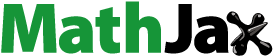
Abstract
The present study aims to improve the tensile and hardness properties of carbon fabric–reinforced epoxy composite (CFEC) by using hexagonal boron nitride (h-BN) and molybdenum disulfide (MoS2) fillers. Magnetic stirring and ultrasonication were used to disperse fillers in epoxy resin. CFEC, h-BN filled CFEC and MoS2 filled CFEC fabricated as per hand layup and vacuum bag technique. The tensile strength of CFEC was remarkably improved up to 6 wt.% h-BN and MoS2 addition separately in the resin matrix. The maximum tensile strength of 486 MPa and Young’s modulus of 44 GPa were noticed in 6 wt.% h-BN incorporated CFEC displaying 59% and 47% improvements, respectively. The maximum toughness is shown by 6 wt.% MoS2 incorporated CFEC indicating 102% improvement. The uniformly dispersed high-performance filler in the epoxy effectively transferred stress, improving tensile properties. However, filler agglomerates, stress-raising spots, and poor filler–matrix interaction beyond 6 wt.% filler reduced the tensile property improvement. The introduction of h-BN and MoS2 in the composite increased hardness, with 6 wt.% h-BN added CFEC recording the highest hardness denoting 23% greater than the neat CFEC.
1. Introduction
The applications of carbon fiber–reinforced epoxy composite (CFEC) for load-bearing structural materials increase predominantly in aviation, defense, railway, and automotive segments because of their decent strength-to-weight ratio, stiffness, thermomechanical, and tailorable properties (Buragohain, Citation2017; Chawla, Citation2012; Glover, Citation2004; Newcomb, Citation2017; Song, Citation2016). CFEC is also used in boat structural components such as a deck and hull, where weight reduction and corrosion resistance are important (Han et al., Citation2020). High speed, better payload capacity, and easy maneuverability can be achieved by using CFEC as construction material in a naval ship (Gargano et al., Citation2017; Tawfik et al., Citation2017). Composites used in these applications are exposed to a variety of loading conditions in their service life. The tensile strength and toughness are extremely important apart from the thermomechanical properties of composites for better performance of structural components. It is known that carbon fiber–neat epoxy composite has moderate tensile (Baptista et al., Citation2016) and toughness (crack resisting ability) properties due to brittle epoxy matrix (Frigione & Lettieri, Citation2020). The hardness of the composite affects its machinability; machining becomes difficult with harder composites. Composites of too high hardness adversely affect the machined surface quality and tool life (Altin Karataş & Gökkaya, Citation2018; Rao et al., Citation2019; Sheikh-Ahmad & Davim, Citation2012). On the contrary, a material with high hardness shows better scratch and abrasion resistance. In general, composites that are harder than the abrasive material exhibit a lower wear rate (better wear resistance). Hence, a composite with excellent tensile properties and moderate hardness is required. The tensile property of epoxy-based composite can be upgraded by adding a solid filler into a matrix while fabricating the composite (Hiremath et al., Citation2021; Sudarshan, Citation2021). The epoxy matrix reinforced with optimum filler concentration improves the matrix and fiber–matrix interface properties (Jiang, Citation2022; Nayak et al., Citation2021; Praveenkumara et al., Citation2021; Rao et al., Citation2021), hence increasing the load-carrying capacity.
Research studies have reported the application of solid-lubricant filler-loaded composite for high-performance (Gantayat et al., Citation2015; Kavimani et al., Citation2021; R. Kumar et al., Citation2018; Ribeiro et al., Citation2020). The solid-lubricant filler has a lamellar structure that shears easily, improves wear resistance of tribological contact surfaces, reduces friction coefficient and lowers thermal degradation of composite (Ben Difallah et al., Citation2012; Donnet & Erdemir, Citation2006; Scharf & Prasad, Citation2013). Commonly used solid-lubricant fillers are graphite, molybdenum disulfide (MoS2), and graphite-like hexagonal boron nitride (h-BN). A group of researchers determined the tensile property of solid-lubricant dispersed polymer composite and studied the filler effect on the obtained property. Kumar et al. (R. Kumar et al., Citation2018) examined the influence of micro graphite flakes (GF) on tensile properties of carbon fabric-phenolic composite (CFPC). The authors reported a tensile modulus of 40 wt.% GF added CFPC improved by 78% whereas, tensile strength of 20 wt.% graphite added CFPC improved by 5%. The authors also mentioned an increased trend of tensile modulus as the GF concentration in phenolic matrix varied from 10 to 40 wt.%. Kavimani et al. (Kavimani et al., Citation2021) reported eight times improvement in tensile strength when glass fiber/epoxy composite was added with hybrid 1 wt.% graphene oxide (GO) and 2 wt.% h-BN fillers, respectively. The interlocking of fillers improved interfacial adhesion and tensile property. However, the tensile strength is reduced for 1 wt.% GO and 3 wt.% h-BN hybrid combination due to the agglomeration of h-BN. It was also confirmed that the inclusion of hybrid GO and h-BN filler makes the composite rigid and brittle. Yu et al. (Yu et al., Citation2017) reported a three times reduction in tensile strength due to 44 vol% micro size h-BN platelet addition to epoxy because of h-BN aggregation. Liu et al. (Liu et al., Citation2016) found 13 and 22% growth in the tensile strength and modulus when 3 wt.% ball milled h-BN dispersed in polyether-ether-ketone (PEEK). The extent of tensile property improvement depends on the size, shape, quantity, and interfacial adhesion of filler with polymer, apart from the chemical compositions (Agunsoye et al., Citation2019; Frigione & Lettieri, Citation2020; Ramdani, Citation2019; Wypych, Citation2016). Sudheer et al. (Sudheer et al., Citation2013) reported a decrease in tensile strength, modulus, and percentage elongation by 17, 4, and 30% due to 10 wt.% MoS2 incorporation in epoxy resin. The stress concentration and microvoid developments in the composite are responsible for the decline in tensile properties. Arshad et al. (Arshad et al., Citation2020) noticed an improvement in the tensile modulus by 7.4 times and tensile strength by 2 times due to 0.9 wt.% MoS2 nanosheets addition to polystyrene matrix. The experimental investigation of BN-CFEC and MoS2-CFEC by Rao et al. (Rao et al., Citation2021) demonstrated that h-BN and MoS2 fillers of less than 8 wt.% are more beneficial for improving flexural and interlaminar shear strength of composites. Ulus et al. (Ulus et al., Citation2015) incorporated 0.5 wt.% multiwalled carbon nanotubes (CNTs) and 0.3 wt.% boron nitride nanoparticles (BNNPs) in the CFEC individually and hybrid combination. As per their study, the tensile strength was increased by 12%, 17%, and 20% whereas, the elastic modulus increased by 6%, 9%, and 10% because of BNNPs, CNTs, and hybrid BNNPs-CNTs addition to the epoxy. The solid-lubricant filler inclusion with epoxy inhibits epoxy chain mobility because of spatial confinement. Hence, the properties (tensile modulus and strength) which are associated with the polymer chain movement vary with filler addition (Rasul et al., Citation2021). Kumar et al. (K. Kumar et al., Citation2021) found that adding 4 wt.% zirconium dioxide (ZrO2) to epoxy increased its tensile strength, modulus and toughness up to 34, 12, and 140%, respectively. The excellent bonding between ZrO2 and epoxy allows for better stress distribution, which is responsible for augmentation in the property. The decreased tensile property of 8 wt.% ZrO2-reinforced epoxy composite was caused by agglomeration, which deteriorated interfacial bonding between ZrO2 and epoxy. According to Bello et al. (Bello et al., Citation2021), 10 wt.% eggshell nanoparticles (ESPs) loaded with recycled low-density polyethylene improved tensile strength by 68% owing to the effective load distribution from polyethylene to ESP. The more filler quantity not only reduces composite properties but also increases the viscosity of the filler-loaded epoxy, which affects the smooth flow and uniform spread of filler-loaded epoxy (K. Kumar et al., Citation2021; Sudheer et al., Citation2013). Consequently, there are more microvoids. Moreover, at high filler concentrations, uniform filler distribution becomes difficult resulting in a weak interface (G. Zhang et al., Citation2011). The material’s abrasive wear loss is inversely related to the product of stress and strain at failure (UTS×strain)−1 according to Ratner-Lancaster model (Lancaster, Citation1968; Srinath & Gnanamoorthy, Citation2006).
