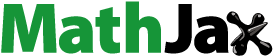
Abstract
Various metal layers grown by e-beam evaporator have been studied to be used as metal contacts for scalable perovskite photovoltaic (PV) devices. The evaporated films consisting of gold (Au), silver (Ag), nickel (Ni), titanium (Ti), tin (Sn), copper (Cu), and molybdenum (Mo) were grown on glass substrates at room temperature with an optimized thickness. Later, the measured optical properties such as transmission and absorptance of such films were used computationally to extract the optimum device performance using SCAPS-1D software. Among all the layers, Ti-based perovskite solar cells outperform other metal contacts with a power conversion efficiency of (>27%). The films were characterized optically, topologically, structurally, and morphologically using ultraviolet—visible (UV—Vis) spectrometry, atomic force microscopy, x-ray photoelectron spectroscopy (XPS), three-dimensional (3D) profilometry, and scanning electron microscopy. The morphological data confirm the growth of compact, uniform, and defect-free metal films as confirmed by the field emission scanning electron microscopy. Contact angle measurement was also performed to determine the wettability of metal surfaces. Both Au and Ni films were found semi-hydrophilic which shows the adaptability of better stability through repelling water from the surface. The computational analysis confirms that screening of suitable metal back contact is necessary to increase device performance and stability significantly.
1. Introduction
Hybrid perovskite photovoltaic devices (HPPDs) have gained significant attention in the photovoltaic (PV) research and development sector due to their promising photoconversion efficiency and low-cost fabrication process (Grätzel, Citation2014; Kumar et al., Citation2018; Quasim Khan & Ahmad, Citation2020; Shao et al., Citation2018; Zhou et al., Citation2018). Although the initial device performance was as low as 3.9% in 2009, the small-scale device performance jumped to 25% by 2020 (Chung et al., Citation2012; Konstantakou & Stergiopoulos, Citation2017), making such hybrid materials (such as methylammonium lead halide, CH3NH3PbI3) highly competitive with other conventional PV device materials (Kim et al., Citation2020; Salem et al., Citation2021). The suitability of such hybrid organic-inorganic materials to develop high efficiency in small- to large-scale HPPDs lies in their properties such as high absorption coefficient, larger carrier diffusion length, lower binding energy, high electron-hole mobility, lesser defects, and bi-functional materials with excitation and mobility (E. Wang et al., Citation2018; Ghosh et al., Citation2021; Lin et al., Citation2019; Mahapatra et al., Citation2022; Nizamuddin et al., Citation2021). However, before full-scale deployment in PV modules in a power plant, certain issues need to be addressed, such as stability, finding suitable and cost-effective metal contacts, and replacing toxic lead with another suitable material (Michaelson,Citation1977; Macis et al., Citation2019; Shiraishi & Ata, Citation2001; Song et al., Citation2012). In general, an HPSC device consists of four main layers: electron transport material, absorber layer, hole transport layer, and metal contact. As shown in Figure , the metal contact layer plays a significant role in determining band alignment and should act as an electron blocking layer diffused from the hole transport material to complete the electrical circuit (Hossain & Aïssa, Citation2017; J. Wu et al., Citation2022; Nath, Ramamurthy, Hegde, et al., Citation2022; Samantaray et al., Citation2022; Y. Wu et al., Citation2022). Until now, gold (Au) has been the most commonly used contact material due to its best-matched work function with hole transport materials (Afzaal & Karkain, Citation2022; Jebakumar et al., Citation2022; Khattak et al., Citation2022; Nath, Ramamurthy, Mahapatra, et al., Citation2022; Saeed & Gelani, Citation2022). Alternative metal contacts such as copper (Cu), aluminum (Al), and silver (Ag) have also been used (M. Wang et al., Citation2022; Raza et al., Citation2022). However, most of them have degrading factors, including oxidization effects, less adhesion, non-feasible large-scale fabrication, very high series resistance, and high fabrication cost (Aitola et al., Citation2022; Jeyakumar & Bag, Citation2022; Sun et al., Citation2022). Despite the undeniable performance of the device, some issues still require attention for full-phase deployment in the form of PV modules in a power plant.
