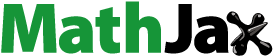
Abstract
Despite previous efforts to detect concentrated stresses in structures with holes, a complete understanding of structures that contain compound openings in addition to screw holes in critical stress areas is incomplete, especially for structures that are subject to sudden collapse. This study aims to conduct a laboratory test program to simulate a suddenly collapsed tank that contains compound holes in addition to the screw holes distributed on the cleaning opening located in the critical stress area. Six laboratory simulated models with two repeats for each are tested to represent the stages of implementation of the cleanout opening and to find out the causes of the sudden failure of the tank. Significant reduction in resistance when having a centre hole compared to the control plate. The importance of the stiffeners is evident in this region, as the resistance decreased slightly compared to the control plate by adding reinforcement plates on both sides. The resistance drop remains almost constant despite the stiffening panels being punctured on both sides with the same diameter as the main hole, which confirms the continued importance of the stiffeners at this stage. An improvement in resistance was observed when a cap was added to one of the holes in the stiffeners. A slight change in resistance was also observed when the cap was pierced with another hole to weld the cleaning pipe due to the failure remaining within the screw holes surrounding the main hole. In conclusion, the safety factor should be increased in areas that contain compound openings.
PUBLIC INTEREST STATEMENT
The study of the presence of openings inside the facilities is very important, especially when they are in high-stress areas, and in particular the compound openings, i.e. more than one opening in the facilities that are likely to be exposed to sudden failure. The aim of this article is to simulate the sudden failure of a water tank containing complex orifices in the zone of greatest stress, where more than one sample containing different composite openings was examined in order to know the tolerance of each sample. It was found that rearranging the compound openings affects the bearing resistance of the tank.
1. Introduction
On 19 February 2017, the date of the failure of the steel water tower tanks at Al-Majar. The water tank is a steel cylinder water tank of diameter () and height (
). Al-Majar is a city in Maysan Governorate, southern Iraq, approximately 24 km from the centre of Maysan. The city’s population reached approximately 110 thousand people, and it is located on the banks of the Majar River, to which the city is named. Al-Majar is a city that is considered the largest district in Maysan Governorate.
The Iraqi Ministry of Construction, Housing and Municipalities/General Directorate of Water issued a ministerial order on 9 April 2017 for the formation of a technical committee to find out the reasons for the failure of this water tower tank, which is considered as a part of Al-Majar water project plant of capacity 2000 m3/hour. The second author of this work is a part of this technical committee.
On 16 April 2017, the committee visited the collapsed reservoir site in Al-Majar to visually inspect the scattered remaining steel parts and investigate the position of the failure crack as shown in Plate 1. The committee also visited the other standing identical water tower tank located in another adjacent district named Qalaat Saleh, as shown in Plate 2.
During the site visit, a meeting was held between the committee and representatives of the resident engineer department to discuss the matter and collect information. The committee requested detailed documents, construction plans and work progress, properties of the materials used, design calculations, pictures throughout the phases of execution, and some important dates to diagnose the reasons for failure.
Due to missing the detailed design drawings and calculations, on 18 May 2017, the manufacturer was contacted via e-mail, asking him to specifically answer whether the sufficient detail (Manway/Cleanout) as shown in Plate 2 was done according to the manufacturer’s plans and specifications provided that the answer is supported by engineering drawings plans and design calculations with official technical details. The committee also asked the manufacturer to determine the amount of the safety factor used in Manway/Cleanout. Where Plate 2 represents the Manway/Cleanout photo of Qalaat Saleh Reservoir, which is approximately identical to Al-Majar tanks in this respect.
On the same date, the manufacturer conducted a preliminary review of the sent pictures (Plate 2), and his answer was as follows. The Manway/cleanout shown in Plate 2 is not as supplied by us. There is a modification/replacement carried out by unauthorized parties without the express approval of the manufacturer.
Administrative correspondence among the client representatives indicates that cracks in the plate near the Manway/cleanout appeared approximately 2 months from the date of tank commenced into service, as shown in Plate 3. It was checked out that the weather was typical on 19 February 2017, the date of the collapse of Al-Majar reservoir from the official weather institute. During the site visit, the executing company made a hole about 20 cm in diameter at the bottom of the tank to solder a cleaning tube irregularly and without the design firm’s consent and a consultant, as shown in Plate 4. The same pipe soldiering but with flange was used in other identical tanks. Fortunately, a plate (cleanout plate) containing the opening and the soldered pipe was found during the site visit, and it was found that there was a main vertical crack, as shown in the accompanying pictures Plate 3.