The present research aims at an in-depth understanding of tensile strength, modulus, and toughness of the carbon fabric/h-BN/epoxy (hybrid), carbon fabric/MoS2/epoxy (hybrid), and carbon fabric/epoxy (neat) composites. Thereby, the effect of h-BN and MoS2 fillers on the aforementioned properties was investigated. Also, the tensile test specimen fracture morphology was analyzed based on scanning electron microscope (SEM) images. To the best of author’s knowledge, research articles documenting the tensile, toughness, and hardness properties considering the above-mentioned combination of fiber and filler with an epoxy matrix are limited. There are limited studies that deal with fillers range from 2 to 8 wt.% at intervals of 2 wt.%. Hence, fillers from 2 to 8 wt.% were chosen in this work.
2. Experimental
2.1. Materials properties
Carbon fabric of twill weave patterns comprising 0 and 90 ° oriented fibers procured from M/S Arrow tech. tex., Mumbai. The carbon fiber bundle consists of 3000 filaments. The yarn has a fineness of 200 tex and a linear density of 5 per 10 mm. The thickness, density, elasticity modulus, and tensile strength of carbon fabric are 0.25 mm, 1.76 g.cm−3, 205 GPa, and 5089 MPa, respectively. Diglycidyl ether of bisphenol-A epoxy and amine hardener were supplied by M/S Atul Ltd., India. Epoxy has a density of 1.15 g.cm−3 and viscosity of 11,000 mPa.s (at 25 °C). The h-BN and MoS2 are procured from M/S Sigma-Aldrich Ltd., India. Young’s modulus of h-BN and MoS2 are 0.93 and 0.36 TPa, respectively (Mukhopadhyay et al., Citation2017). The density of h-BN and MoS2 are 2.1 and 5.1 g.cm−3 respectively. The h-BN has good thermal conductivity, dimension stability, dielectric property, fire-retardant ability, and anti-oxidation lubricant (Duan et al., Citation2014; Ma et al., Citation2019). MoS2 is a transition metal di-chalcogenide that exhibits noteworthy electronic, mechanical, barrier, and thermal properties (Arshad et al., Citation2020; Jing et al., Citation2020).
2.2. Composite preparation
The h-BN and MoS2 fillers of 2, 4, 6, and 8 wt.% dispersed separately in the weighted quantity of epoxy matrix. The filler was added to epoxy and placed over a magnetic stirrer (rotational speed 500 rpm, temperature 60 °C, time 20 minutes) for uniform mixing. Later, the epoxy–filler solution was ultrasonicated for 30 minutes in a probe sonicator (frequency 20 kHz, amplitude 50%) where the h-BN/MoS2 structure scission due to the cavitation effect generated by acoustic-wave energy. After stirring and sonication, the filler-epoxy solution was blended with the triethylene-tetraamine cure agent taken 12 wt.% of epoxy, and hand stirred for a few moments. Bottom peel-ply and carbon fabric are placed on mild steel mold, which is applied with a release agent. The fabric is chosen in such a way that warp and weft fibers are balanced to obtain identical in-plane properties. The resulting filler-loaded resin was manually applied with a brush over laid carbon fabric. Each laminate is composed of 10 stacked layers that are covered with peel-ply and porous breather fabric. The entire arrangement was wrapped in a vacuum bag film and connected to a vacuum pump. The vacuum pressure (350 mm of Hg) was applied to force out excess resin and compact the laminate. The composite laminate was then post-cured for 2 hours at 50 °C accompanied by room-temperature curing for one full day. Thus, produced laminates had a thickness ≈ 4 mm. The carbon fabric-neat epoxy was prepared without using filler. The complete details of composite ingredients and the designation of various composites are disclosed in Table . Figure depicts a schematic of the composite material preparation method. Figure shows the images of fabrication process for h-BN loaded CFEC. The same procedure is used to prepare MoS2 loaded CFEC.
Table 1. Material combination and physical properties of the neat and hybrid composites
2.3. Density and void measurement
The density and void fraction of composites are estimated as per the ASTM D2374 (ASTM International, Citation2016). The theoretical density and void volume fraction (%) were calculated using Equationequations (1)(1)
(1) and (Equation2
(2)
(2) ) respectively. The experimental density is evaluated by dividing composite weight by volume.
Where carbon fiber weight fraction (WCF) = 0.5; carbon fiber density = 1.8 g.cm−3; epoxy weight fraction (WEP) = 0.5-filler weight fraction; epoxy density = 1.15 g.cm−3; filler weight fraction (WF) = 0.02 to 0.08; h-BN density = 2.1 g.cm−3; MoS2 density = 5.06 g.cm−3.
2.4. FESEM morphology characterization
The morphology of fillers was studied through a field emission scanning electron microscope (FESEM) (MIRA-3 LMH, TESCAN). The fillers were gold-sputtered using a sputter coater (HHV, BT300). The energy dispersive spectroscope (EDS) (EDAX corporation, Inc.) is used to analyze elements or chemical composition of BN-CFEC and MoS2-CFEC.
2.5. Tensile test details
Young’s modulus, tensile strength, and strain of different composites can be determined from the tensile test. These are required for designing composites. The composites’ tensile behavior was studied as per ASTM D3039 (ASTM International, Citation2017) in a computerized universal testing machine (Zwick/Roell Z100), and displacement was measured with an extensometer of resolution 0.12 µm. The cross-head speed of 2 mm.min−1 was used for the test. The morphology of the tensile fractured specimens was inspected by using the SEM (ZEISS-EVO 18 and JSM-6380, JEOL), fracture surface was gold sputter-coated using coater (JFC-1600, JEOL, and Quorum SC-7620) to avoid sample charging during an inspection.
2.6. Hardness test details
Hardness denotes a material’s resistance to the indenter penetration under the action of a specific force that causes stretching, shear, and compression (Babenko et al., Citation2012). Knowledge about the hardness of a composite provides an approximate idea of its performance in wear and scratch tests. Harder composite is more resistant to scratches and wear. The bulk hardness of composites is measured using a Rockwell hardness tester on B-scale (Hitech-HIEPL). The hardened steel ball indenter (diameter 1.59 mm (1/16′′)) was used for the Rockwell hardness examination, which ensures even distribution of the load. A minor load of 10-kg force is applied to the specimen through the indenter, which eliminates the effect of backlash and surface asperities on the obtained hardness. Later, a major load of 100-kg force is applied. After 15 seconds, the major load is removed and the hardness number is noted down from the dial indicator. The depth of penetration of the ball indenter decides the hardness. The average hardness value reported was based on five indents performed at different locations on each sample. The indenter impression and the surrounding damage surface of each composite were inspected through an optical microscope (Olympus BX53M).
3. Results and discussion
3.1. FESEM characterization of fillers
The FESEM image of h-BN particles shown in Figure ) depicts disk-like morphology having a narrow thickness below 20 nm. The Gaussian fitting of h-BN particle size distribution histogram given in Figure ) is drawn using Image-J 1.52b software. The size distribution histogram shows that the majority of h-BN particles have diameters ranging from 60 to 120 nm. The FESEM image of MoS2 shown in Figure ) reveals a thin sheet-like morphology having a narrow thickness below 10 nm. The MoS2 is irregular in shape and its Gaussian peak fitting size distribution histogram shown in Figure ) reveals size variation from 10 to 1800 nm. The maximum number of MoS2 particles ranges in size from 200 to 400 nm, with an average size of 314 nm.