Generally, the device performance and stability significantly depend on the band alignment of the metal contacts (Bal et al., Citation2022). It is significantly required to develop pristine metal contacts in a precise environment to minimize interface defects states with a very carrier mobility to achieve the highest power conversion efficiency. In one previously published article, it has been found that device performance depends proportionally on the increasing metal function value as carrier collection improves significantly (Hossain & Aïssa, Citation2017). The achieved results suggest that it is not trivial to tailor the work function accordingly for the optimum charge extraction. In this work, we have grown different metal layers such as gold (Au), silver (Ag), nickel (Ni), tin (Sn), copper (Cu), and molybdenum (Mo) using e-beam evaporation on glass substrates by varying the thicknesses. Later, the samples were characterized optically, electrically, and morphologically. The optical properties were feed in SCAPS-1D software to extract the device performance accordingly. This will help in screening out the suitable metal contact to develop highly efficient HPPDs. In addition, thickness should be tuned accordingly to make them suitable for developing bi-facial perovskite solar cells where incident light can be exposed through the metal contact, which in general provides a gain of 25% of the overall performance. Performing numerical analysis is crucial in order to assess device performance and identify the most suitable material for full-scale deployment. Therefore, in this study, we utilized experimental results to evaluate key performance indicators such as open-circuit voltage (VOC), short-circuit current (JSC), fill factor (FF), and efficiency. Our analysis focused on the optical properties of the metal layers that were developed. Specifically, we investigated the impact of metal contacts on the performance of perovskite solar cells based on CH3NH3PbI3. Our investigation took into account the electrode work function and optical properties for charge collection, and we reported our findings in detail.2. Methodology
2.1. Materials preparation
Metal thin films of gold (Au), silver (Ag), nickel (Ni), tin (Sn), copper (Cu), titanium (Ti), and molybdenum (Mo) were grown on soda lime glass samples (1” × 3”) using e-beam evaporation at room temperature at a very controlled deposition rate of 1 Å/s without any oxygen flow. The deposition rate was controlled through automation of the software where tooling factor, material density, and Z-ratio were inserted to adjust the final thickness accordingly. The final thickness for all films were 50 nm. Samples were optically measured using ultraviolet—visible (UV—Vis) spectroscopy. At the beginning, thickness optimization was carried for each layer to maximize the optical properties. Our tool allows us to deposit multi-layers in a stacked manner without breaking the vacuum. The substrates were sonicated and cleaned using different solvents such as DI water, acetone, and isopropanol. Later, they were dried under inert nitrogen. The e-beam evaporation tool was a Denton Vacuum ExplorerTM evaporator where evaporation pellets were purchased from Kurt J. Lesker (purity: 99.9995%).
3. Characterization
UV—Vis spectroscopy (PerkinElmer Lambda 1050 UV/VIS/NIRTM) and contact angle (KrussTM) techniques were performed to characterize the optical as well as wettability (hydrophilicity/hydrophobicity) properties of the films. X-ray photoelectron spectroscopy (XPS) (Escalab 250×iTM) with a monochromatic Al Kα source was used to find the stoichiometry of the thin films, and later, Avantage software was used to analyze spectral analysis and peak fitting. Films morphology was scanned using a JEOL 7610TM microscopy. DektakTM stylus profilometer was used to study the 3D average roughness of the as grown films. BrukerTM AFM was used to find out the topology of the metal films and KRUSSTM system helped in measuring the wettability of the metal films.
4. Numerical simulation
Using the SCAPS-1D software, which was developed at the University of Gent, Belgium (Bal et al., Citation2022; Belarbi et al., Citation2022; Chawki et al., Citation2022; Hossain et al., Citation2015; Moulaoui et al., Citation2022; Qasim et al., Citation2022), we conducted numerical simulations of a PSC solar cell. This software employs a finite difference approach to solve the electron and hole drift-diffusion equations in position space to represent charge movement within the device, based on given input parameters (electrical and optical) for each layer of the device. At each mesh point in position space, a set of equations for carrier trapping and escape are solved in energy space. The potential profile across the device, which is space charge dependent, is modeled using Poisson’s equation, while the carrier continuity equations are used to calculate charge transport in the device. The TiO2/perovskite absorber layer/Spiro-OMETAD/metal contacts structure was employed for the numerical analysis, utilizing the data presented in Table .