After studying the failure’s reasons, the technical committee believes that the failure occurred because the executing company made a hole in the cleanout plate in the most significant stress zone in an irregular manner without the manufacturer’s approval and consultant supervision. This hole is implemented inside the original opening covered by the cleanout plate from both sides. The execution company did not know the subject of the stress concentration. The execution procedure was horrible, with no scientific and engineering protocol. No approved detailed design drawing. Without knowing the value of the design’s safety factor used in the design of the tank tower, the hole is made. As the committee also concludes that the water tower tank’s safety factor was not sufficient.
Identical structure collapse based on the literature was examined. The three most disasters are as follows. In the first event without warning, in January 1919, molasses surged over Boston, and a frightful flood devastated a vast area of the city Tide (Arora et al., Citation2011). The 2.3 million gallon Boston molasses tank was only 3-year old at the time of failure. The tank was 50 feet tall and 90 feet in diameter. The tank was made of 15 mm thick steel plates. Before the explosion, the tank’s owner painted it brown and made it challenging to see molasses leaking. Failure investigations revealed that the possible cause was the sudden temperature change as the temperature on the previous day was −17°C and on the day of occurrence was 4.5°C. The design was inadequate to withstand the pressure created by expanding molasses, and the factor of safety used was considered low CALLISTER Jr (CALLISTER, Citation2000).
In the second event, over 5000 Liberty ships and T-2 tankers were mass-produced during World War II Zhang,b (Cerit, Citation2019). Of these, 1000 suffered significant failures; some broke into two between 1942 and 1946 because of low temperature, while 200 suffered severe fractures between 1942 and 1952. The welded ships’ failure rate was statistically astronomical in the North Atlantic, while none existed in the South Pacific Zhang,a (Faur et al., Citation2014).
De Havilland Comet, the world’s first commercial jetliner, went into service in 1952 is the third disaster. In contrast to propeller-based planes, the comet flew above the weather eight miles up in the stratosphere. It provided a quiet and smooth ride with a fully pressurized cabin previously unheard of in commercial aviation. Comet carried 28,000 passengers with a total of 104 million miles during its first year of operation Withey (GRIFFITH, Citation1920). Comet had square windows, and U.S. Civil Aeronautics Administration has refused to grant it an airworthiness certificate to fly in the United States. After only 18 months of service, two aircraft disappeared within 3 months of each other. The confidence of the public in the Comet was undiminished until 10 January 1954, when a Comet departing from Roma plunged into the sea from an altitude of 26,000 ft. After a great deal of effort, the wreckage was salvaged, which suggested that the cabin itself had failed. The conclusion was that the failure was due to explosive decompression of the cabin Arora et al. (Inglis, Citation1913).
It is known that Kirsch G. early identified the phenomena of stress concentration in 1898 Kirsch (Irwin, Citation1948) which was proved that the stress subjected to the infinite plate containing a circular hole becoming triple at the tip of the hole. The study of elliptical holes in tension strips by Inglis (Kashkoli et al., Citation2019) has focused on the severity of a crack in a structure. Griffith in 1920 (Kirsch, Citation1898) considered the propagation of cracks in the glass and developed the right ideas for crack growth. He formulated that an existing crack will grow provided its growth lowers the total energy of the system. His work was not noticed at that time due to the World War’s exigencies and the following depression worldwide. Griffith could not coin a convenient parameter for predicting the failure load of a component through the growth of a crack. Extension of Griffith’s ideas for brittle solid to ductile high strength materials was done by Irwin (Kostinakis & Morfidis, Citation2017). Irwin’s theory’s primary focus is on the crack tip rather than the crack; by moving the analysis to the crack tip, Irwin devised workable parameters like stress intensity factor and energy release rate. After that, Airy’s stress function and Westergaard’s stress function are used to solving the problem that contained hole and cracks and finds the stress concentration and stress intensity factors.
Experiments and numerical simulations for under water explosion phenomena were done by Lee et al. (Lee et al., Citation2021), the underwater pressures, accelerations, velocities, and strains by shock waves were measured, and also the whipping deformations of the ship were explored.
