking approach. The individual particles are tracked in the flow field. In this type of particle tracking, the inertia, gravity, and diffusivity terms are considered directly making it advantageous. The Lagrangian equation that governs particle motion can be expressed as
where and
represent, respectively, the particle position and particle velocity.
The left-hand side of Equationequation 4(4)
(4) is the acceleration or the inertial force per unit mass. The term “F” represents all the forces that act on the particle, such as the drag force, gravity (buoyancy force) and additional forces like the Brownian and Saffman lift force.
In Equationequation 5(5)
(5) , only the gravity and drag force are considered for the present study. This study does not consider Brownian and Saffman lift forces as they are negligibly small. The force balance equation of the individual particle is integrated to find and carry out the Lagrangian particle tracking approach.
where up and ug are the velocity vectors for the particle and air respectively, ‘ρg’ and ‘ρp’ are fluid and particle densities, respectively. In Equationequation (6)(6)
(6) the drag force
(also written as
) for the unit particle mass and is defined as
where μg and dP are fluid dynamic viscosity and particle diameter, respectively. The Reynolds number for the particle Rep is defined as
is the coefficient drag force which is employed by the ANSYS FLUENT2022 R1 software from Morsi and Alexander (Citation1972).
In Equationequation 9(9)
(9) , a1, a2, and a3 reflect the constants that apply to smooth spherical particles throughout a wide range of
(Karakosta et al., Citation2015). The microparticles with an aerodynamic diameter ranging from 1 micron to 60 µm are considered in this study. It is assumed that the particles are spherical having a density of 1100 kg/m3 (Islam et al., Citation2021). The particles are injected into the nasal cavity through the nostril with the same velocity as that of inlet air velocity at the nostrils. The particles are assumed to be inert without any interaction between them. The injection type considered is the surface injection where the particles are released from each facet of the surface. It is assumed that the particles are deposited as they hit the wall. The forces involved in rotational and thermophoretic actions are assumed to be negligible. An ensemble of around 60,000 micro-sized particles ranging from 2 to 60 µm was uniformly injected from the nostrils. The particle number 60,000 is finalized based on the particle independence test as shown in Figure , which is carried out for 5 µm sized particles at 5 LPM.
Particle transport is assumed to be in a one-way coupling because the concentration of inhaled particles is dilute. Hence, the analysis of the nasal airflow is first carried out followed by the trajectory analysis for the particles. Cheng (Citation2003) observed that for particle sizes less than 0.5 µm, the inertial impaction mechanism predominates and the deposition depends on the Stokes number, whereas for particle sizes less than 0.5 µm the diffusion mechanism predominates and the deposition relies on the Schmidt and Reynolds numbers. Traditionally, the researchers have followed the Lagrangian approach for micron-sized particles as inertia forces govern and the Eulerian approach for nano-sized particles because the Brownian diffusion is the dominant mechanism (Abouali et al., Citation2012; Bahmanzadeh et al., Citation2015; Das et al., Citation2018; Hazeri et al., Citation2021; Abouali et al., Citation2012; Y Zhang et al., Citation2019). This study considers a steady flow condition for inspiration flow rates of 5, 10, and 15 LPM which is for the laminar nature of flow as per the literature studied.
2.5. Mesh generation and boundary conditions
The developed nasal cavity was imported into ANSYS FLUENT2022 R1 in .stl format and is followed by meshing activity. The accuracy of the numerical solution depends largely on the density and distribution of the mesh. The complex nasal airway was meshed with a polyhedral mesh with six prism layers instead of using only the tetrahedral mesh elements. The first layer height is maintained such that the y+ value is less than 1. The maximum skewness of the mesh was limited to 0.7. The meshing considers four mesh sizes named N1, N2, N3, and N4 which are indicated in Table . Figure shows a sample meshing in the mid-nasal region of the nasal cavity.
Table 4. Details of mesh
Mesh dependency is checked based on the velocity values along the nasal valve region. Figure shows the velocity values for different mesh densities and based on the works of Inthavong et al. (Citation2018), we can conclude the final mesh for numerical simulation as 3.436 million, 3.824 million, and 3.232 million for P01A, P01B, and P02, respectively.
At the nostrils, mass flow inlets corresponding to 5 LPM, 10 LPM, and 15 LPM which represent normal breathing conditions are applied. At the outlet which is at the end of the nasopharynx, the outflow boundary condition is applied because the velocity and pressure are unknown beforehand. The outflow boundary condition is based on the assumption of a fully developed flow. The air used in this simulation is incompressible in nature having a density of 1.225 kg/m3 (Cal et al., Citation2017). Additionally, the dynamic viscosity of 1.7894 × 10−5 kg/(ms) is assumed for simulations.