Table 1. List of parameters used in this numerical simulation are provided (Hossain et al., Citation2015)
5. Results and discussions
Chemical properties of gold (Au) and copper (Cu) thin films
Figure captures the X-ray photoelectron spectroscopy (XPS) analysis as fitted by Avantage software. The source used to excite electron is Al K alpha with an energy of 1486.68 eV, pass energy of 20 eV for all narrow scans and 100 eV for survey scans. During the high-resolution scanning, each spectrum considers 10 scans with 1-survey spectra. As studied, the survey spectrum confirms the growth of pristine gold (Au) and copper (Cu) films with a minimal carbon contamination. In general, deposition pressure plays a significant role to turn surface into oxidized phase. Al evaporated samples of Au and Cu confirm the stoichiometric growth without any foreign contamination. In our study, we have considered only these two metal films to investigate the deposition conformity.
5.2. Optical properties of Nickel oxide (NiO) thin films
The optical properties of the films were studied using UV-Vis spectroscopy (Perkin ElmerTM) for the wavelength range of 200 to 2000 nm as shown in . Absorptance spectra was calculated using:
Figure 3. UV-Vis results of various metal thin films with 50 nm of thickness (a) transmittance (%), (b) reflectance (%), and (c) absorptance (%).

Where A is the absorptance, T is the transmittance, and R is the reflectance.
Transmission, reflectance, and absorptance spectra confirm a clear difference among all films grown with 50 nm of thicknesses for the wavelength range of 200 to 2000 nm. Hence, metallic properties become explicit parameters for further understanding. The reflection characteristics of metallic films can vary greatly, and for our particular application, it is important that the percentage of reflected light (R%) is minimized in the visible region and maximized in the infrared region. Additionally, we require the percentage of transmitted light (T%) to be high for effective light management, particularly for bi-facial perovskite solar cells. Our experiments show that the 300 nm films exhibit the lowest R% and the highest percentage of absorption (A%), which is consistent with other reports that have shown a correlation between lower reflectance and thicker films (Choi et al., Citation2022). The transmission, reflectance, and absorptance spectra indicate clear differences among all the metal films for the wavelength range of 200 to 2000 nm. The Ti films exhibit the lowest R% among all samples except for the remaining materials. Subsequently, we utilized the spectrum data to perform numerical simulations using SCAPS software.
5.3. Surface roughness analysis
The average roughness measurements obtained using the 3D mode are presented in Figure . These measurements confirmed that the average roughness varies depending on the metal layers used. Specifically, the Ni layers exhibited the highest average roughness of 51.04 nm, while the lowest average roughness of 19.33 nm was observed with Ag. These results are consistent with the contact results, which suggest that smaller grains can increase super-hydrophobicity due to the increased tension between water molecules and thin films. It is important to note that the metal layers play a crucial role in regulating the roughness of the films.
5.4. Contact angle properties
In general, super-hydrophilic (0°–30°) or super-hydrophobic (>100°) of thin films can be used for anti-soiling applications (Blossey, Citation2003; Cheng & Rodak, Citation2005; Feng & Jiang, Citation2006; Fürstner et al., Citation2005; Lafuma & Quéré, Citation2003; Miwa et al., Citation2000). Super-hydrophobic surface is mainly consisted of high surface energy materials to repel dust accumulation. Such surface phenomenon is the best tangible product to develop anti-soiling coatings, for example in the space industry. In our study, it is inevitably important to tune the surface properties for a hydrophobic surface. As shown in Figure , Ni films show the highest hydrophobicity with a CA of about 101°, whereas hydrophilic behavior has been measured for the some other films. Previous study confirms that surface wettability changes significantly with roughness (Hossain et al., Citation2021). Super-hydrophilic Ti films with an angle of 0° is mainly due to less surface roughness. Super-hydrophobic surface can be engineered through surface tuning to be rougher.
5.5. Morphological analysis
Figure shows the general surface images of the evaporated Ti, Cu, and Ni with 50 nm of thickness, where Figure capture the cross-sectional image of Ti films. As confirmed by the measurement, all evaporated layers are dense, homogenous, and without any pinholes. As confirmed, evaporated films are with entire coverage and uniformity, which are essential to develop high-end optoelectronic devices such as infrared filters, metallic contacts. For our application, it is significantly important to develop films with pristine quality for an optimized light management as cracked surfaces with defects can suppress film properties of reflecting infrared solar spectrum and also through acting as recombination centers. As imaged, all films show smaller grains and confirm uniform films throughout the entire surface.