3.2. Composites density and void fraction
Table shows the density and void percentage of the neat-CFEC and filler dispersed CFECs. It is found that the theoretical and experimental densities of filler-dispersed CFEC are higher compared to neat composite. The density and void percentage of composite raised as the filler content increased. The composite density attained the maximum at a higher filler concentration of 8 wt.%. The density of MoS2-CFEC is slightly greater than that of BN-CFEC because MoS2 is denser than h-BN. The void in the composite affects tensile, compressive, shear, flexural, and fatigue strength (Mehdikhani et al., Citation2019; A. Zhang et al., Citation2016). The void in filler-dispersed CFEC was found to be affected by filler size distributions and filler concentration. Minimum void content of 0.83% was found in 2BN-CFEC. The addition of nitride filler, like h-BN, to a polymer matrix improves reinforcement-matrix adhesion and minimizes voids in the composite (A. Khan et al., Citation2022). The largest void content of 2.58% was found in 8MoS2-CFEC. The smooth flow of the polymer is impaired as the filler quantity increases, resulting in poor carbon fiber wetting by viscous epoxy filler, and hence the voids are increased.
3.3. Tensile properties
The tensile stress-strain behavior of neat and hybrid composites is presented in Figure . Table shows the average tensile test result with error variation based on five samples tested in each composition. The percentage change in UTS, Young’s modulus, and toughness of each composite group in comparison to baseline neat composite are presented in Table . All the hybrid composites showed better tensile strength and modulus than neat composite because of the superior mechanical properties of the h-BN and MoS2 fillers. Tensile strength increased up to 6 wt.% filler and decreased after 6 wt.% filler loading in both types of composites. The better stress transfer in h-BN-loaded CFEC is expected due to hydrogen bonding between the nitrogen atoms of h-BN and the hydroxyl group of epoxy (Tsuji et al., Citation2019). Furthermore, improved interactivity of high-performance h-BN filler with epoxy effectively distributes the load from the epoxy to carbon fiber via filler media. Filler loadings of 2 and 4 wt.% may not be adequate to modify the entire epoxy matrix and interface properties, and therefore not able to witness tensile strength improvement as that of 6 wt.%. The 6BN-CFEC and 6MoS2-CFEC exhibited the maximum ultimate tensile strength (UTS) of 487 MPa and 456 MPa, respectively, which were 59% and 49% higher than the neat-CFEC. The 6BN-CFEC exhibited higher tensile strength as well as higher Young’s modulus. The reason for an increase in tensile strength is also due to a well-distributed filler, which promotes fair fiber–filler–matrix interfacial adhesion and sustains maximum load (Chaudhry & Mittal, Citation2014). Tensile strength was reduced in 8BN-CFEC and 8MoS2-CFEC. In the literature, decreased UTS was reported at higher loading of exfoliated MoS2 added to polystyrene matrix (M. B. Khan et al., Citation2017) and h-BN added to sisal fiber/epoxy composite (Agrawal et al., Citation2020). This decrement in the improvement of tensile property at higher filler loading is due to agglomeration, which is likely caused by the matrix saturation, i.e., the inability of epoxy to bind all filler particles. As a result, during tensile loading, empty micro spaces/voids and cavities are generated. This inference is supported by the literature (Agunsoye et al., Citation2019). Moreover, the increased number of close particles resulted in stress concentration, which constitutes weak points and leads to composite failure at low load (Bello et al., Citation2018; K. Kumar et al., Citation2021). The inadequacy of resin bonding to the filler within the agglomerates causes their de-cohesion under the applied load (Baptista et al., Citation2016). Agglomerated filler hinders the interchain interactions (crosslinking) during polymer cure, reducing tensile strength (Frigione & Lettieri, Citation2020). Also, agglomerated filler behaves as a filler with a limited surface area, reducing the effective filler-matrix bonding (Azimpour-Shishevan et al., Citation2020). Yet, the UTS and Young’s modulus of 8 wt.% filler added CFEC was still higher than the neat-CFEC due to the strength and stiffness contribution of fillers to the composite.
Table 2. Tensile strength, modulus, strain, and toughness of the neat and hybrid composites
With the addition of filler to CFEC, the change in tensile stress is significantly greater than the change in strain. Therefore, Young’s modulus increases as stress increases up to 6% filler addition to CFEC. The improved Young’s modulus of filler dispersed CFEC compared to neat-CFEC attributed to constrained polymer chain mobility, better stress transfer at the interface, and increased stiffness of composite with filler inclusion in the matrix (Fu et al., Citation2008; Joy et al., Citation2020). At all filler concentrations, Young’s modulus of BN-CFEC is higher than MoS2-CFEC. This difference in the improvement level of modulus is likely due to the difference in modulus (stiffness) between h-BN and MoS2 fillers. The maximum Young’s modulus of 43.76 GPa was witnessed for 6BN-CFEC, indicating a 47% improvement. In MoS2-CFEC, the highest Young’s modulus of 38.15 GPa was noticed for 4MoS2-CFEC. A decrease in Young’s modulus detected as the filler concentration exceeds 6 wt.% indicates poor interface adhesion of filler with matrix and large agglomerates of filler particles (Wypych, Citation2016). Layer-wise filler slip under the action of tensile force also reduces composite Young’s modulus.
The tensile stress-strain response demonstrates that 6 wt.% filler-loaded CFEC fails at a slightly larger strain. The increased strain (elongation at break) in both filler added composites illustrates that filler domains may cause cavitation and polymer chains to yield during mechanical deformation (Joy et al., Citation2020). The tensile failure strain of 8BN-CFEC and 8MoS2-CFEC although reduced but not significantly. This behavior is consistent with the literature (Shivamurthy et al., Citation2013), which reports that a higher concentration of graphite filler addition to glass fabric/epoxy composite results in lower tensile strain. The lower composite failure strain indicates poor filler–epoxy interface adhesion, increased stress concentration, and discontinuities in the epoxy (Joy et al., Citation2020; Sudheer et al., Citation2013).
The obtained trend of Young’s modulus, strength, and strain agree with the observed behavior of GO nanoflakes-filled carbon fabric/epoxy (Adak et al., Citation2018), nano h-BN-filled epoxy (Joy et al., Citation2020), and exfoliated h-BN added PEEK (Liu et al., Citation2016). Furthermore, the maximum tensile property attained at filler wt.% differed from that described in the literature, possibly due to differences in filler size, filler morphology, and processing conditions adopted.
The toughness signifies the amount of energy necessary for the complete fracture of a tensile specimen due to imposed stress (Chatterjee et al., Citation2011). It is estimated by integrating the absolute area under the stress–strain curve up to the fracture point in “Origin pro®” software. The key factors that contribute to the toughness of polymer nanocomposite include crack deflection, matrix deformation, fiber/filler debonding, fiber/filler pull-outs, and fiber/filler fracture (Adak et al., Citation2018; G. Zhang et al., Citation2011). 6MoS2-CFEC exhibited the maximum toughness of 2943 kJ.m−3 indicating 102% higher compared to the neat-CFEC. The combined increase of failure stress and strain in 6MoS2-CFEC causes improvement in the toughness (energy absorption capability). The 6BN-CFEC also improved the toughness by 93%. It is observed that the 6BN-CFEC is stiffer but less tough compared to the 6MoS2-CFEC. The uniformly distributed high modulus fillers in epoxy efficiently transfer load and improve toughness. Previous research studies demonstrated that the h-BN nanofiller increases the toughness of polyethylene (Chaurasia et al., Citation2019) and epoxy (song et al., Citation2019). In another study, the authors claimed that adding MoS2 to polystyrene is capable of improving toughness (Arshad et al., Citation2020). As the filler concentration surpasses 6 wt.%, clusters of filler aggregate formation likely reduce the toughness of composite (Chaurasia et al., Citation2019).