An uncoupled discrete-phase method of calculation is employed for particle tracking where the continuous flow field calculation is carried out which is followed by the particle trajectory calculations. This methodology is considered feasible when the discrete phase has low mass and momentum loadings where the continuous phase is not affected by the discrete phase. The trapped particles add up and give an account of the particle deposition which is defined according to deposition fraction (DF) or total deposition efficiency (TDE) in a specific location defined by Z Zhang et al. (Citation2005) which are defined below.
Summarizing the boundary conditions, this study uses mass flow at the nostril inlet and the outflow condition at the nasopharynx outlet. Nasal walls are considered with no slip, and the presence of mucus layer and nasal hair are excluded. For DPM, the nostrils and nasopharynx are set in the “escape” condition and the nasal walls are set in the “trap” condition.
The equations governing the flow use the control volume method for discretization, where the domain is discretized to control volumes. Integration of each of the equations in the computational mesh is done once the governing equations are converted to integral forms followed by solving for dependent variables which are pressure and velocity by setting up and solving the algebraic equations. A pressure-based solver in ANSYS FLUENT2022 R1 is used to solve the governing equations. Solving the momentum equation gives the velocity and the required pressure is solved to arrive at a required pressure field. A SIMPLE algorithm is used to relate velocity and pressure corrections and to enforce mass conservation and pressure fields. In an iterative method of the numerical solution, the residuals quantify the errors in the set of equations, thereby indicating the convergence which is typically considered to be a minimum of four orders of magnitude.
2.6. Numerical model validation
The computation models for P01A, P01B, and P02 are validated against published values. For particle sizes ranging from 2 µm to 60 µm, the total deposition efficiency values for a flow rate of 5 LPM are displayed against the impaction parameter.
Figure indicates the deposition efficiencies of the numerical models of this study are in line with the work done by researchers in the past. Minor deviations from the literature values can be attributed to the inherent anatomical variations that cannot be avoided. Hence, the numerical models can be used to analyse the flow parameters and the particle deposition patterns.
3. Results
3.1. Nasal airway geometry comparison
The maxillary expansion protocol makes the nasal airway undergo morphological changes which also affect the nasal airway volume and cross-sectional areas. In the present study, the surface area of the nasal cavity increased from 1.75 × 10−2 m2 for the pre-case to 1.84 × 10−2 m2 for the post-maxillary expansion case. This was about a 5.1% increase in the total surface area. The nasal volume for the pre- and post-case was 2.774 × 10−5 m3 and 3.326 × 10−5 m3, respectively, representing a 16.5% increase in the final volume.
For pre- and post-maxillary cases, the cross-sectional area at the nasal valve region is 9.5 × 10−5 m2 and 1.82 × 10−4 m2, respectively. The nasal valve region’s cross-sectional area for P02, a patent nasal cavity, is 1.42 × 10−4 m2. It should be noted that the nasal valve region’s cross-sectional area has risen by 1.9 times as a result of the maxillary expansion treatment. The nasal cavity’s anterior, middle, and posterior regions are indicated in Figure by the prefixes “A,” “M,” and “P,” respectively. Therefore, the values are assessed at three regions in the nasal cavity’s anterior portion, ten regions in the middle, and four regions in the posterior portion, as shown in Figure .
3.2. Velocity profiles
The velocity profiles of all three cases (P01A, P01B, and P02) at different cross-sections are indicated in the figures below for all three flow rates. Figure shows the velocity profiles across the nasal cavity at different cross-sections for a flow rate of 5 LPM and 15 LPM. The nasal valve experiences the highest velocity since it is the most restricted part of the nasal cavity. However, in our study, we have found that for the post-maxillary expansion case the peak velocity occurs somewhere between the nasal valve and the first plane of the mid-nasal region (between A3 and M1). However, the overall velocity distribution for P01B is lower compared to the P01A case due to the higher cross-sectional area achieved by the maxillary expansion. Additionally, it should be emphasised that in the normal nasal cavity as compared to the case of post-maxillary expansion, the velocity profiles at the various cross-sections are higher.
Figure 8. Velocity contours (a) P01A—5LPM, (b) P01B—5 LPM, (c) P02–5 LPM, (d) P01A—15 LPM (e) P01B—15 LPM, (f) P02–15 LPM.

The velocity profiles indicate an overall decrease in post-maxillary protraction cases when compared to pre-cases as shown in Figure . The nasal valve region has the highest velocity, which is followed by a drop in velocity as we move towards the nasopharynx region. The maximum velocity around the nasal valve region in post-maxillary expansion cases is reduced by about 1.8 times for all the flow rates. This indicates the positive results of the post-maxillary expansion which is intended as a part of nasal airway improvement. When compared to the case of post-maxillary expansion, the maximum velocity for the normal nasal cavity is roughly 1.5 times greater in the nasal valve region.