5.6. Topological analysis
AFM analysis results (Figure ) confirm that surface roughness is around 1.56 nm for Au sample and 1.71 nm for Ti films. Additionally, the results can be correlated with SEM and profilometer images. Such results can be correlated with formation of islands, improved surface diffusion of atoms, and rearrangements of the crystallites.
5.7. Numerical analysis
The numerical analysis was conducted on a device structure consisting of FTO/TiO2/perovskite absorber layer/Spiro-OMETAD/metal contacts, using SCAPS software. The analysis utilized the optical properties of the metal films, which were determined from experimental optical data. Figure displays the results obtained by varying the thickness of the perovskite absorber layer from 200 nm to 650 nm. The calculations indicate that using a Ti metal contact layer in perovskite devices results in higher Jsc (46.89 mA/cm2) and Voc (0.770 V). This improvement in performance is mainly attributed to the improved optical properties, which leads to an increase in Jsc due to the absence of defects during growth. Voc is a function of Jsc, and thus an increase is expected. However, it was observed that both Voc and Jsc decrease significantly when the perovskite thickness exceeds 400 nm, resulting in a drop in the photoconversion efficiency from 28.1% to 24.8% as the thickness increased from 450 to 650 nm, respectively. It is evident that when the recombination constant has a small value, both Jsc and Voc, and therefore PCE, reach saturation beyond an absorbing layer thickness of 400 nm. However, for higher values of the rate, they decrease. Additionally, it is noteworthy that the decrease in Voc is more substantial than that of the short-circuit current for thick perovskite layers. As shown, fill factor remains constant throughout all simulation results. Better optical properties of the metal layer tend to yield the higher PV performance.
6. Conclusions
We developed and determined the impact of different metal contacts on cell performance by employing numerical simulations of full cells. In our study, gold (Au), silver (Ag), nickel (Ni), titanium (Ti), tin (Sn), copper (Cu), and molybdenum (Mo) confirm pristine growth with an optimized thickness as investigated by different characterization tools. Absorber layer thickness as well as the optical properties were varied accordingly. Among all the structures, Ti-based devices outperform other metal contacts with a power conversion efficiency of (>27%), which is hypothetically due to the lowest reflectance. Also, it has been noticed that a clear drop occurs for perovskite thickness above 400 nm, resulting in turns in a clear drop of the photo conversion efficiency, from 28.1% to 24.80% when thickness increased from 450 to 650 nm, respectively. Our calculations suggest that the device performance is somewhat less sensitive to the thickness up to 400 nm. Furthermore, for the first time, we demonstrate the impact of different metal layers on the performance of PSCs. Based on results from this study, we have demonstrated a correlation between device performance and various metal contacts, where the optoelectrical properties were kept fixed. This is a significant advancement from prior full-cell simulation studies that have used estimated parameters. Finally, our results provide an opportunity to improve the performance by tuning the processing parameters to favor semi-deep level defects with lower formation energies rather than deep level defects. Therefore, such structures with metal layers will result in scalability and stability.
Disclosure statement
No potential conflict of interest was reported by the authors.
Additional information
Funding
References
- Afzaal, M., & Karkain, S. (2022). Environmental Assessment of Perovskite Solar Cells. In The effects of dust and heat on photovoltaic modules: Impacts and solutions (pp. 279–11). Elsevier.
- Aitola, K., Sonai, G. G., Markkanen, M., Kaschuk, J. J., Hou, X., Miettunen, K., & Lund, P. D. (2022). Encapsulation of commercial and emerging solar cells with focus on perovskite solar cells. Solar Energy, 237, 264–283. https://doi.org/10.1016/j.solener.2022.03.060
- Bal, S. S., Basak, A., & Singh, U. P. (2022). Numerical modeling and performance analysis of Sb-based tandem solar cell structure using SCAPS–1D. Optical Materials, 127, 112282. https://doi.org/10.1016/j.optmat.2022.112282
- Belarbi, M., Zeggai, O., & Louhibi-Fasla, S. (2022). Numerical study of methylammonium lead iodide perovskite solar cells using SCAPS-1D simulation program. Materials Today: Proceedings. Algeria.