The reciprocal of the product of average UTS and strain was evaluated. It was found that the (UTS×strain)−1 decreased up to 6 wt.% filler dispersed CFEC and then increased at 8 wt.% filler content. The larger (UTS×strain)−1 factor is caused by a sudden decrease in strain, thus the composite exhibits brittle behavior. The graph presented in Figure shows the average variation of UTS, strain, modulus, and toughness among BN-CFEC and MoS2-CFEC at different filler concentrations. The change in crosslink density due to an immobilized network of epoxy around filler is the possible reason for the mobility change of epoxy molecules, which alters the stiffness (modulus), Tg, and toughness of composite (Sue et al., Citation2000; Wetzel et al., Citation2006).
Figure 5. UTS, strain, modulus, toughness trends in BN-CFEC and MoS2-CFEC at different filler concentrations.

Figure ) shows the photographs of tensile test specimens indicating the failure zone. All composite samples failed within the gage region. Neat-CFEC experiences edge delamination and angled failure apart from fiber breakage indicating brittle failure. The 2BN-CFEC, 8BN-CFEC, 4MoS2-CFEC, and 8MoS2-CFEC specimens undergo lateral cross-section failure. The 4BN-CFEC experienced lateral gage failure and fiber breakage. An angled failure mode was evident in 6BN-CFEC. 2MoS2-CFEC exhibited fiber splitting and fiber pull-out. The 6MoS2-CFEC specimen display damage at two different locations apart from damage near the grip.
3.4. SEM image analysis of tensile specimens
The fracture types of composite in the tensile test were studied through SEM characterization. The fracture micrograph of neat-CFEC is presented in Figure ). The transverse carbon fiber breakage is more severe than longitudinal fibers (Figure )) because the fibers oriented 90 ° to the loading direction break earlier than the loading direction fibers. In addition, the strength of fibers follows a statistical distribution as few fibers will endure slightly low-stress levels and break early compared to the others (Mallick, Citation2007). Figure ) displays evidence of resin cusp formation visible as raised platelets on the fiber edges resulting from the brittle failure. In the literature, the presence of resin cusp in carbon/epoxy laminates has also been mentioned (Souza et al., Citation2019). When the applied tensile load exceeds the interface bond strength, the carbon fibers debond from the surrounding epoxy via a crack running at the fiber–matrix interface, as seen in Figure ; Mallick, Citation2007). This denotes the incapability of the initiated matrix crack to propagate across the carbon fibers because of the weak interface in neat CFEC (Agarwal et al., Citation2015). Therefore, neat-CFEC has low toughness. Figure ) shows deep holes developed because of fiber pull-out. A circled region in Figure ) indicates the surface of bare carbon fibers, which reveals an adhesive failure with fiber pull-outs. A similar observation was reported in the literature (Jain et al., Citation2021).
The SEM micrograph of 4BN-CFEC taken at the fracture location is presented in Figure ). Serration and matrix fracture (Figure )) and splintered carbon fibers (Figure )) are visible at a few locations on the fracture surface. The void formed (Figure )) due to carbon fiber pull-out. The river-like pattern reveals that 4BN-CFEC inhibits crack propagation and requires more deformation energy to fracture. Michalcová & Kadlec (Michalcová & Kadlec, Citation2016) made a similar observation for multiwalled CNTs added to CFEC. Figure ) depicts the zone of intimate contact between carbon fibers and h-BN filled epoxy, accompanied by intact carbon fibers. However, 4BN-CFEC also exhibits transverse carbon fiber debonding (Figure )).
The fracture micrograph of 6BN-CFEC is presented in Figure ). Figure ) reveals the matrix microcrack and fiber imprint (step-like feature) on the matrix. Figure ) shows projected carbon fibers. Figure ) shows matrix flaking and carbon fiber–hBN filler–epoxy intimate contact zone (good bonding). The cross-sectional morphology view of 6BN-CFEC from Figure ) reveals a carbon fiber/h-BN/epoxy interface with less fiber–matrix debonding. A similar finding was also reported for graphene nanoplatelets (GNPs) distributed CFEC (Jain et al., Citation2021). The path of debonded carbon fibers and microcrack propagation is confirmed in Figure ). The stiff h-BN distributed epoxy provides resistance against crack propagation by deviating the crack flow and absorbing fracture energy. In the literature, identical observations have been made for h-BN filled epoxy (Ulus et al., Citation2014). Carbon fibers circumferentially surrounded by h-BN dispersed epoxy and crack branching marks are seen in Figure ).
The fracture micrograph of 8BN-CFEC is shown in Figure ). Good carbon fiber/h-BN/epoxy bonding is seen in Figure ) at a few locations. Nevertheless, tiny pores/crazes (Figure )) are produced due to increased stress during tension/pull. The h-BN filler protruded and was present at the fracture surface as in Figure ); this was perhaps caused by the addition of more h-BN concentration to epoxy, which reduced its ability to interact with epoxy. Material dislodges due to crack propagation and carbon fiber pull-out are found in the fracture micrograph of 8BN-CFEC (Figure )). The transverse carbon fiber debonding is evident in Figure ). Hackle formation (Figure )) occurs when the microcrack growth in a composite is prevented.
The SEM image of a tensile tested 4MoS2-CFEC is shown in Figure ). The carbon fiber–dominated failure (Figure )) was witnessed instead of matrix failure in 4MoS2-CFEC due to MoS2 strengthened epoxy. Shallow cusp, matrix feature presents on the carbon fiber edges (Figure )). Cusp developed in the composite due to localized stress (Suresha et al., Citation2007). Epoxy resin shards cling to carbon fiber (Figure )) indicating good fiber–matrix adhesion. Figure ) shows a cross-section view of the fracture region and demonstrates MoS2 strengthened epoxy impeding crack growth. The tensile test fracture morphology of 6MoS2-CFEC is shown in Figure ). Splintered carbon fibers (Figure )) and fibers debonding (Figure )) are witnessed in 6MoS2-CFEC. In 4MoS2-CFEC (Figure )) and 6MoS2-CFEC (Figure )), fillers are present as a coating on the carbon fiber surface. Therefore, a greater pull-out force is necessary to dislodge fiber from the matrix, thus exhibiting larger resistance to tensile loading. Nayak et al. made a similar observation for coconut shell powder modified glass/epoxy composite (Nayak et al., Citation2022). Carbon fibers circumferentially wetted by MoS2 filled epoxy seen in Figure ) illustrate intimate contact of the fiber–filler–matrix. Therefore, 6MoS2-CFEC has better tensile strength and toughness. A similar inference was also reported for GNPs added CFEC (Jain et al., Citation2021).
The tensile test fracture surface of 8MoS2-CFEC is shown in Figure ). As presented in Figure ), intralayer carbon fiber splaying (separation) occurs under tensile load, and it may be due to an abrupt loading effect. Carbon fiber splintering (Figure )) and broom-like fiber bundle distortion (Figure )) are noticed in the fracture morphology of 8MoS2-CFEC. Interface debonding (Figure )) occurred might be due to weak carbon fiber–MoS2–epoxy interface bonding as a result of MoS2 agglomeration. The cross-section of fiber fracture and severe matrix rupture caused by overloading (8 wt.%) MoS2 filler (Figure )). MoS2-rich resin in between fibers is noticed in Figure ).