3.3. Nasal resistance and wall shear stress
Nasal Resistance is the ratio of the pressure difference between the nostrils and the nasopharynx to the flow rate in millilitres/sec. The nasal resistance values obtained for this study are shown in Table which show decreased nasal resistance due to maxillary protraction procedures.
Table 5. Nasal resistance
The post-maxillary expansion cases resulted in a decrease in nasal resistance values by 2.1, 2.5, and 2.1 times for 5 LPM, 10 LPM, and 15 LPM, respectively. Inferring Table , we see that the reduced nasal resistance supports the subjective reportage in literature with children citing positive results (Ghoneima et al., Citation2015). However, the normal nasal cavity exhibited a higher nasal resistance when compared to the post-maxillary expansion case which can be attributed to the anatomical considerations of the nasal cavity.
The pressure drop across the nasal cavity is plotted against the flow rates as shown in Figure . The results show that the pressure drop has decreased for the post-case by around 2.5 times which affects the calculated nasal resistance value. The figure also includes the pressure drop obtained by previous researchers for a 5-year-old male (Xi et al., Citation2012). It can also be noted that in the normal nasal cavity, P02 has a higher pressure drop when compared to the pre- and post-maxillary expansion cases. The variations in the pressure drop are due to the anatomical variations in the nasal cavity models considered in this study.
The variations of pressure along the length of the nasal cavity for a flow rate of 15 LPM are shown in Figure .
It can be noted from Figure that the pressure values show a decreasing trend along the nasal cavity which is a desirable outcome. Since the chest expands during inspiration and the lung’s internal pressure is lowered below that of the surrounding environment, a reducing value of pressure in the nasal passages is essential.
The maximum wall shear stress (WSS) values are plotted for the 15 LPM as shown in Figure . The figure shows a maximum WSS value in the mid-nasal region for all three cases. Higher wall shear stress indicates nasal irritation and discomfort in breathing. Figure also shows higher WSS in the pre-case when compared to post-maxillary expansion cases for their respective flow rates, which indicates the ease of breathing and better airflow in the nasal cavity.
3.4. Particle deposition results
The particle deposition studies are carried out for particle sizes ranging from 2 µm to 60 µm. The Impaction parameter is calculated which is a product of “Q” which is the flow rate through the nostril and “d2” which is the square of the particle diameter (IP = Q*d2). The impact parameter is plotted against the particle deposition fraction in the nasal cavity. The experimental data and numerical simulations by Schroeter et al. (Schroeter et al., Citation2011) and Bahmanzadeh et al. (Bahmanzadeh et al., Citation2015), respectively, are compared with the results obtained in this study as in Figure .
The particle deposition pattern is plotted against the particle diameter in Figure . Though the values are not exactly comparable, the deposition efficiency patterns are compared with the work done by past researchers on a 5-year-old child (Zhou et al., Citation2013). The differences in the deposition patterns can be possibly due to variations in the nasal anatomy since a 5-year-old nasal cavity model was used, and the present study deals with nasal cavities of the 11-year-old age group. Another possible reason may be the nasal replica that was used in the 5-year-old nasal cavity model which may have factors like surface roughness and manufacturing accuracy that impact the deposition patterns.
The variation of the total deposition efficiency based on the inlet mass flow rates is shown in Figure . It is evident from the plot that as the inlet flow rates increased the deposition efficiency increased for a given particle diameter. For 10 LPM, the particles over 45 µm show total depositions and for 15 LPM almost all particles over 40 µm exhibited total deposition.
4. Discussions
RME has been shown to significantly increase the forward growth of the maxilla, significantly reduce the forward growth of the mandible, and significantly rotate the mandible clockwise due to its effects on the craniofacial structures and upper-airway dimension (Hiyama et al., Citation2002). The post-palatal and nasopharyngeal airway dimensions can be widened in growing skeletal class III subjects with maxillary retrusion. By widening the airway, maxillary protraction may lower the risk of obstructive sleep apnea syndrome in children with maxillary retrusion (Ming et al., Citation2018).
This study primarily investigates the nasal airflow and nasal deposition patterns in the pediatric airway following a maxillary protraction protocol which is carried out as a part of airway improvement. The procured CT scans were developed to get a nasal airway geometry which was used to carry out airflow simulations using ANSYS FLUENT 2022 R1. The simulations were carried out in the laminar range of flows that are below 15 LPM. The meshing of the nasal airways is carried out by using a polyhedral meshing technique instead of a traditionally used tetrahedral and hybrid mesh. Polyhedral mesh has been widely used by many researchers recently and has the advantages of faster convergence, and minimised cell counts and computational time (Bass et al., Citation2019; Brüning et al., Citation2020).