- Blossey, R. (2003). Self-cleaning surfaces—virtual realities. Nature Materials, 2(5), 301–306. https://doi.org/10.1038/nmat856
- Chawki, N., Rouchdi, M., & Fares, B. (2022). Numerical study of BaZrS3 based Chalcogenide Perovskite solar cell using SCAPS-1D device simulation. Optical Materials, 126, 112250 .
- Cheng, Y.T., & Rodak, D. E. (2005). Is the lotus leaf superhydrophobic? Applied Physics Letters, 86(14), 144101. https://doi.org/10.1063/1.1895487
- Choi, H. K., Bae, S., Lee, S. K., Lee, S. H., Lee, K., Ko, S. Y., Kang, J. W., Yang, S. Y., & Kim, T. W. (2022). Tailoring the internal structure of porous copper film via size-controlled copper nanosheets for electromagnetic interference shielding. Materials Science and Engineering: B, 278, 115611. https://doi.org/10.1016/j.mseb.2022.115611
- Chung, I., Lee, B., He, J., Chang, R. P. H., & Kanatzidis, M. G. (2012). All-solid-state dye-sensitized solar cells with high efficiency. Nature, 485(7399), 486–489. https://doi.org/10.1038/nature11067
- Feng, X. J., & Jiang, L. (2006). Design and creation of superwetting/antiwetting surfaces. Advanced Materials, 18(23), 3063–3078. https://doi.org/10.1002/adma.200501961
- Fürstner, R., Barthlott, W., Neinhuis, C., & Walzel, P. (2005). Wetting and self-cleaning properties of artificial superhydrophobic surfaces. Langmuir, 21(3), 956–961. https://doi.org/10.1021/la0401011
- Ghosh, P., Sundaram, S., Nixon, T. P., & Krishnamurthy, S. (2021). Influence of nanostructures in Perovskite solar cells reference module in materials science and materials engineering. Elsevier.
- Grätzel, M. (2014). The light and shade of perovskite solar cells. Nature Materials, 13(9), 838–842. https://doi.org/10.1038/nmat4065
- Hossain, M. I., & Aïssa, B. (2017). Effect of structure, temperature, and metal work function on performance of organometallic perovskite solar cells. Journal of Electronic Materials, 46(3), 1806–1810. https://doi.org/10.1007/s11664-016-5232-8
- Hossain, M. I., Aïssa, B., Samara, A., Mansour, S. A., Broussillou, C. A., & Bermudez Benito, V. (2021). Hydrophilic antireflection and antidust silica coatings. ACS Omega, 6(8), 5276–5286. https://doi.org/10.1021/acsomega.0c05405
- Hossain, M. I., Alharbi, F. H., & Tabet, N. (2015). Copper oxide as inorganic hole transport material for lead halide perovskite based solar cells. Solar Energy, 120, 370–380. https://doi.org/10.1016/j.solener.2015.07.040
- Jebakumar, J., Moni, D. J., Gracia, D., & Shallet, M. D. (2022). Design and simulation of inorganic perovskite solar cell. Applied Nanoscience, 12(5), 1–12. https://doi.org/10.1007/s13204-021-02268-7
- Jeyakumar, R., & Bag, A. (2022). Performance evaluation and optimization of CH3NH3PbBr3 based planar perovskite solar cells using various hole-transport layers. Solar Energy, 236, 832–840. https://doi.org/10.1016/j.solener.2022.03.048
- Khattak, Y. H., Vega, E., Baig, F., & Soucase, B. M. (2022). Performance investigation of experimentally fabricated lead iodide perovskite solar cell via numerical analysis. Materials Research Bulletin, 151, 111802. https://doi.org/10.1016/j.materresbull.2022.111802
- Kim, J., Kim, K. S., & Myung, C. W. (2020). Efficient electron extraction of SnO2 electron transport layer for lead halide perovskite solar cell. Npj Computational Materials, 6(1), 61 6 1–8. https://doi.org/10.1038/s41524-020-00370-y
- Konstantakou, M., & Stergiopoulos, T. (2017). A critical review on tin halide perovskite solar cells. Journal of Materials Chemistry, 5(23), 11518–11549. https://doi.org/10.1039/C7TA00929A
- Kumar, P. M., Das, A., Seban, L., & Nair, R. G. (2018). Chapter 8. Fabrication and life time of Perovskite solar cells.