3.5. Microstructure of filler–epoxy samples
The uniformly dispersed filler in the matrix causes better filler–matrix interaction and can upgrade the resultant properties of the matrix by improving stress transfer from matrix to reinforcement (Nayak et al., Citation2021). From the prepared filler–epoxy composite sample without fiber, the microstructure of filler and its distribution in an epoxy matrix are investigated using SEM. Figures ) represent SEM micrographs of MoS2 and h-BN dispersed in epoxy resin at lower and higher filler concentrations. The region of well-impregnated MoS2 filler by the epoxy resin is witnessed in 2 wt.% MoS2-epoxy (Figure )), and it guarantees enhanced performance of the composite. MoS2 dispersed throughout the epoxy resin at 8 wt.% filler concentration (Figure )). The circled region with yellow color in Figure ) depicts a zone of evenly spaced MoS2 particles. However, MoS2 agglomeration is visible at some locations in the micrograph of 8 wt.% MoS2-epoxy, as indicated by the red color circled region (Figure )) on account of filler particle colonies (Zhou et al., Citation2014). The site of agglomerated MoS2 becomes a stress concentration point and results in poor filler–epoxy interaction, which lowers the composite tensile property (Azimpour-Shishevan et al., Citation2020). Also, microvoid formation is witnessed at higher MoS2 concentration as shown by the orange color circled region in Figure ). The SEM micrograph of 2 wt.% h-BN-filled epoxy in Figure ) reveals widely spaced h-BN particles at a few spots with some areas exclusively being epoxy resin. However, as the h-BN quantity increased to 8 wt.%, clusters/agglomeration of h-BN filler (Figure )) lessened the composite’s load-carrying capacity because the effective interaction surface area of h-BN with epoxy was reduced. A similar observation has been reported for BN nanosheet dispersed polymers (Rasul et al., Citation2021).
3.6. Energy dispersive spectroscope analysis
The scanned zones for EDS analysis, along with a graph representing intensity (counts per second) versus energy (keV) are shown in Figures ; BN-CFEC) and 14(b)(MoS2-CFEC). The identified elements in weight% are shown in Figure . In BN-CFEC, carbon (C) is the main element witnessed in addition to oxygen (O), boron (B), and nitrogen (N) elements. Boron and nitrogen elements confirm the presence of h-BN particles. The EDS graph of MoS2-CFEC confirms the existence of molybdenum (Mo) and sulfur (S) related to MoS2 particles apart from carbon (C) and oxygen (O).
3.7. Hardness
The Rockwell hardness number (RHN) variation with filler concentrations is displayed in Figure . The addition of stiff h-BN and MoS2 in CFEC increased the RHN of the composites at all the tested filler concentrations. The RHN gradually increases with h-BN and MoS2 content up to 6 wt.% then starts a decreasing trend for further filler addition in the composite. The increased hardness of filler added composite indicates that filler contributes its hardness as well as stiffness to the matrix. In addition, good bonding between filler-loaded matrix and fibers is responsible for improved composite hardness (Li et al., Citation2013). The greatest RHN of 88 (23%↑) was attained for 6BN-CFEC, indicating larger resistance exhibited by the composite to indenter penetration because of improved laminate interface bonding and remarkable stiffness as well as the hardness of h-BN. Thus, it produces a smaller depth of impression. This finding is supported by the literature (Liu et al., Citation2016), which reported a 34% increase in the ball indentation hardness of 5 wt.% h-BN added PEEK. RHN of 6MoS2-CFEC improved from 72 (neat-CFEC) to 87. MoS2 inclusion in polystyrene improved Brinell hardness by 20% as it exhibits resistance to deformation (M. B. Khan et al., Citation2017). In another study, it was reported that 10 wt.% MoS2 addition to neat-epoxy and glass/epoxy improved Rockwell hardness by 3.9 (RHN 80) and 19.5% (RHN 92), respectively (Sudheer et al., Citation2013). In the present work, the hardness of CFEC added with 8 wt.% filler slightly decreased due to micro-voids and the possibility of agglomeration in the composite. The reduced Rockwell hardness was reported at increased boron nitride nanotube concentration loaded to polyurethane (Li et al., Citation2013). Furthermore, the hardness is location-dependent due to the random distribution of fillers in CFEC, composite anisotropy, and inhomogeneity.
The optical microscope images of Rockwell hardness-tested composites surface are shown in Figure . No evidence of surface cracks visible from optical microscope images reveals composites have reasonably good bulk hardness. The image analysis confirms the difference in depth of indentation and damaged area (boundary) around the vicinity of indentation among various composites. The damaged area is computed through Image-J software, and the outcome is revealed in Figure . The minimum damaged area of 1.85 and 2.96 mm2 were noticed in 6MoS2-CFEC and 6BN-CFEC, respectively, due to a higher resistance exhibited by the aforesaid composites surface for indenter movement. The larger damaged areas of 3.95 and 3.74 mm2 were detected for 8MoS2-CFEC and neat-CFEC samples, respectively.
4. Conclusions
The tensile strength and Young’s modulus attained the maximum for 6BN-CFEC, exhibiting 59% and 47% enhancement, respectively. The increased stiffness, constrained epoxy chain mobility, and better degree of stress transfer due to filler inclusion in the CFEC increased Young’s modulus. The fracture morphology of 6BN-CFEC shows the intimate contact of carbon fiber/h-BN/epoxy in addition to carbon fiber imprint and debonded carbon fiber.
6MoS2-CFEC has the highest toughness. The zone of intact carbon fibers and crack growth obstruction due to MoS2 strengthened epoxy indicates improved energy absorption of 6MoS2-CFEC.
The tensile strength and toughness of 8 wt.% filler added CFEC not significantly improved due to agglomeration, which induces stress concentration and deteriorates the filler–matrix bonding. Therefore, the load-carrying capacity of this composite was limited.
When compared to the neat CFEC, the Rockwell hardness of 6BN-CFEC and 6MoS2-CFEC improved by 23% and 21%, respectively. The hardness improvement was due to a better fiber/matrix/filler interface and remarkable stiffness of the filler, which exhibits resistance to indenter penetration.
The research work could be extended in the future to study the effect of h-BN and MoS2 fillers on machinability, impact strength, residual stress, and tribological properties of CFEC. The tensile properties and toughness of CFEC blended with hybrid h-BN and MoS2 fillers can be explored in future works.
Acknowledgements
The authors are grateful to the Manipal Academy of Higher Education for providing the infrastructural facility to perform research.
Disclosure statement
No potential conflict of interest was reported by the author(s).
Additional information
Funding
Notes on contributors
Yermal Shriraj Rao
Mr Yermal Shriraj Rao is currently pursuing a Ph.D. in the Department of Mechanical and Industrial Engineering at the Manipal Institute of Technology, MAHE, India. He holds a B.E. in Mechanical Engineering and M.Tech. in Machine Design. His research area of interest includes the characterization of polymer nanocomposites and machining studies of composites.
Basavannadevaru Shivamurthy
Dr Basavannadevaru Shivamurthy, Associate Professor (Senior Scale) in the Department of Mechanical and Industrial Engineering at the Manipal Institute of Technology, MAHE, India, has received a Ph.D. from the National Institute of Technology Karnataka (NITK) Surathkal in the field of Materials Engineering. He has more than 10 years of industrial experience in the polymer processing and research. For the last 21 years, he has been involved in teaching and research. Also, he has been an active researcher in polymer nanocomposites, tribology, ballistic materials, and EMI shielding materials. He has published several research articles in national and international journals of repute.
Nanjangud Subbarao Mohan
Dr Nanjangud Subbarao Mohan, Professor in the Department of Mechanical and Industrial Engineering at Manipal Institute of Technology, MAHE Manipal, India, received his Ph.D. from the Manipal Institute of Technology in the field of machining studies on glass fiber composites. He has 35 years of teaching and research experience. His research interest includes composite machining, hygrothermal aging studies on natural fiber composite, and polymer nanocomposites. He has published several research articles in national and international journals of repute.
Nagaraja Shetty
Dr Nagaraja Shetty, Associate Professor in the Department of Mechanical and Industrial Engineering at Manipal Institute of Technology, MAHE Manipal, India, received his Ph.D. in the machining of carbon fiber–epoxy composite from the National Institute of Technology Karnataka (NITK) Surathkal. He has 8 years of experience in teaching and research. His areas of interest include the machining study of polymer composites, design, and optimization. He has published several research articles in national and international journals of repute.