The velocity profiles are assessed at various points within the nasal cavity, and they reveal a maximum velocity at the nasal valve region, followed by a decrease in velocity as the flow moves into the mid-nasal region. The maximum velocity in the nasal valve region is found to reduce by 85% for all the three flow rates as a result of airway improvement due to maxillary expansion. Also, the patent nasal cavity exhibited around 50% higher peak velocity at the nasal valve region when compared to the post-maxillary expansion case. Studies have shown that the post-maxillary expansion reduced the velocity by 40% and the pressure by 80% thereby indicating improved nasal airway ventilation (Iwasaki et al., Citation2012).
The pressure drop obtained across the nasal cavity was reported to be 0.807 Pa, 2.224 Pa, and 4.245 Pa for the post-maxillary expansion case for the flow rates of 5 LPM, 10 LPM, and 15 LPM, respectively, which were reduced, respectively, by around 46%, 40%, and 46% when compared to the pre-cases. The nasal resistances were consequently reduced for the post-maxillary cases by a similar margin which indicated ease of breathing. Researchers have found nasal resistance values ranging from 0.046 Pa.s/ml to 0.07 Pa.s/ml for a flow rate of 15 LPM (Garcia et al., Citation2007). The maximum WSS values plotted along the length of the nasal cavity indicate a higher value in the mid-nasal region contrary to the nasal valve region as indicated by many of the earlier researchers (Zubair, Abdullah et al., Citation2013).
The particle deposition studies indicate that some of the particles are trapped by the nasal cavity while remaining ones are transported to the lungs. This study shows higher particle depositions in post-maxillary expansion cases when compared to pre-cases. It can also be observed that with the increase in the flow rate, the particle depositions increase.
The study has some limitations. The study is carried out only at the laminar range of flows. Further studies shall include the range of airflows in both laminar and turbulent flows. Additionally, only the total nasal deposition is considered in this study. The regional deposition along with variable flow rates will be a future scope of the study.
5. Conclusions
The maxillary expansion protocol is performed in the pediatric population to improve and facilitate ease of nasal breathing. This study was used to examine the airflow patterns and particulate build-up in the nasal airways due to post-maxillary protraction performed in a 12-year-old male child. With the use of CFD and feasible computing systems, the simulations were performed which resulted in the following inferences.
The maxillary protraction protocol in the pediatric nasal airway resulted in an increase in the overall surface area and nasal airway volume by 5.1% and 16.5%, respectively. The larger surface area increases the contact of the inspired air with the inner nasal cavity, resulting in improved heating and humidification functions.
Consequently, there was an improvement in the velocity profiles across the nasal cavity where the velocity at post-case was reduced by 85% at the nasal valve region. A reduced velocity increases the time of contact of the inspired air which also aids in heating and humidification.
The nasal resistance which is an indicator of the work of breathing is reduced by 40% to 46% for post-maxillary expansion cases for the three flow rates of this study. An elevated nasal resistance is an indication of upper airway obstruction, and this procedure has drastically reduced the nasal resistance.
The maximum WSS is reduced by 47% in post-maxillary expansion cases at the nasal valve region. WSS is a measure of the frictional force created when moving air collides with the nasal wall, and higher frictional forces indicate irritation of blood vessels in the nasal cavity. Maxillary protraction has been found to significantly reduce WSS in the nasal cavity.
The particle deposition studies were conducted at normal and low activity breathing conditions below 15 LPM which showed an overall reduction in the deposition of inhaled particles in the nasal cavity in the post-maxillary expansion case.
The patent nasal cavity when compared to the post-maxillary expansion case exhibits a higher nasal resistance of the order of 3.5 times for 5 LPM and 4.5 times for 10 LPM and 15 LPM. Additionally, around 45% higher velocity is observed in the normal nasal cavity when compared to the post-maxillary expansion case.
The particle deposition studies show that more of the particles are passing through the nasal cavity into the lungs, which can have both merits and demerits. The disadvantage is that more dust particles are reaching the lungs, whereas this can be thought of as an advantage when considering nasal medications which show more drugs and medicines reaching the lungs.
This study was useful in predicting the airflow patterns confirming the positive effects of the intended use of maxillary expansion in children. Additionally, particle deposition studies provided insight into the particles deposited in the nasal cavity and the particles escaped into the lungs due to an enhanced nasal airway.