A series of studies concerning the dynamic behaviour of stress concentration, corrosion concurrent with stress concentration, circular and elliptical holes in cylindrical pressure vessels, and the effect of multiple holes on the stress concentration (Srinivasan et al. (Li & Chen, Citation2020), Magnucki et al. (Li et al., Citation2003), F. M. Li et al. (Luo et al., Citation2012), Zirka (Magnucki et al., Citation2002), Makulsawatudom et al. (Makulsawatudom et al., Citation2004), Troyani et al. (Rebora & Vernassa, Citation2020) Luo et al. (Srinivasan & Lehnhoff, Citation2001), Faur et al. (Tao et al., Citation2020), Kostinakis et al. (Tide, Citation2003), Cerit (Troyani et al., Citation2005), Kashkoli et al. (Withey, Citation1997), Tao et al. (Zhang, Citation2016a), Rebora et al. (Zhang, Citation2016b), F. Li et al. (Zirka & Chernopiskii, Citation2003)). They reached in their studies to an identical conclusion on the importance of stress concentration problem in structural analysis. Despite the efforts in finding the stress concentration in structural member with a hole in different geometries that have been developed and coded before many decades, the full understanding of the stress analysis of structures with compound openings still lacks, especially for complex structural sudden failure mechanisms. The objective of this study is through using the full experimental program in modelling the stresses in the cleanout plate of the collapsed water tower steel tank in the presence of the compound openings. This experimental simulation is used to understand the reasons for sudden collapse better and capture more accurate and reliable conclusions for the influence of creating hole inside openings cleanout plate in the critical stress zone of the steel tower water tank. An attempt through this paper is to assess the manufacturer’s safety factor in the design of the tank. The concentration of stresses in compound openings, especially in sensitive structures that are subject to sudden collapses, has become necessary to study them intensively.
2. Water tower definition
The water tower tank is a steel cylindrical uniform geometry along with the height. The cross-sectional internal diameter is approximately 12 m with 35 m approximate height, as shown in Figures . The cylindrical tank consists of 25 steel plate rings with constant geometry but variable thickness starting with 9.5 mm for the first bottom ring till 3.4 mm for the top ring, as shown in Figure . The Manway/cleanout locates at the first bottom ring where the hoop stresses are maximum. The diameter of the hole used as Manway is approximately 80 cm, as shown in Figure . This hole is located at the bottom plate, where the hoop stresses are significantly large in this zone. The absence of such a large hole led absolutely to change the region to discontinue region where the stresses are magnified. The manufacturer adds two octagonal plates on each side to compensate for the strength losses due to the hole. The octagonal plate thickness is 9.5 mm, equal to the thickness of the primary metal plate. The hole is covered with a steel plate cap of a diameter of approximately 90 cm. The octagonal plates and the cap plate are fixed throughout an appropriate number of bolts. The bolt diameter used is approximately 1 cm. The bolts’ arrangement around the octagonal plate’s perimeter and the cap plate is shown in Figure .
As mentioned in the introduction, after the tank’s commencement operation, and the tank is approximately full of water, the crack initiation started and grew. After 2 months, the crack thickness becomes visible. With the bad treatment from the execution company using basic welding techniques, this crack became a failure crack. The crack was found out after the sudden collapse of the tank. Based on that observation, the failure crack was vertical. Hence, vertical crack is imitated by the effect of tensile stress by a hoop concept. The crack happened in the discontinue region at the Manway/cleanout steel plate. The crack extended from the hole passed through the cap plate and the octagonal plates.
3. Experimental program
The experimental program is built in this paper to simulate the Manway/cleanout plate in a model under hoop tensile stresses. The program contained six specimens (Plate 1 to Plate 6) it means six treatments. For each treatment, two repeats are used to separate the random error in the experiment. The plate geometry and configuration of the Manway/cleanout with cap plate and the additional hole that represented the execution company’s hole are shown in Figure . The thickness of the all-metal plate is 6 mm, and the yielding strength is 350 MPa. Several strain gages in the form of the rosette are used in the experimental program to simulate the biaxial stress state at different positions. The reason for choosing the number and position of strain gauges is based on the critical region and location based on experience dependent on most of the critical areas in which it can be installed. The locations of the strain gauges in the plates are shown in Figure . Plate 1 is a solid plate that represents the control specimen. Three strain gauges (G1-1, G1-2, and G1-3) are pasted on this plate. A circular hole of 40 cm was created in Plate 2, identical to the control plate. Two strain gauges (G2-2 and G2-3) are pasted on plate 2. Plate 3 is equivalent to Plate 2, with central octagonal strengthening plates fixed from both sides. Five strain gauges (G3-1 from both sides, G3-2, and G3-3 from both sides) are pasted on this plate. Plate 4 is similar to Plate 3, with a central hole in both octagonal strengthening plates. Two strain gauges (G4-1 and G4-2) are pasted on this plate. Plate 5 is equivalent to Plate 4 with a central circular cover plate from one side of the hole in the octagonal strengthening plates. Four strain gauges (G5-1, G5-2, and G5-3 from both sides) are pasted on this plate. Finally, Plate 6 is similar to Plate 5 with a side circular hole in the central cover plate. Four strain gauges (G6-1, G6-2, and G6-3 from both sides) are pasted on this plate. The bolt configuration is arranged for the octagonal plates and the cap plate, as shown in Figure . The specification of used bolts is 6 mm diameter and yielding strength of 250 MPa. The summary plate configuration is shown in Table .