Sanjay Mavinkere Rangappa
Dr Sanjay Mavinkere Rangappa is currently working as a Senior Research Scientist/Associate Professor and also Advisor within the office of the President for University Promotion and Development towards International goals at King Mongkut’s University of Technology North Bangkok (KMUTNB), Thailand. He received a Post Doctorate from KMUTNB. His current research areas include natural fiber composites, polymer composites, and advanced material technology.
Suchart Siengchin
Prof. Dr.-Ing. habil. Suchart Siengchin is the President of King Mongkut’s University of Technology North Bangkok (KMUTNB), Thailand. He received his Dipl.-Ing. in Mechanical Engineering from University of Applied Sciences Giessen/Friedberg, Hessen, Germany, M.Sc. in Polymer Technology from University of Applied Sciences Aalen, Baden-Wuerttemberg, Germany, M.Sc. in Material Science at the Erlangen-Nürnberg University, Bayern, Germany, Doctor of Philosophy in Engineering (Dr.-Ing.) from Institute for Composite Materials, University of Kaiserslautern, Rheinland-Pfalz, Germany and Postdoctoral Research from School of Materials Engineering, Purdue University, USA. In 2016 he completed the Habilitation (Dr.-Ing. habil.) in Mechanical Engineering from Chemnitz University of Technology, Saxony, Germany.
References
- Adak, N. C., Chhetri, S., Kim, N. H., Murmu, N. C., Samanta, P., & Kuila, T. (2018). Static and dynamic mechanical properties of graphene oxide-incorporated woven carbon fiber/epoxy composite. Journal of Materials Engineering and Performance, 27(3), 1138–24. https://doi.org/10.1007/s11665-018-3201-5
- Agarwal, B. D., Broutman, L. J., & Chandrashekhara, K. (2015). Analysis and performance of fiber composites (3rd ed.) (pp.1–562). In John Wiley & Sons, Inc. Wiley India Pvt. Ltd.
- Agrawal, A., Chandrakar, S., & Sharma, A. (2020). Mechanical and thermal behaviour of epoxy/hexagonal boron nitride/short sisal fiber hybrid composites. IOP Conference Series: Materials Science and Engineering, 840(1), 012011. https://doi.org/10.1088/1757-899X/840/1/012011
- Agunsoye, J. O., Bello, S. A., & Adetola, L. O. (2019). Experimental investigation and theoretical prediction of tensile properties of Delonix regia seed particle reinforced polymeric composites. Journal of King Saud University - Engineering Sciences, 31(1), 70–77. https://doi.org/10.1016/j.jksues.2017.01.005
- Altin Karataş, M., & Gökkaya, H. (2018). A review on machinability of carbon fiber reinforced polymer (CFRP) and glass fiber reinforced polymer (GFRP) composite materials. Defence Technology, 14(4), 318–326. https://doi.org/10.1016/j.dt.2018.02.001
- Arshad, M. U., Raza, H., Khan, M. B., & Hussain, A. (2020). Synthesis of 2D molybdenum disulfide (MoS2) for enhancement of mechanical and electrical properties of polystyrene (PS) polymer. Polymer Testing, 90, 106646. https://doi.org/10.1016/j.polymertesting.2020.106646
- ASTM International. (2016). ASTM D2734, Standard test methods for void content of reinforced plastics. https://doi.org/10.1520/D2734-16
- ASTM International. (2017). ASTM D3039, Standard test method for tensile properties of polymer matrix composite materials. https://doi.org/10.1520/D3039_D3039M-17
- Azimpour-Shishevan, F., Akbulut, H., & Mohtadi-Bonab, M. A. (2020). Synergetic effects of carbon nanotube and graphene addition on thermo-mechanical properties and vibrational behavior of twill carbon fiber reinforced polymer composites. Polymer Testing, 90, 106745. https://doi.org/10.1016/j.polymertesting.2020.106745
- Babenko, V. V., Chun, H. H., & Lee, I. (2012). Types of elastic surfaces and research of their mechanical characteristics. In V. V. Babenko, H. H. Chun, & I. Lee (Eds.), Boundary layer flow over elastic surfaces (pp. 181–293). Elsevier. https://doi.org/10.1016/B978-0-12-394806-9.00002-1
- Baptista, R., Mendão, A., Guedes, M., & Marat-Mendes, R. (2016). An experimental study on mechanical properties of epoxy-matrix composites containing graphite filler. Procedia Structural Integrity, 1, 74–81. https://doi.org/10.1016/j.prostr.2016.02.011
- Bello, S. A., Agunsoye, J. O., Adebisi, J. A., & Hassan, S. B. (2018). Optimisation of charge ratios for ball milling synthesis: Agglomeration and refinement of coconut shells. Engineering and Applied Science Research, 45(4), 262–272. https://ph01.tci-thaijo.org/index.php/easr/article/view/109771/116051
- Bello, S. A., Raji, N. K., Kolawole, M. Y., Adebayo, M. K., Adebisi, J. A., Okunola, K. A., & AbdulSalaam, M. O. (2021). Eggshell nanoparticle reinforced recycled low-density polyethylene: A new material for automobile application. Journal of King Saud University - Engineering Sciences. https://doi.org/10.1016/j.jksues.2021.04.008
- Ben Difallah, B., Kharrat, M., Dammak, M., & Monteil, G. (2012). Mechanical and tribological response of ABS polymer matrix filled with graphite powder. Materials & Design, 34, 782–787. https://doi.org/10.1016/j.matdes.2011.07.001
- Buragohain, M. K. (2017). Composite structures: Design, mechanics, analysis, manufacturing and testing. CRC Press. https://doi.org/10.1201/9781315268057
- Chatterjee, S., Nüesch, F. A., & Chu, B. T. T. (2011). Comparing carbon nanotubes and graphene nanoplatelets as reinforcements in polyamide 12 composites. Nanotechnology, 22(27), 275714. https://doi.org/10.1088/0957-4484/22/27/275714
- Chaudhry, A. U., & Mittal, V. (2014). Masterbatch approach to generate HDPE/CPE/graphene nanocomposites. In V. Mittal (Ed.), Synthesis techniques for polymer nanocomposites (pp. 31–50). Wiley-VCH Verlag GmbH & Co. KGaA. https://doi.org/10.1002/9783527670307.ch2
- Chaurasia, A., Verma, A., Parashar, A., & Mulik, R. S. (2019). Experimental and computational studies to analyze the effect of h-BN nanosheets on mechanical behavior of h-BN/polyethylene nanocomposites. The Journal of Physical Chemistry C, 123(32), 20059–20070. https://doi.org/10.1021/acs.jpcc.9b05965
- Chawla, K. (2012). Composite materials: Science and engineering (3rd ed.). In Springer Science & Business Media (pp. 1–510). Springer, New York.