Author Contributions
MZ, JVC, JE – Conceptualization. JVC, JE, MZ – Methodology Development and modelling of the work. JE, SN – Identifying CT scans. JVC, PK, MZ – Analysis and processing data. MZ, SN – Supervision of project. JVC, MZ, PK, SN – Manuscript writing, reviewing, editing.
Availability of data and materials
Not applicable.
Ethical Clearance
This study is carried out after obtaining Institutional Ethical clearance (Protocol Reference Number: 19031).
Declarations
Competing interests
The authors declare that they have no competing interests.
Acknowledgements
The authors would like to thank the Department of Aeronautical and Automobile Engineering, Manipal Institute of Technology, Manipal Academy, Manipal, for the computing resources provided to carry out this work.
Disclosure statement
No potential conflict of interest was reported by the author(s).
Additional information
Funding
References
- Abouali, O., Keshavarzian, E., Farhadi Ghalati, P., Faramarzi, A., Ahmadi, G., & Bagheri, M. H. (2012). Micro and nanoparticle deposition in human nasal passage pre and post virtual maxillary sinus endoscopic surgery. Respiratory Physiology & Neurobiology, 181(3), 335–21. https://doi.org/10.1016/j.resp.2012.03.002
- Bahmanzadeh, H., Abouali, O., Faramarzi, M., & Ahmadi, G. (2015). Numerical simulation of airflow and micro-particle deposition in human nasal airway pre- and post-virtual sphenoidotomy surgery. Computers in Biology and Medicine, 61, 8–18. https://doi.org/10.1016/j.compbiomed.2015.03.015
- Bailie, N., Hanna, B., Watterson, J., & Gallagher, G. (2006). An overview of numerical modelling of nasal airflow. Rhinology, 44(1), 53–57.
- Bass, K., Boc, S., Hindle, M., Dodson, K., & Longest, W. (2019). High-efficiency nose-to-lung aerosol delivery in an infant: Development of a validated computational fluid dynamics method. Journal of Aerosol Medicine and Pulmonary Drug Delivery, 32(3), 132–148. https://doi.org/10.1089/jamp.2018.1490
- Bhatt, A., Priyadarshini, S., Acharath Mohanakrishnan, A., Abri, A., Sattler, M., & Techapaphawit, S. (2019). Physical, chemical, and geotechnical properties of coal fly ash: A global review. Case Studies in Construction Materials, 11, e00263. https://doi.org/10.1016/j.cscm.2019.e00263
- Brüning, J., Hildebrandt, T., Heppt, W., Schmidt, N., Lamecker, H., Szengel, A., Amiridze, N., Ramm, H., Bindernagel, M., Zachow, S., & Goubergrits, L. (2020). Characterization of the airflow within an average geometry of the healthy human Nasal Cavity. Scientific Reports, 10(1), 3755. https://doi.org/10.1038/s41598-020-60755-3
- Büyükçavuş, M. H. (2019). Alternate Rapid Maxillary Expansion and Constriction (Alt-RAMEC) protocol: A comprehensive literature review. Turkish Journal of Orthodontics, 32(1), 47–51. https://doi.org/10.5152/TurkJOrthod.2019.18021
- Cal, I. R., Cercos-Pita, J. L., & Duque, D. (2017). The incompressibility assumption in computational simulations of nasal airflow. Computer Methods in Biomechanics and Biomedical Engineering, 20(8), 853–868. https://doi.org/10.1080/10255842.2017.1307343
- Celikoglu, M., & Buyukcavus, M. H. (2017). Changes in pharyngeal airway dimensions and hyoid bone position after maxillary protraction with different alternate rapid maxillary expansion and construction protocols: A prospective clinical study. The Angle Orthodontist, 87(4), 519–525. https://doi.org/10.2319/082316-632.1
- Cheng, Y. S. (2003). Aerosol deposition in the extrathoracic region. Aerosol Science and Technology, 37(8), 659–671. https://doi.org/10.1080/02786820300906