Table 1. Plate configurations
The experimental runs are conducted at Al-Nahrain University, Civil Engineering Laboratory. An automatic Tensile Machine of capacity 2000 tones is used to apply the hoop tensile stresses to plates until failure. Rosette strain gauges are used to simulate the biaxial state of stresses generated due to the compound openings in plates, as in Figure . Three strain directions can be measured for each one strain gauge. The at 0° position,
at 45° position and
at 315° position. The strain gages are passed on plates according to pasting protocol with appropriate tools and cohesion paste as in Figure . A strain meter of 10 channels is used as a data logger of TML origin, which is identical to the strain gauge brand used in this experiment.
4. Results and discussion
To separate the random error, two repeats of each plate are tested until failure under identical conditions. The ultimate load and the corresponding displacement for each sample with two repeats are recorded in Table . The mean and the standard deviation of ultimate strength readings are used to quantify the experimental error. Table also shows the failure pattern of different plate levels. The entire load–elongation curve for two repeats of each type of plate is drawn in Figure . It is observed that the standard error of the experiment for the two repeats of each type of plate is not significant. This fact is also clear in Figure for the entire behavior. The mean value of the ultimate strength is considered for further discussion.
Table 2. Ultimate load and the corresponding displacement with repeats
The result of the analysis indicates that a decrease of 38.54% in the ultimate strength of plate 2 (Plate 2 is identical to Plate 1 with a central circular hole) to the control plate (Plate 1). This decrease in resistance is due to the presence of a central circular hole in the plate. This phenomenon is in line with what was mentioned concerning the concentration of stress phenomenon given by Kirsch (Citation1898), while only a 27.78% decrease is observed in the ultimate strength of plate 3 (Plate 3 is identical to Plate 2 with central octagonal strengthening plates from both sides) relative to the control plate (Plate 1). This difference is due to the presence of octagonal stiffening plates on both sides of the hole, which helps to reduce the stress concentration, as in plate 2. This result confirms the importance of the stiffeners in the areas of stress concentration.
Despite making an opening in the stiffeners with the same diameter as the original hole in the plate as in the model of the fourth plate (Plate 4 is identical to Plate 3 with a central hole in both octagonal strengthening plates), the amount of resistance is only reduced by 20.67% compared to the control panel. This also indicates the importance of strengthening plates, although there is an opening on both sides equal to the one to be supported.
In the fifth examination of the fifth panel (Plate 5 is identical to Plate 4 with a central circular cover plate from one side of the hole in the octagonal strengthening plates), for adding a cover on one side to the slot in the stiffeners, we notice an improvement in performance and the decrease in resistance is only 15.48% compared to the resistance of the control plate. This confirms the participation of the cap for some stress centered on the hatch.
The same behavior between the sixth and fifth models was observed when examining the last sixth plate, although there was a hole inside the cover used in the sixth model. Failures in both models were seen through the screw holes that were used to attach the stiffeners to the motherboard.
The strain analysis is conducted based on the rosette strain gauge concept in a different critical position for each plate as in Figure to catch the biaxial stress behaviour. Rosette strain gauge consists of three strain gauge (,
,
) oriented at 0°, 45° and 315° with x-axis, respectively. Knowing that the x-axis coincides with the longitudinal axis of the plate as shown in Figure . The biaxial stress state (
,
,
) can be obtained based on the measured strains as:
,
and
. The recorded strain gauge for each position of each plate with the load level is written in Table and is graphed in Figure for some important strain readings.
Table 3. Strain field, µε
The results of the strain measurement according to Table and Figure show that the sensor readings in the three locations match up to the 160 kN load limit in plate 1, after which the values of the readings change according to the position with an almost constant increase, where the highest value of strain readings was observed in the sensor installed in the middle of the panel.