- Lafuma, A., & Quéré, D. (2003). Superhydrophobic states. Nature Materials, 2(7), 457–460. https://doi.org/10.1038/nmat924
- Lin, L., Jiang, L., Li, P., Fan, B., & Qiu, Y. (2019). A modeled perovskite solar cell structure with a Cu2O hole-transporting layer enabling over 20% efficiency by low-cost low-temperature processing. The Journal of Physics and Chemistry of Solids, 124, 205–211. https://doi.org/10.1016/j.jpcs.2018.09.024
- Macis, S., Aramo, C., Bonavolontà, C., Cibin, G., D’elia, A., Davoli, I., Lucia, M. D., Lucci, M., Lupi, S., Miliucci, M., Notargiacomo, A., Ottaviani, C., Quaresima, C., Scarselli, M., Scifo, J., Valentino, M., Padova, P. D., & Marcelli, A. (2019). MoO3 films grown on polycrystalline Cu: Morphological, structural, and electronic properties. Journal of Vacuum Science & Technology, 37(2), 021513. https://doi.org/10.1116/1.5078794
- Mahapatra, B., Krishna, R. V., Laxmi, & Patel, P. K. (2022). Design and optimization of CuSCN/CH3NH3PbI3/TiO2 perovskite solar cell for efficient performance. Optics Communications, 504, 127496. https://doi.org/10.1016/j.optcom.2021.127496
- Mechtly E A 2002 Properties of materials reference data for engineers. Newnes.
- Michaelson, H. B (1977). The work function of the elements and its periodicity related articles the work function of the elements and its periodicity addit. Journal of Applied Physics, 48(11), 191911. https://doi.org/10.1063/1.323539
- Miwa, M., Nakajima, A., Fujishima, A., Hashimoto, K., & Watanabe, T. (2000). Effects of the surface roughness on sliding angles of water droplets on superhydrophobic surfaces. Langmuir, 16(13), 5754–5760. https://doi.org/10.1021/la991660o
- Moulaoui, L., Bajjou, O., Najim, A., Archi, M. and Rahmani, K., 2022, March. Numerical simulation of the NiO as hole transport layer in CH 3 NH 3 PbBr 3 perovskite based-solar cell using SCAPS-1D. In 2022 2nd International Conference on Innovative Research in Applied Science, Engineering and Technology (IRASET), Morocco, (pp. 1–7). IEEE.
- Nath, B., Ramamurthy, P. C., Hegde, G., & Roy Mahapatra, D. (2022). Role of electrodes on perovskite solar cells performance: A review. ISSS Journal of Micro and Smart Systems, 11(1), 1–19. https://doi.org/10.1007/s41683-021-00089-y
- Nath, B., Ramamurthy, P. C., Mahapatra, D. R., & Hegde, G. (2022). Electrode transport layer–metal electrode interface morphology tailoring for enhancing the performance of Perovskite solar cells. ACS Applied Electronic Materials, 4(2), 689–697. https://doi.org/10.1021/acsaelm.1c01100
- Nizamuddin, A., Arith, F., Rong, I. J., Zaimi, M., Rahimi, A. S., & Saat, S. (2021). Investigation of Copper(I)Thiocyanate (CuSCN) as a hole transporting layer for perovskite solar cells application. Journal of Advanced Research in Fluid Mechanics and Thermal Sciences, 78(2), 153–159. https://doi.org/10.37934/arfmts.78.2.153159
- Qasim, I., Ahmad, O., Ul Abdin, Z., Rashid, A., Nasir, M. F., Malik, M. I., Rashid, M., & Hasnain, S. M. (2022). Design and numerical investigations of eco-friendly, non-toxic (Au/CuSCN/CH3NH3SnI3/CdTe/ZnO/ITO) perovskite solar cell and module. Solar Energy, 237, 52–61. https://doi.org/10.1016/j.solener.2022.02.056
- Quasim Khan, M., & Ahmad, K. (2020). Origin and fundamentals of Perovskite solar cells. IntechOpen.