- Chen, S., Wang, J., Xi, X., Zhao, Y., Liu, H., & Liu, D. (2022). Rapid maxillary expansion has a beneficial effect on the ventilation in children with nasal septal deviation: A computational fluid dynamics study. Frontiers in Pediatrics, Internet].[accessed, https://doi.org/10.3389/fped.2021.718735
- Das, P., Nof, E., Amirav, I., Kassinos, S. C., Sznitman, J., & Gurka, R. (2018). Targeting inhaled aerosol delivery to upper airways in children: Insight from computational fluid dynamics (CFD).Gurka R, editor. PLoS ONE, 13(11), e0207711. https://doi.org/10.1371/journal.pone.0207711
- Dastan, A., Abouali, O., & Ahmadi, G. (2014). CFD simulation of total and regional fiber deposition in human nasal cavities. Journal of Aerosol Science, 69, 132–149. https://doi.org/10.1016/j.jaerosci.2013.12.008
- Dong, J., Ma, J., Shang, Y., Inthavong, K., Qiu, D., Tu, J., & Frank-Ito, D. (2018). Detailed nanoparticle exposure analysis among human nasal cavities with distinct vestibule phenotypes. Journal of Aerosol Science, 121, 54–65. https://doi.org/10.1016/j.jaerosci.2018.05.001
- Dong, J., Shang, Y., Inthavong, K., Chan, H.-K., & Tu, J. (2018). Partitioning of dispersed nanoparticles in a realistic nasal passage for targeted drug delivery. International Journal of Pharmaceutics, 543(1–2), 83–95. https://doi.org/10.1016/j.ijpharm.2018.03.046
- Doorly, D. J., Taylor, D. J., & Schroter, R. C. (2008). Mechanics of airflow in the human nasal airways. Respiratory Physiology, 11. https://doi.org/10.1016/j.resp.2008.07.027
- Elad, D., Liebenthal, R., Wenig, B. L., & Einav, S. (1993). Analysis of air flow patterns in the human nose. Medical & Biological Engineering & Computing, 31(6), 585–592. https://doi.org/10.1007/BF02441806
- Garcia, G. J. M., Bailie, N., Martins, D. A., & Kimbell, J. S. (2007). Atrophic rhinitis: A CFD study of air conditioning in the nasal cavity. Journal of Applied Physiology, 103(3), 1082–1092. https://doi.org/10.1152/japplphysiol.01118.2006
- Ghoneima, A., AlBarakati, S., Jiang, F., Kula, K., & Wasfy, T. (2015). Computational fluid dynamics analysis of the upper airway after rapid maxillary expansion: A case report. Progress in Orthodontics, 16(1), 10. https://doi.org/10.1186/s40510-015-0085-x
- Guha, A. (2008). Transport and deposition of particles in turbulent and laminar flow. Annual Review of Fluid Mechanics, 40(1), 311–341. https://doi.org/10.1146/annurev.fluid.40.111406.102220
- Hagemeyer, A. N., Sears, C. G., & Zierold, K. M. (2019). Respiratory health in adults residing near a coal-burning power plant with coal ash storage facilities: A cross-sectional epidemiological study. International Journal of Environmental Research and Public Health, 16(19), 3642. https://doi.org/10.3390/ijerph16193642
- Hahn, I., Scherer, P. W., & Mozell, M. M. (1993). Velocity profiles measured for airflow through a large-scale model of the human nasal cavity. Journal of Applied Physiology, 75(5), 2273–2287. https://doi.org/10.1152/jappl.1993.75.5.2273
- Havakeshian, G., Koretsi, V., Eliades, T., & Papageorgiou, S. N. (2020). Effect of orthopedic treatment for class iii malocclusion on upper airways: A systematic review and meta-analysis. Journal of Clinical Medicine, 9(9), E3015. https://doi.org/10.3390/jcm9093015
- Hazeri, M., Faramarzi, M., Sadrizadeh, S., Ahmadi, G., & Abouali, O. (2021). Regional deposition of the allergens and micro-aerosols in the healthy human nasal airways. Journal of Aerosol Science, 152, 105700. https://doi.org/10.1016/j.jaerosci.2020.105700
- Hiyama, S., Suda, N., Ishii-Suzuki, M., Tsuiki, S., Ogawa, M., Suzuki, S., & Kuroda, T. (2002). Effects of maxillary protraction on craniofacial structures and upper-airway dimension. The Angle Orthodontist, 72(1), 43–47. https://doi.org/10.1043/0003-3219(2002)072<0043:EOMPOC>2.0.CO;2.