- Donnet, C., & Erdemir, A.(2006). Friction mechanisms and fundamental aspects in solid lubricant coatings. In Y. Pauleau(Ed.), Materials surface processing by directed energy techniques (1st) (pp 573–593). Elsevier. https://doi.org/10.1016/B978-008044496-3/50018-6
- Duan, X., Wang, M., Jia, D., Jing, N., Wu, Z., Yang, Z., Tian, Z., Wang, S., He, P., Wang, Y., & Zhou, Y. (2014). Anisotropic mechanical properties and fracture mechanisms of textured h-BN composite ceramics. Materials Science and Engineering: A, 607, 38–43. https://doi.org/10.1016/j.msea.2014.03.132
- Frigione, M., & Lettieri, M. (2020). Recent advances and trends of nanofilled/nanostructured epoxies. Materials, 13(15), 3415. https://doi.org/10.3390/ma13153415
- Fu, J. F., Shi, L. Y., Yuan, S., Zhong, Q. D., Zhang, D. S., Chen, Y., & Wu, J. (2008). Morphology, toughness mechanism, and thermal properties of hyperbranched epoxy modified diglycidyl ether of bisphenol A (DGEBA) interpenetrating polymer networks. Polymers for Advanced Technologies, 19(11), 1597–1607. https://doi.org/10.1002/pat.1175
- Gantayat, S., Prusty, G., Rout, D. R., & Swain, S. K. (2015). Expanded graphite as a filler for epoxy matrix composites to improve their thermal, mechanical and electrical properties. New Carbon Materials, 30(5), 432–437. https://doi.org/10.1016/S1872-5805(15)60200-1
- Gargano, A., Pingkarawat, K., Blacklock, M., Pickerd, V., & Mouritz, A. P. (2017). Comparative assessment of the explosive blast performance of carbon and glass fibre-polymer composites used in naval ship structures. Composite Structures, 171, 306–316. https://doi.org/10.1016/j.compstruct.2017.03.041
- Glover, B. M. (2004). History of development of commercial aircraft and 7E7 dreamliner. Aviation Engineering, 592, 16–21.
- Han, Z., Choi, J., Hwang, I., Kim, J., & Oh, D. (2020). Basic design of high-speed riverine craft made of carbon fiber reinforced polymer. Journal of the Society of Naval Architects of Korea, 57(4), 241–253. https://doi.org/10.3744/SNAK.2020.57.4.241
- Hiremath, P., Kini, A., Shettar, M., Sharma, S., Jayashree, P. Benfratello, S. (2021). Investigation on tensile properties and analysis of wear property of glass fiber-epoxy-nanoclay ternary nanocomposite using response surface methodology. Cogent Engineering, 8(1), 1877869. https://doi.org/10.1080/23311916.2021.1877869
- Jain, V., Bisht, A., Jaiswal, S., Dasgupta, K., & Lahiri, D. (2021). Assessment of interfacial interaction in graphene nanoplatelets and carbon fiber-reinforced epoxy matrix multiscale composites and its effect on mechanical behavior. Journal of Materials Engineering and Performance, 30(12), 8913–8925. https://doi.org/10.1007/s11665-021-06115-2
- Jiang, Y. (2022). Using nano-powders to improve the interfacial bonding strength of carbon fiber/epoxy composites. Fibers and Polymers, 23(5), 1431–1439. https://doi.org/10.1007/s12221-022-4651-9
- Jing, Y., Wang, P., Yang, Q., He, Y., & Bai, Y. (2020). Molybdenum disulfide with poly(dopamine) and epoxy groups as an efficiently anticorrosive reinforcers in epoxy coating. Synthetic Metals, 259, 116249. https://doi.org/10.1016/j.synthmet.2019.116249
- Joy, J., George, E., Thomas, S., & Anas, S. (2020). Effect of filler loading on polymer chain confinement and thermomechanical properties of epoxy/boron nitride (h-BN) nanocomposites. New Journal of Chemistry, 44(11), 4494–4503. https://doi.org/10.1039/C9NJ05834F
- Kavimani, V., Gopal, P. M., Stalin, B., Karthick, A., Arivukkarasan, S., Bharani, M., & Tanna, A. R. (2021). Effect of graphene oxide-boron nitride-based dual fillers on mechanical behavior of epoxy/glass fiber composites. Journal of Nanomaterials, 2021, 1–10. https://doi.org/10.1155/2021/5047641
- Khan, M. B., Jan, R., Habib, A., & Khan, A. N. (2017). Evaluating mechanical properties of few layers MoS2 nanosheets-polymer composites. Advances in Materials Science and Engineering, (2017, 1–6. https://doi.org/10.1155/2017/3176808
- Khan, A., Puttegowda, M., Jagadeesh, P., Marwani, H. M., Asiri, A. M., Manikandan, A., Parwaz Khan, A. A., Ashraf, G. M., Rangappa, S. M., & Siengchin, S. (2022). Review on nitride compounds and its polymer composites: A multifunctional material. Journal of Materials Research and Technology, 18, 2175–2193. https://doi.org/10.1016/j.jmrt.2022.03.032
- Kumar, K., Ghosh, P. K., Kumar, A., & Singh, O. (2021). Enhanced thermomechanical properties of ZrO2 particle reinforced epoxy nanocomposite. Journal of Materials Engineering and Performance, 30(1), 145–153. https://doi.org/10.1007/s11665-020-05350-3
- Kumar, R., Kar, K., & Dasgupta, K. (2018). Static and dynamic mechanical analysis of graphite flake filled phenolic-carbon fabric composites and their correlation with interfacial interaction parameters. Polymer Engineering & Science, 58(11), 1987–1998. https://doi.org/10.1002/pen.24809
- Lancaster, J. K. (1968). Relationships between the wear of polymers and their mechanical properties. Proceedings of the Institution of Mechanical Engineers, Conference Proceedings, 183(16), 98–106. https://doi.org/10.1243/PIME_CONF_1968_183_283_02
- Li, L., Chen, Y., & Stachurski, Z. H. (2013). Boron nitride nanotube reinforced polyurethane composites. Progress in Natural Science: Materials International, 23(2), 170–173. https://doi.org/10.1016/j.pnsc.2013.03.004
- Liu, L., Xiao, L., Li, M., Zhang, X., Chang, Y., Shang, L., & Ao, Y. (2016). Effect of hexagonal boron nitride on high-performance polyether ether ketone composites. Colloid and Polymer Science, 294(1), 127–133. https://doi.org/10.1007/s00396-015-3733-2
- Mallick, P. K. (2007). Fiber-reinforced composites: Materials, manufacturing, and design (3rd) ed.). CRC Press. https://doi.org/10.1201/9781420005981
- Ma, J., Luo, N., Xie, Z., Chen, F., & Fu, Q. (2019). Preparation of modified hexagonal boron nitride by ball-milling and enhanced thermal conductivity of epoxy resin. Materials Research Express, 6(10), 1050d8. https://doi.org/10.1088/2053-1591/ab432a
- Mehdikhani, M., Gorbatikh, L., Verpoest, I., & Lomov, S. V. (2019). Voids in fiber-reinforced polymer composites: A review on their formation, characteristics, and effects on mechanical performance. Journal of Composite Materials, 53(12), 1579–1669. https://doi.org/10.1177/0021998318772152
- Michalcová, L., & Kadlec, M. (2016). Carbon/epoxy composite delamination analysis by acoustic emission method under various environmental conditions. Engineering Failure Analysis, 69, 88–96. https://doi.org/10.1016/j.engfailanal.2016.01.008
- Mukhopadhyay, T., Mahata, A., Adhikari, S., & Zaeem, M. A. (2017). Effective mechanical properties of multilayer nano-heterostructures. Scientific Reports, 7(1), 15818. https://doi.org/10.1038/s41598-017-15664-3
- Nayak, S. Y., Shenoy, S., Hameed Sultan, M. T., Kini, C. R., Seth, A., Prabhu, S., & Safri, S. N. A. (2021). Effect of CNT-based resin modification on the mechanical properties of polymer composites. Frontiers in Materials, 7. https://doi.org/10.3389/fmats.2020.609010