- Raza, E., Ahmad, Z., Asif, M., Aziz, F., Riaz, K., Mehmood, M. Q., Bhadra, J., & Al-Thani, N. J. (2022). Numerical modeling and performance optimization of carbon-based hole transport layer free perovskite solar cells. Optical Materials, 125, 112075. https://doi.org/10.1016/j.optmat.2022.112075
- Saeed, F., & Gelani, H. E. (2022). Unravelling the effect of defect density, grain boundary and gradient doping in an efficient lead-free formamidinium perovskite solar cell. Optical Materials, 124, 111952. https://doi.org/10.1016/j.optmat.2021.111952
- Salem, M. S., Shaker, A., Zekry, A., Abouelatta, M., Alanazi, A., Alshammari, M. T., & Gontand, C. (2021). Analysis of hybrid hetero-homo junction lead-free perovskite solar cells by scaps simulator. Energies, 14(18), 5741. https://doi.org/10.3390/en14185741
- Samantaray, M. R., Rana, N. K., Kumar, A., Ghosh, D. S., & Chander, N. (2022). Stability study of large‐area perovskite solar cells fabricated with copper as low‐cost metal contact. International Journal of Energy Research, 46(2), 1250–1262. https://doi.org/10.1002/er.7243
- Shao, S., Liu, J., Portale, G., Fang, H. -H., Blake, G. R., Brink, G. H. T., Koster, L. J. A., & Loi, M. A. (2018). Highly reproducible Sn-Based Hybrid Perovskite solar cells with 9% efficiency. Advanced Energy Materials, 8(4), 1702019. https://doi.org/10.1002/aenm.201702019
- Shiraishi, M., & Ata, M. (2001). Work function of carbon nanotubes Carbon. Carbon, 39(12), 1913–1917. https://doi.org/10.1016/S0008-6223(00)00322-5
- Song, S. M., Park, J. K., Sul, O. J., & Cho, B. J. (2012). Determination of work function of graphene under a metal electrode and its role in contact resistance. Nano Letters, 12(8), 3887–3892. https://doi.org/10.1021/nl300266p
- Sun, X., Lin, T., Ding, C., Guo, S., Ismail, I., Wang, Z., Wei, J., Luo, Q., Lin, J., Zhang, D., & Ma, C. Q. (2022). Fabrication of opaque aluminum electrode-based perovskite solar cells enabled by the interface optimization. Organic Electronics, 104, 106475. https://doi.org/10.1016/j.orgel.2022.106475
- Wang, E., Chen, P., Yin, X., Gao, B., & Que, W. (2018). Boosting efficiency of planar heterojunction perovskite solar cells by a low temperature TiCl4 treatment. Journal of Advanced Dielectrics, 8(02), 1850009. https://doi.org/10.1142/S2010135X18500091
- Wang, M., Liu, J., Ma, C., Wang, Y., Li, J., & Bian, J. (2022). Modular perovskite solar cells with Cs0. 05 (FA0. 85MA0. 15) 0.95 Pb (I0. 85br0. 15) 3 light-harvesting layer and graphene electrode. Journal of Electronic Materials, 51(5), 2381–2389. https://doi.org/10.1007/s11664-022-09509-7
- Wu, J., Cha, H., Du, T., Dong, Y., Xu, W., Lin, C. T., & Durrant, J. R. (2022). A comparison of charge carrier dynamics in organic and perovskite solar cells. Advanced Materials, 34(2), 2101833. https://doi.org/10.1002/adma.202101833
- Wu, Y., Sun, X., Dai, S., Li, M., Zheng, L., Wen, Q., Tang, B., Yun, D. Q., & Xiao, L. (2022). Broad-band-enhanced plasmonic perovskite solar cells with irregular silver nanomaterials. ACS Applied Materials & Interfaces, 14(14), 16269–16278. https://doi.org/10.1021/acsami.2c01759
- Zhou, D., Zhou, T., Tian, Y., Zhu, X., & Tu, Y. (2018). perovskite-based solar cells: materials, methods, and future perspectives. Journal of Nanomaterials, 2018, 1–15. https://doi.org/10.1155/2018/8148072