- Inthavong, K., Chetty, A., Shang, Y., & Tu, J. (2018). Examining mesh Independence for flow dynamics in the human nasal cavity. Computers in Biology and Medicine, 102, 40–50. https://doi.org/10.1016/j.compbiomed.2018.09.010
- Islam, M. S., Larpruenrudee, P., Hossain, S. I., Rahimi-Gorji, M., Gu, Y., Saha, S. C., & Paul, G. (2021). Polydisperse aerosol transport and deposition in upper airways of age-specific lung. International Journal of Environmental Research and Public Health, 18(12), 6239. https://doi.org/10.3390/ijerph18126239
- Iwasaki, T., Saitoh, I., Takemoto, Y., Inada, E., Kanomi, R., Hayasaki, H., & Yamasaki, Y. (2012). Improvement of nasal airway ventilation after rapid maxillary expansion evaluated with computational fluid dynamics. American Journal of Orthodontics and Dentofacial Orthopedics, 141(3), 269–278. https://doi.org/10.1016/j.ajodo.2011.08.025
- Iwasaki, T., Takemoto, Y., Inada, E., Sato, H., Suga, H., Saitoh, I., Kakuno, E., Kanomi, R., & Yamasaki, Y. (2014). The effect of rapid maxillary expansion on pharyngeal airway pressure during inspiration evaluated using computational fluid dynamics. International Journal of Pediatric Otorhinolaryngology, 78(8), 1258–1264. https://doi.org/10.1016/j.ijporl.2014.05.004
- Karakosta, P., Alexopoulos, A. H., & Kiparissides, C. (2015). Computational model of particle deposition in the nasal cavity under steady and dynamic flow. Computer Methods in Biomechanics and Biomedical Engineering, 18(5), 514–526. https://doi.org/10.1080/10255842.2013.819856
- Kaygisiz, E., Tuncer, B. B., Yüksel, S., Tuncer, C., & Yildiz, C. (2009). Effects of maxillary protraction and fixed appliance therapy on the pharyngeal airway. The Angle Orthodontist, 79(4), 660–667. https://doi.org/10.2319/072408-391.1
- Kelly, J. T., Prasad, A. K., & Wexler, A. S. (2000). Detailed flow patterns in the nasal cavity. Journal of Applied Physiology, 89(1), 323–337. https://doi.org/10.1152/jappl.2000.89.1.323
- Keyhani, K., Scherer, P. W., & Mozell, M. M. (1995). Numerical simulation of airflow in the human nasal cavity. Journal of Biomechanical Engineering, 117(4), 429–441. https://doi.org/10.1115/1.2794204
- Kiasadegh, M., Emdad, H., Ahmadi, G., & Abouali, O. (2020). Transient numerical simulation of airflow and fibrous particles in a human upper airway model. Journal of Aerosol Science, 140, 105480. https://doi.org/10.1016/j.jaerosci.2019.105480
- Kleinstreuer, C., & Zhang, Z. (2003). Laminar-to-turbulent fluid-particle flows in a human airway model. International Journal of Multiphase Flow, 29(2), 271–289. https://doi.org/10.1016/S0301-9322(02)00131-3
- Liou, E. J.-W., & Tsai, W.-C. (2005). A new protocol for maxillary protraction in cleft patients: Repetitive weekly protocol of alternate rapid maxillary expansions and constrictions. The Cleft Palate-Craniofacial Journal, 42(2), 121–127. https://doi.org/10.1597/03-107.1
- Maspero, C., Farronato, M., Bellincioni, F., Annibale, A., Machetti, J., Abate, A., & Cavagnetto, D. (2020). Three-dimensional evaluation of maxillary sinus changes in growing subjects: A retrospective cross-sectional study. Materials (Basel), 13(4), 1007. https://doi.org/10.3390/ma13041007
- Ming, Y., Hu, Y., Li, Y., Yu, J., He, H., & Zheng, L. (2018). Effects of maxillary protraction appliances on airway dimensions in growing class III maxillary retrognathic patients: A systematic review and meta-analysis. International Journal of Pediatric Otorhinolaryngology, 105, 138–145. https://doi.org/10.1016/j.ijporl.2017.12.013
- Morsi, S. A., & Alexander, A. J. (1972). An investigation of particle trajectories in two-phase flow systems. Journal of Fluid Mechanics, 55(2), 193. https://doi.org/10.1017/S0022112072001806
- Sarkar, A., Rano, R., Mishra, K. K., & Sinha, I. N. (2005). Particle size distribution profile of some Indian fly ash—a comparative study to assess their possible uses. Fuel Processing Technology, 86(11), 1221–1238. https://doi.org/10.1016/j.fuproc.2004.12.002
- Schroeter, J. D., Garcia, G. J. M., & Kimbell, J. S. (2011). Effects of surface smoothness on inertial particle deposition in human Nasal Models. Journal of Aerosol Science, 42(1), 52–63. https://doi.org/10.1016/j.jaerosci.2010.11.002