- Nayak, S. Y., Shenoy, S., Kini, C. R., Sultan, M. T. H., & Shahar, F. S. (2022). Experimental investigation into mechanical properties of coconut shell powder modified epoxy/3d e-glass composites. Engineered Science, 20. https://doi.org/10.30919/es8e759
- Newcomb, B. A. (2017). Carbon fibers. In Kirk-Othmer encyclopedia of chemical technology(pp. 1–21) John Wiley & Sons, Inc. https://doi.org/10.1002/0471238961.0301180222051411.a01.pub3
- Praveenkumara, J., Madhu, P., Yashas Gowda, T. G., Sanjay, M. R., & Siengchin, S. (2021). A comprehensive review on the effect of synthetic filler materials on fiber-reinforced hybrid polymer composites. The Journal of the Textile Institute, 1–9. https://doi.org/10.1080/00405000.2021.1920151
- Ramdani, N. (2019). Polymer and ceramic composite materials (1st) ed.). CRC Press. https://doi.org/10.1201/b22371
- Rao, Y. S., Mohan, N. S., Shetty, N., & Shivamurthy, B. (2019). Drilling and structural property study of multi-layered fiber and fabric reinforced polymer composite - A Review. Materials and Manufacturing Processes, 34(14), 1549–1579. https://doi.org/10.1080/10426914.2019.1686522
- Rao, Y. S., Mohan, N. S., Shetty, N., & Shivamurthy, B. (2021). Effects of solid lubricant fillers on the flexural and shear strength response of carbon fabric-epoxy composites. Polymer Testing, 96, 107085. https://doi.org/10.1016/j.polymertesting.2021.107085
- Rasul, M. G., Kiziltas, A., Arfaei, B., & Shahbazian-Yassar, R. (2021). 2D boron nitride nanosheets for polymer composite materials. Npj 2D Materials and Applications, 5(1), 56. https://doi.org/10.1038/s41699-021-00231-2
- Ribeiro, H., Trigueiro, J. P. C., Woellner, C. F., Pedrotti, J. J., Miquita, D. R., Silva, W. M., Lopes, M. C., Fechine, G. J. M., Luciano, M. A., Silva, G. G., & Ajayan, P. M. (2020). Higher thermal conductivity and mechanical enhancements in hybrid 2D polymer nanocomposites. Polymer Testing, 87, 106510. https://doi.org/10.1016/j.polymertesting.2020.106510
- Scharf, T. W., & Prasad, S. V. (2013). Solid lubricants: A review. Journal of Materials Science, 48(2), 511–531. https://doi.org/10.1007/s10853-012-7038-2
- Sheikh-Ahmad, J., & Davim, J. P. (2012). Tool wear in machining processes for composites. H. Hocheng(Ed.), Machining technology for composite materials. (Vol. Vol. 1, pp. 116–153). Elsevier. https://doi.org/10.1533/9780857095145.1.116
- Shivamurthy, B., Bhat, K. U., & Anandhan, S. (2013). Mechanical and sliding wear properties of multi-layered laminates from glass fabric/graphite/epoxy composites. Materials & Design, 44, 136–143. https://doi.org/10.1016/j.matdes.2012.07.059
- Song, J. H. (2016). Tensile and bending behavior for woven carbon-aramid/epoxy hybrid composites with various lamination structures by the VARTM method. Fibers and Polymers, 17(8), 1269–1276. https://doi.org/10.1007/s12221-016-6240-2
- Song, J., Dai, Z., Li, J., Zhao, H., & Wang, L. (2019). Silane coupling agent modified BN–OH as reinforcing filler for epoxy nanocomposite. High Performance Polymers, 31(1), 116–123. https://doi.org/10.1177/0954008317748716
- Souza, R. R. R., Nascimento Junior, S. L., Silveira, N. N. A., Arbelo, M. A., & Donadon, M. V. (2019). Translaminar fracture toughness and fatigue crack growth characterization of carbon‐epoxy plain weave laminates. Polymer Composites, 40(10), 3791–3804. https://doi.org/10.1002/pc.25247
- Srinath, G., & Gnanamoorthy, R. (2006). Two-body abrasive wear characteristics of Nylon clay nanocomposites—effect of grit size, load, and sliding velocity. Materials Science and Engineering: A, 435–436, 181–186. https://doi.org/10.1016/j.msea.2006.07.117
- Sudarshan, R. K. (2021). Dynamic mechanical behavior of unfilled and graphite filled carbon epoxy composites. IOP Conference Series: Materials Science and Engineering, 1126(1), 012033. https://doi.org/10.1088/1757-899X/1126/1/012033
- Sudheer, M., Madhyastha, N. K., Amanna, M. K., Jonthan, B., & Jayaprakash, K. M. (2013). Mechanical and abrasive wear behavior of metal sulphide lubricant filled epoxy composites. ISRN Polymer Science, (2013, 1–8. https://doi.org/10.1155/2013/242450
- Sue, H. J., Puckett, P. M., Bertram, J. L., & Walker, L. L.(2000). The network structure of epoxy systems and its relationship to toughness and toughenability. In R. A. Pearson, H. J. Sue, & A. F. Yee(Eds.), Toughening of plastics (1st) (pp. 171–197). American Chemical Society. https://doi.org/10.1021/bk-2000-0759.ch011
- Suresha, B., Chandramohan, G., Renukappa, N. M., & Siddaramaiah. (2007). Mechanical and tribological properties of glass–epoxy composites with and without graphite particulate filler. Journal of Applied Polymer Science, 103(4), 2472–2480. https://doi.org/10.1002/app.25413
- Tawfik, B. E., Leheta, H., Elhewy, A., & Elsayed, T. (2017). Weight reduction and strengthening of marine hatch covers by using composite materials. International Journal of Naval Architecture and Ocean Engineering, 9(2), 185–198. https://doi.org/10.1016/j.ijnaoe.2016.09.005
- Tsuji, Y., Kitamura, Y., Someya, M., Takano, T., Yaginuma, M., Nakanishi, K., & Yoshizawa, K. (2019). Adhesion of epoxy resin with hexagonal boron nitride and graphite. ACS Omega, 4(3), 4491–4504. https://doi.org/10.1021/acsomega.9b00129
- Ulus, H., Şahin, Ö. S., & Avcı, A. (2015). Enhancement of flexural and shear properties of carbon fiber/epoxy hybrid nanocomposites by boron nitride nano particles and carbon nano tube modification. Fibers and Polymers, 16, 12. https://doi.org/10.1007/s12221-015-5603-4
- Ulus, H., Üstün, T., Eskizeybek, V., Şahin, Ö. S., Avcı, A., & Ekrem, M. (2014). Boron nitride-MWCNT/epoxy hybrid nanocomposites: Preparation and mechanical properties. Applied Surface Science, 318, 37–42. https://doi.org/10.1016/j.apsusc.2013.12.070
- Wetzel, B., Rosso, P., Haupert, F., & Friedrich, K. (2006). Epoxy nanocomposites – Fracture and toughening mechanisms. Engineering Fracture Mechanics, 73(16), 2375–2398. https://doi.org/10.1016/j.engfracmech.2006.05.018
- Wypych, G. (2016). The effect of fillers on the mechanical properties of filled materials. In Handbook of fillers (4th). pp. 467–531). ChemTec Publishing. https://doi.org/10.1016/B978-1-895198-91-1.50010-5
- Yu, C., Zhang, J., Li, Z., Tian, W., Wang, L., Luo, J., Li, Q., Fan, X., & Yao, Y. (2017). Enhanced through-plane thermal conductivity of boron nitride/epoxy composites. Composites Part A: Applied Science and Manufacturing, 98, 25–31. https://doi.org/10.1016/j.compositesa.2017.03.012
- Zhang, A., Lu, H., & Zhang, D. (2016). Research on the mechanical properties prediction of carbon/epoxy composite laminates with different void contents. Polymer Composites, 37(1), 14–20. https://doi.org/10.1002/pc.23149
- Zhang, G., Rasheva, Z., Karger-Kocsis, J., & Burkhart, T. (2011). Synergetic role of nanoparticles and micro-scale short carbon fibers on the mechanical profiles of epoxy resin. Express Polymer Letters, 5(10), 859–872. https://doi.org/10.3144/expresspolymlett.2011.85
- Zhou, K., Liu, J., Wen, P., Hu, Y., & Gui, Z. (2014). A noncovalent functionalization approach to improve the dispersibility and properties of polymer/MoS2 composites. Applied Surface Science, 316, 237–244. https://doi.org/10.1016/j.apsusc.2014.07.136