In specimen No. 3, an approximate match was found between the strain readings at positions 2 and 3 with a load limit of 180 kN, while the strain readings at position 1 (center) were very large compared to the two above-mentioned sites.
The largest strain reading was observed in plate 5 at the location of sensor 3 (installed on the tip of the hole), while the largest strain reading was recorded in plate 6 at position 2. From all the results of the above strain field, we conclude that the values of the maximum strains and their position change in the regions of concentration of stresses and change whenever the system of reinforcement of the openings is also changed or the addition of another hole. It is recommended to extend the study throughout a comprehensive numerical analysis using either finite element method or boundary element method.
4.1. Conclusions
The result of experimental analysis for the sudden collapse of steel water tower tank due to compound openings in critical zone yields the following conclusions:
Significant decrease in maximum resistance of about 40% due to the presence of a central circular hole (the difference in resistance between Panel 1 and Panel 2).
Adding stiffeners (reinforcement octagonal plate) on both sides, as in Panel No. 3, improves the behavior of the specimen and reduces the drop from 40% to nearly 30%.
Maintain approximately the same decrease value in the ultimate strength even with making holes in the stiffeners (protective reinforcement octagonal panels) on both sides with the same main hole diameter, as in Panel No. 4.
An improvement in the decrease of the maximum resistance by almost 15% compared to the control plate (Plate 1), due to the addition of a cover to one side of the holes in the stiffeners as in panel 5.
Maintaining the same drop in resistance despite the presence of a hole inside the cover used in plate No. 5 as found from the tested plate No. 6.
The onset of failure in most panels with compound holes starts from the screw holes and this indicates the use of a low safety factor in the design.
The results of the strain reading confirm the presence of a biaxial strain field, as this strain field is affected by its location to the openings and by the change of the openings system.
Disclosure statement
No potential conflict of interest was reported by the author(s).
Additional information
Funding
Notes on contributors

Hussam K. Risan
Hussam K. Risan born in Basra, Iraq, a PhD doctorate in civil engineering from Al-Nahrain University, Baghdad, Iraq, in 2013 had been obtained, with a specialization in structural engineering. He is currently teaching with the title of Assistant Professor at Al-Nahrain University – College of Engineering, Civil Engineering Department. He teaches many subjects for undergraduate and postgraduate students and has an important and large group of research that can be accessed through the available electronic search engines. Assist Prof. Dr. Risan has a member of the Iraqi Engineers Syndicate and a faculty member at Al-Nahrain University and other educational institutes.
References
- Arora, P., Singh, P. K., Bhasin, V., Vaze, K. K., Ghosh, A. K., Pukazhendhi, D. M., Gandhi, P., & Raghava, G. (2011). Predictions for fatigue crack growth life of cracked pipes and pipe welds using RMS SIF approach and experimental validation. International Journal of Pressure Vessels and Piping, 88(10), 384–17. https://doi.org/10.1016/j.ijpvp.2011.07.003
- CALLISTER, W. D., Jr. (2000). Materials science and engineering: An introduction.
- Cerit, M. (2019). Corrosion pit-induced stress concentration in spherical pressure vessel. Thin-Walled Structures, 136, 106–112. https://doi.org/10.1016/j.tws.2018.12.014
- Faur, N., Galatanu, S. V., & Hluscu, M. (2014). Study of multiple holes influence on theoretical stress concentration coefficient in case of cylindrical vessels. Key Engineering Materials, 601, 129–132. https://doi.org/10.4028/www.scientific.net/KEM.601.129
- GRIFFITH, A. A. (1920). The phenomenon of rupture and flow in solids. Philosophical Transactions of the Royal Society A: Mathematical, Physical and Engineering Sciences, 221, 163–198. https://doi.org/10.1098/rsta.1921.0006
- Inglis, C. E. (1913). Stresses in a plate due to the presence of cracks and sharp corners. Transactions of the Royal Institution of Naval Architects Part B: International Journal of Small Craft Technology, 55, 219–241. https://imechanica.org/files/1913%20Inglis%20Stress%20in%20a%20plate%20due%20to%20the%20presence%20of%20cracks%20and%20sharp%20corners_0.pdf
- Irwin, G. R. (1948). Fracture dynamics, fracturing of metals. American Society of Metals, 296
- Kashkoli, M. D., Tahan, K. N., & Nejad, M. Z. (2019). Creep damage and life assessment of thick cylindrical pressure vessels with variable thickness made of 304L austenitic stainless steel. Steel and Composite Structures, 32(6), 701–715. https://doi.org/10.12989/scs.2019.32.6.701
- Kirsch, C. (1898). Die theorie der elastizitat und die bedurfnisse der festigkeitslehre. Zeitschrift Des Vereines Deutscher Ingenieure, 42, 797–807. https://www.researchgate.net/profile/Manfred-Staat/post/Generalization-of-Kirschs-problem-to-finite-plate/attachment/5e11a949cfe4a777d4011a72/AS%3A843743624171521%401578175564579/download/Kirsch.pdf.