- Segal, R. A., Kepler, G. M., & Kimbell, J. S. (2008). Effects of differences in nasal anatomy on airflow distribution: A comparison of four individuals at rest. Annals of Biomedical Engineering, 36(11), 1870–1882. https://doi.org/10.1007/s10439-008-9556-2
- Tuncer, B. B., Kaygisiz, E., Tuncer, C., & Yüksel, S. (2009). Pharyngeal airway dimensions after chin cup treatment in Class III malocclusion subjects. Journal of Oral Rehabilitation, 36(2), 110–117. https://doi.org/10.1111/j.1365-2842.2008.01910.x
- Wen, J., Inthavong, K., Tu, J., & Wang, S. (2008). Numerical simulations for detailed airflow dynamics in a human nasal cavity. Respiratory Physiology & Neurobiology, 161(2), 125–135. https://doi.org/10.1016/j.resp.2008.01.012
- Xi, J., Berlinski, A., Zhou, Y., Greenberg, B., & Ou, X. (2012). Breathing resistance and ultrafine particle deposition in Nasal–Laryngeal airways of a Newborn, an infant, a child, and an adult. Annals of Biomedical Engineering, 40(12), 2579–2595. https://doi.org/10.1007/s10439-012-0603-7
- Yilmaz, B. S., & Kucukkeles, N. (2014). Skeletal, soft tissue, and airway changes following the alternate maxillary expansions and constrictions protocol. The Angle Orthodontist, 84(5), 868–877. https://doi.org/10.2319/092713-705.1
- Zamankhan, P., Ahmadi, G., Wang, Z., Hopke, P. K., Cheng, Y.-S., Su, W. C., & Leonard, D. (2006). Airflow and deposition of nano-particles in a human Nasal Cavity. Aerosol Science and Technology, 40(6), 463–476. https://doi.org/10.1080/02786820600660903
- Zhang, Z., Kleinstreuer, C., Donohue, J. F., & Kim, C. S. (2005). Comparison of micro- and nano-size particle depositions in a human upper airway model. Journal of Aerosol Science, 36(2), 211–233. https://doi.org/10.1016/j.jaerosci.2004.08.006
- Zhang, Y., Shang, Y., Inthavong, K., Tong, Z., Sun, B., Zhu, K., Yu, A., & Zheng, G. (2019). Computational investigation of dust mite allergens in a realistic human nasal cavity. Inhalation Toxicology, 31(6), 224–235. https://doi.org/10.1080/08958378.2019.1647315
- Zhao, Y. A., & Shusterman, D. (2012). Occupational rhinitis and other work-related upper respiratory tract conditions. Clinics in Chest Medicine, 33(4), 637–647. https://doi.org/10.1016/j.ccm.2012.09.004
- Zhou, Y., Xi, J., Simpson, J., Irshad, H., & Cheng, Y.-S. (2013). Aerosol deposition in a Nasopharyngolaryngeal Replica of a 5-year-old child. Aerosol Science and Technology, 47(3), 275–282. https://doi.org/10.1080/02786826.2012.749341
- Zierold, K. M., & Odoh, C. (2020). A review on fly ash from coal-fired power plants: Chemical composition, regulations, and health evidence. Reviews on Environmental Health, 35(4), 401–418. https://doi.org/10.1515/reveh-2019-0039
- Zubair, M. (2012). Review A critical overview of limitations of CFD modeling in Nasal airflow. Journal of Medical and Biological Engineering, 32(2), 77. https://doi.org/10.5405/jmbe.948
- Zubair, M., Abdullah, M. Z., & Ahmad, K. A. (2013). Hybrid Mesh for Nasal airflow studies. Computational and Mathematical Methods in Medicine, 2013, 1–7. https://doi.org/10.1155/2013/727362
- Zubair, M., Riazuddin, V. N., Abdullah, M. Z., Ismail, R., Shuaib, I. L., Hamid, S. A., & Ahmad, K. A. (2010). Airflow inside the nasal cavity: Visualization using computational fluid dynamics. Asian Biomedicine, 4(4), 657–661. https://doi.org/10.2478/abm-2010-0085
- Zubair, M., Riazuddin, V. N., Abdullah, M. Z., Rushdan, I., Shuaib, I. L., & Ahmad, K. A. (2013). COMPUTATIONAL FLUID DYNAMICS STUDY OF PULL AND PLUG FLOW BOUNDARY CONDITION ON NASAL AIRFLOW. Biomedical Engineering: Applications, Basis and Communications, 25(4), 1350044. https://doi.org/10.4015/S1016237213500440
- Zuber, M., Valerian Corda, J., Ahmadi, M., Satish Shenoy, B., Anjum Badruddin, I., E. Anqi, A., Arifin Ahmad, K. M., Abdul Khader, S., Lewis, L., Anas Khan, M., & Kamangar, S. (2020). Investigation of coronavirus deposition in realistic human Nasal Cavity and impact of social distancing to contain COVID-19: A computational fluid dynamic approach. Computer Modeling in Engineering & Sciences, 125(3), 1185–1199. https://doi.org/10.32604/cmes.2020.015015