- Kostinakis, K., & Morfidis, K. (2017). The impact of successive earthquakes on the seismic damage of multistorey 3D R/C buildings. Earthquakes and Structures, 12(1), 1–12. https://doi.org/10.12989/eas.2017.12.1.001
- Lee, S., Cho, J., Lee, C., & Cho, S. (2021). Experimental and numerical investigations of near-field underwater explosions. Structural Engineering and Mechanics, 77(3), 395–406. https://doi.org/10.12989/sem.2021.77.3.395
- Li, F., & Chen, X. (2020). POD analysis for modeling wind pressures and wind effects of a cylindrical shell roof. Wind and Structures, 30(6), 559–573. https://doi.org/10.12989/was.2020.30.6.559
- Li, F. M., Hu, C., & Huang, W. H. (2003). Elastic wave scattering and dynamic stress concentrations in cylindrical shells with a circular cutout. Journal of Sound and Vibration, 259(5), 1209–1223. https://doi.org/10.1006/jsvi.2002.5222
- Luo, L., Xiang, Y., & Wang, Q. (2012). Stress concentration factor expression for tension strip with eccentric elliptical hole. Applied Mathematics and Mechanics, 33(1), 117–128. https://doi.org/10.1007/s10483-012-1537-7
- Magnucki, K., Szyc, W., & Lewiński, J. (2002). Minimization of stress concentration factor in cylindrical pressure vessels with ellipsoidal heads. International Journal of Pressure Vessels and Piping, 79(12), 841–846. https://doi.org/10.1016/S0308-0161(02)00101-1
- Makulsawatudom, P., Mackenzie, D., & Hamilton, R. (2004). Stress concentration at crossholes in thick cylindrical vessels. The Journal of Strain Analysis for Engineering Design, 39(5), 471–481. https://doi.org/10.1243/0309324041896506
- Rebora, A. U., & Vernassa, G. (2020). Transverse circular holes in cylindrical tubes loaded in traction and in flexion: A new analytical approximation of the stress concentration factor. Materials, 13(6), 1331. https://doi.org/10.3390/ma13061331
- Srinivasan, G., & Lehnhoff, T. F. (2001). Bolt head fillet stress concentration factors in cylindrical pressure vessels. Journal of Pressure Vessel Technology, 123(3), 381–386. https://doi.org/10.1115/1.1379530
- Tao, M., Zhao, R., Du, K., Cao, W., & Li, Z. (2020). Dynamic stress concentration and failure characteristics around elliptical cavity subjected to impact loading. International Journal of Solids and Structures, 191, 401–417. https://doi.org/10.1016/j.ijsolstr.2020.01.009
- Tide, D. (2003). The great Boston molasses flood of 1919. Stephen Puleo. Beacon Press.
- Troyani, N., Jaimes, N., Sterlacci, G., & Gomes, C. J. (2005). Stress concentration effects in short cylindrical vessels with holes subjected to tension: A complete account. Journal of Pressure Vessel Technology, 127(2), 184–189. https://doi.org/10.1115/1.1904051
- Withey, P. A. (1997). Fatigue failure of the de Havilland comet I. Engineering Failure Analysis, 4(2), 147–154. https://doi.org/10.1016/S1350-6307(97)00005-8
- Zhang, W. (2016a). Evaluation of susceptibility to hydrogen embrittlement—A rising step load testing method. Materials Sciences and Applications, 7(8), 389. https://doi.org/10.4236/msa.2016.78035
- Zhang, W. (2016b). Technical problem identification for the failures of the liberty ships. Challenges, 7(2), 20. https://doi.org/10.3390/challe7020020
- Zirka, A. I., & Chernopiskii, D. I. (2003). Stress concentration in an axially compressed cylindrical shell of medium thickness with an elliptic opening. International Applied Mechanics, 39(11), 1335–1338. https://doi.org/10.1023/B:INAM.0000015605.53515.4a